Jessica D. Briley1, Kate M. Bailey2 and Lydia Love1 1 Department of Molecular Biomedical Sciences, College of Veterinary Medicine, North Carolina State University, Raleigh, North Carolina, USA 2 United Veterinary Care, and College of Veterinary Medicine, North Carolina State University (Adjunct), Raleigh, North Carolina, USA Since William Thomas Green Morton’s demonstration of ether use in 1846, the delivery of anesthesia and the monitoring of anesthetized patients have undergone significant transformation [1]. Today, vigilant perianesthetic care necessitates the assessment of cardiovascular, ventilatory, and metabolic status. Among these, monitoring ventilation plays a role in providing crucial patient‐ and equipment‐related information. During anesthesia, the patient’s ability to maintain proper ventilation may be compromised by anesthetic and analgesic drugs, muscle relaxation, and changes in respiratory drive. Monitoring ventilation allows assessment of carbon dioxide production, delivery to the lungs, and elimination via gas exchange, thereby preventing complications and ensuring patient well‐being [2,3]. It is imperative for an anesthetist to understand the physiologic principles of ventilation and various assessment methods to ensure patient safety and optimize anesthesia delivery. Although occasionally used interchangeably, ventilation and respiration can be viewed as two separate physiologic functions. Ventilation refers to the movement of gases, specifically oxygen and carbon dioxide (CO2), in and out of the alveoli in the lungs. Respiration refers to the cellular utilization of oxygen in the process of generating energy in the form of adenosine triphosphate (ATP). In aerobic respiration, glycolysis of carbohydrates occurs in the cytoplasm in the presence of oxygen. Pyruvate moves into mitochondria and enters the tricarboxylic acid (TCA) cycle after conversion to acetyl‐CoA (Fig. 14.1). The final step in aerobic respiration is oxidative phosphorylation, including the electron transport chain and the process of chemiosmosis. This series of reactions generates ATP, which is the primary source of energy for cells. For efficient aerobic respiration to occur, there must be an adequate supply of glucose and oxygen‐rich blood to the mitochondria in the tissues. Carbon dioxide is generated as a by‐product of aerobic respiration. The partial pressure of carbon dioxide (PCO2) is highest within the mitochondria, from which it passes through the cytoplasm to the blood, and then is carried to the lungs for excretion. Carriage of CO2 within the blood occurs primarily as bicarbonate, as a dissociation product from the buffering of CO2 with water to form carbonic acid, and, to a lesser extent, as carbamino compounds bound to hemoglobin and plasma proteins or dissolved gas (see Chapter 37). Once delivered to the lungs, alveolar ventilation allows for gas exchange between the alveoli and circulation resulting in the acquisition of oxygen and expiration of CO2. Alveolar CO2 (PACO2) is directly proportional to the amount of CO2 produced by metabolism (VCO2) and subsequently delivered to the lungs and inversely proportional to alveolar ventilation (VA): The constant 0.863 accounts for dissimilar units. Given the relationship between ventilation and perfusion, monitoring CO2 in the respiratory gases provides the anesthetist with important information about metabolism, cardiac output, and ventilation. Figure 14.1 The tricarboxylic acid (TCA) cycle is the foundation of cellular energy generation. In the cytoplasm, glycolysis of carbohydrates creates pyruvate. Pyruvate enters the TCA cycle in the mitochondrial matrix after conversion to acetyl‐Coenzyme A (acetyl‐CoA) by pyruvate dehydrogenase (PDH). Each oxidative step in the TCA cycle reduces a coenzyme (nicotinamide adenine dinucleotide [NADH] or flavin adenine dinucleotide [FADH2]). These reduced coenzymes participate in the electron transport chain, creating the majority of ATP production. FAD, flavin adenine dinucleotide; FADH2, flavin adenine dinucleotide (reduced); GDP, guanosine diphosphate; GTP, guanosine triphosphate; NAD+, nicotinamide adenine dinucleotide; NADH, nicotinamide adenine dinucleotide (reduced). Source: Borkum [4], with permission. Carbon dioxide and its equilibration between the intracellular and extracellular environments are critical to acid–base homeostasis and can provide valuable information about respiratory status and compensatory mechanisms. The concentration of CO2 in the blood is directly related to the regulation of pH. Increased levels of CO2 result in higher carbonic acid (H2CO3) formation and thus the generation of H+ ions, leading to a decrease in blood pH (acidemia) and a compensatory increase in blood bicarbonate (HCO3‐) concentration. Conversely, decreased CO2 levels reduce carbonic acid formation, resulting in an increase in blood pH (alkalemia) and compensatory decrease in blood HCO3‐. Respiratory alkalemia (hypocapnia) and acidemia (hypercapnia) result in rapid equilibration between the extra‐ and intracellular fluids, causing more dramatic shifts in pH during these conditions when compared to metabolic acidemia or alkalemia [5]. There are many potential physiologic consequences of hypercapnic acidemia including catecholamine release (may lead to tachyarrhythmias), increased cardiac output, electrolyte shifts (e.g., hyperkalemia), rightward shift of the oxygen–hemoglobin dissociation curve, and cerebral vasodilation [6–8]. In the face of severe hypercapnia (> 80 mmHg), central nervous system signs including narcosis and coma can occur [9,10]. When decreases in the arterial partial pressure of CO2 (PaCO2) result in pH greater than 7.6, the physiologic consequences may include cerebral and myocardial vasoconstriction with resulting decreases in blood flow, decreased cardiac output, leftward shift of the oxygen–hemoglobin dissociation curve, hypokalemia, and predisposition to arrhythmias [11]. Perianesthetic mortality trends appear to be decreasing in veterinary patients over time, though remain significantly higher than in humans [12–23]. Given the wide array of physiologic consequences of ventilatory derangements, early detection of respiratory events through advanced monitoring, including capnography, allows for timely intervention and enhanced patient safety throughout the perioperative period [24–27]. The American College of Veterinary Anesthesia and Analgesia (ACVAA) published its stance on anesthetic monitoring of small animal patients in 2009 [28]. Within those recommendations, multiple ventilation monitoring techniques are mentioned, including subjective measurements, such as visualization of chest wall expansion or movement of an attached reservoir bag, and thoracic auscultation via external or esophageal stethoscope, or the use of an audible respiratory monitor. These techniques indicate a binary endpoint (i.e., absence or presence) and do not allow for objective measurements, as they do not provide a carbon dioxide value. Partial pressure of end‐tidal carbon dioxide (PETCO2) with a capnograph or blood gas analysis to obtain PaCO2 or partial pressure of venous carbon dioxide (PvCO2) are the major objective monitoring methods for ventilation. The use of spirometry is mentioned for measurement of tidal volume and investigation of respiratory mechanics, and while this is an objective measurement, it does not provide partial pressure of carbon dioxide. The subjective measurements are considered essential or required by the ACVAA. Capnography is recommended, and blood gas analysis recommended as needed on a patient‐specific basis. The Small Animal Monitoring Guidelines are in the process of being updated as of 2023. The American Society of Anesthesiologists (ASA) has dictated since 1989 that any procedure in which endotracheal intubation or laryngeal mask airway is used requires the use of capnometry, capnography, or mass spectroscopy to quantify expired carbon dioxide. The requirement for quantitative monitoring of carbon dioxide was extended to sedated patients in 2010 [29]. It is recommended that alarms associated with abnormal carbon dioxide are audible in the operating room. A closed claims analysis at the end of the last century determined that of respiratory events, including inadequate ventilation, esophageal intubation, and difficult airway, 72% could have been prevented using better respiratory monitoring, and specifically, that the combination of pulse oximetry and capnography could have prevented > 90% of claims for inadequate ventilation and esophageal intubation [2]. The value of capnography in veterinary anesthesia and critical care settings is such that its use should be encouraged in primary care practice settings and an expectation in a specialty or tertiary care setting. The most accessible methods to monitor ventilation are the subjective assessment of breathing by visualization and auscultation of all lung fields and the trachea with a stethoscope. These methods should be included in every preanesthetic assessment, should accompany other monitoring techniques throughout the anesthetic event, including the recovery period, and should be utilized diagnostically in the event of a respiratory complication. Additionally, auscultation of lung sounds bilaterally directly after intubation is a highly recommended technique to confirm appropriate endotracheal tube (ETT) placement and aid in early diagnosis of endobronchial or esophageal intubation. The lung sounds, as well as respiratory rate, effort, and quality, can indicate various causes for a change in ventilation. For example, a patient with a fixed airway obstruction will tend toward a decreased respiratory rate with a longer inspiratory phase. A patient with an alveolar disease or pleural space disease will often experience an increase in respiratory effort and could have increased (e.g., crackles in pneumonia) or decreased (e.g., absence due to pleural fluid) lung sounds. Respiratory disease may negatively impact ventilation; anesthetic management requirements of specific respiratory conditions vary and are discussed in Chapter 38. Apnea monitors are simple respiratory monitoring tools that have been used historically in veterinary medicine and persist in some practice settings. Apnea monitors attach to the circuit between the distal end of the ETT and the patient end of the breathing circuit. The major information gained from an apnea monitor is the presence of inhalation/exhalation and a respiratory rate. Their design can be as simple as a low‐profile plastic attachment that creates a “whistle” noise during the breathing cycle. They also come in a more sophisticated design with a circuit attachment and separate digital display that alerts the user with an audible and visual respiratory “beep” with each breath, displays the time between breaths, and provides an alarm that is triggered by a preset apnea duration (Fig. 14.2). Most models are equipped with battery power, making them highly portable. These monitors give a binary response (i.e., movement of gas or not) but do not provide any information about the effectiveness of respiratory efforts. In addition, like any adapter between the ETT and the breathing circuit, they add mechanical deadspace. Figure 14.2 A digital apnea alert device attached to the endotracheal tube. The red circle highlights the airway adapter, which is connected to the digital display. An audible signal indicates air flow across the sensor with each breath and the apnea alarm can be adjusted to be 10–60 s in duration. Source: Thomas and Lerche [30]. Table 14.1 Definitions associated with the various forms of carbon dioxide measurement during ventilation. The use of quantified carbon dioxide as a monitoring tool in general anesthesia has multiple merits and can be accomplished in several forms (Table 14.1). In addition to the ability to provide information about metabolism, lung perfusion, and adequacy of ventilation, detection of carbon dioxide in respiratory gases can indicate integrity of the ETT and breathing circuit. Continuous CO2 monitoring provides real‐time data and allows for immediate detection of changes in ventilation and prompt intervention. It is primarily used to confirm ventilatory status (including respiratory rate, PETCO2, and inhaled CO2 [PICO2]). Importantly, capnometry/capnography is the most reliable primary method for identifying whether an endotracheal tube is correctly placed within the airway instead of the esophagus. Capnometry/capnography can also be instrumental in detecting life threatening cardiorespiratory complications. For example, the rapid decline in PETCO2 as detected by a capnograph may be the first and only reliable indicator of cardiopulmonary arrest in the anesthetized patient, as detailed below. Like other forms of patient monitoring in veterinary medicine, the implementation of CO2 measurement requires equipment that meets specific criteria to ensure its regular use and effectiveness. It is important for monitoring equipment to be affordable and readily available. Cost‐effective options enable wider accessibility, allowing veterinary professionals to incorporate CO2 monitoring into their standard protocols. Equipment should be easy to use and should not require extensive training or complex setup procedures. Additionally, lightweight, portable, and durable equipment offers flexibility for the rigors of daily use and enables monitoring in different areas of the veterinary hospital or during transport. In the case of monitoring ventilation, equipment should be ideal for the properties of CO2 and be able to distinguish it among other gases that are present with minimal interference. The gold standard for determination of PaCO2 is arterial blood gas analysis. An arterial sample is obtained either via puncture or from an indwelling catheter into a preheparinized syringe. Air bubbles are expelled, and the sample should be analyzed within 30 min or stored on ice. Both laboratory‐based and patient‐side blood gas analyzers are available that utilize a glass electrode covered with a CO2 permeable membrane (the Severinghaus electrode) to ascertain CO2 concentrations. Carbon dioxide from the blood sample diffuses across the membrane into a bicarbonate solution. Carbonic acid is created and devolves into H+ and HCO3–. The generation of H+ ions, and therefore pH, is logarithmically related to the concentration of CO2 in the sample, creating a voltage difference between the glass electrode and the reference electrode [31]. Disadvantages of arterial blood gas analysis include intermittent sampling and the need to puncture an artery. In addition, the required equipment may not be available in all veterinary clinical settings. Chemical detection is based on the principle that when CO2 is combined with water, carbonic acid is formed, which then dissociates into hydrogen ions and bicarbonate. In turn, the pH is decreased, and this change acts as a surrogate marker for detection of CO2 when using a pH‐sensitive indicator. These devices typically house a color changing, pH‐sensitive indicator within a clear window, which can be connected to standard endotracheal tube and breathing circuit components. For each device, a chart will reference which color change correlates with a range of CO2 measurements. Though the measurements are not precise, they have proven to be successful at detection of endotracheal intubation, prediction of return of spontaneous circulation in resuscitation, and monitoring of ventilation for many hours where quantitative methods are not available [32–34]. Advantages for this type of device include its light weight and low cost. Disadvantages include generation of semiquantitative results, conditional interpretation of color change, short‐term usability, and added resistance to flow. The widely used technology that possesses a majority of the aforementioned qualities is infrared (IR) spectroscopy. IR spectroscopy involves the analysis of the interaction between IR radiation and asymmetric, polyatomic molecules at distinct spectra. Homogeneous molecules, such as oxygen, do not absorb IR radiation, and techniques for oxygen measurement are discussed in Chapter 15. IR radiation consists of a range of wavelengths that fall between the visible and microwave regions of the electromagnetic spectrum. When a sample is exposed to IR radiation, the molecules within the sample absorb energy at specific frequencies that are characteristic of the chemical bonds present. The wavelength at which CO2 has the strongest absorption band is approximately 4.3 μm, which allows reasonable discrimination from other molecules that also absorb IR radiation and are commonly found in an expired breath (i.e., inhalant anesthetics). Notably, two molecules with IR absorption bands close to CO2 that may cause cross‐interference are water vapor and nitrous oxide (N2O). Once the absorption of IR radiation by CO2 in a sample of expired breath is measured, the principles of the Beer–Lambert law are applied: where A is the absorbance, ɛ is the molecular absorption coefficient, l is the length of the optical path, and c is the molecular concentration. Absorption of IR radiation at the specified wavelength is proportional to the concentration of the molecule (CO2) being measured. When the absorbance is compared to a known standard, the amount of CO2 present in respiratory gases can be quantified as a partial pressure (representative of the number of molecules present) or converted to a percent concentration (expressed as a proportion of the total). The essential elements for IR spectroscopy include: a source of IR radiation, a sample cell, and a photodetector [35]. IR capnographs may use an optical filter to transmit only the desired wavelength (nondispersive) or may separate the wavelengths spatially with prisms or diffraction (dispersive). Nondispersive IR spectroscopy is most commonly in use as it is more efficient. In general, IR radiation sources may be considered broad‐ or narrowband. One example of a broadband source is a heated element known as a “blackbody emitter.” In this case, filters are utilized to eliminate IR that is outside the desired range, and the constant source is mechanically chopped by a spinning wheel. The spinning wheel alternately blocks and allows the IR radiation to pass through to the sample or detector. This chopping action creates a time‐varying signal, which is important for separating the desired IR signal from any background noise or interference. Multigas analyzers employ several specific narrowband filters to allow for the measurement of halogenated agents along with CO2 and may also include integrated technology for measurement of oxygen. An example of a narrowband IR source is laser‐based molecular correlation spectroscopy, or Microstream technology. This uses a unique approach to emit a band of IR radiation that is highly precise for CO2. In Microstream technology, a glass electrode that contains several gases, including nitrogen and CO2, is exposed to a radio frequency voltage. Consequently, the nitrogen molecules experience excitation and their interaction with CO2 causes excitation of those molecules. When CO2 returns to its resting state, it emits radiation, which is used as the narrow spectrum IR source. This IR source is then electronically modulated in order to allow sampling every 25 ms. Ultimately, the IR light is transmitted through the sample chamber, and the filtered and modulated signal reaches a detector where the intensity is measured, processed, and directed to the user interface where it is displayed. The IR absorption band for CO2 lies between two strong water vapor absorption bands, and water vapor can interfere with CO2 measurement in several ways. When exhaled breath passes through the respiratory system, it picks up water vapor, which can significantly contribute to the total gas volume. Water vapor can condense at the sample cell, where it will absorb IR radiation, leading to a falsely increased CO2
14
Monitoring Ventilation
Introduction
Physiology of aerobic respiration
Carbon dioxide and acid–base implications
Importance of monitoring ventilation
Monitoring standards
Physical methods of monitoring ventilation
Apnea monitors
Capnometry
The measurement of the partial pressure of carbon dioxide in respiratory gas
Capnometer
A diagnostic tool that measures the partial pressure of carbon dioxide in respiratory gas
Capnogram
A cyclical waveform of respiratory gas carbon dioxide plotted against time or expired volume
Capnography
The continuous analysis and recording of the measurement of the partial pressure of carbon dioxide in respiratory gases over time or expired volume
Capnograph
The instrument that analyzes CO2 in respiratory gases and displays a capnogram
Quantitative monitoring of carbon dioxide
Methods of carbon dioxide measurement
Blood gas analysis
Chemical detection (colorimetry)
Infrared spectroscopy
Infrared radiation source
Problems with infrared spectroscopy
Stay updated, free articles. Join our Telegram channel
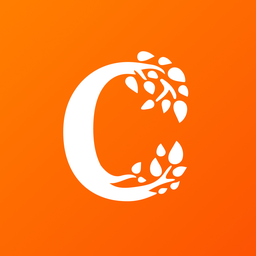
Full access? Get Clinical Tree
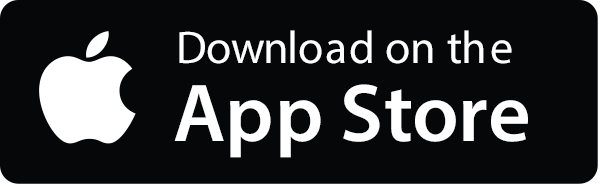
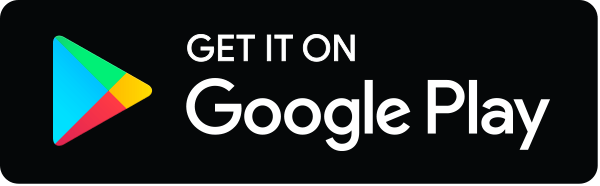