Chiara Adami Department of Veterinary Medicine, Cambridge Veterinary School, University of Cambridge, Madingley Road, Cambridge, UK The goal of physiologic monitoring during anesthesia is to ensure an adequate oxygen supply to maintain mitochondrial aerobic metabolism and, ultimately, cellular function. Several techniques have been developed to monitor oxygenation for clinical and research purposes. Currently, there is no gold standard technique for monitoring oxygenation that combines quick response time, suitability for in vivo assessment, ease of use, inexpensiveness, and ability to evaluate local and regional tissue oxygenation. The available techniques provide information reflecting the status of the whole body, making them unsuitable to detect regional and local alterations until they are well established and may have resulted in irreversible organ damage. On the other hand, many techniques that specifically investigate oxygen concentrations at the cellular level are only applicable in vitro, on prepared isolated tissues, or are poorly applicable to the clinical setting due to cost of the equipment, difficulty of use, and limited experience in veterinary patients. This chapter describes the techniques available for real‐time monitoring of tissue oxygenation and metabolism in living animals, with a focus on the technical aspects, clinical use, and limitations. Although the terms hypoxia and hypoxemia are often used interchangeably, they are not the same (Table 15.1). Hypoxia is a condition in which the supply of oxygen to tissues fails to ensure normal cellular metabolism, whereas hypoxemia describes a state of low arterial oxygen tension (interchangeably referred to as “oxygen partial pressure”). Hypoxemia is a frequent cause of tissue hypoxia, but it is not the only one, with other major causes being anemia, histotoxicity, and reduced forward blood flow. The causes of hypoxemia are classified in various ways in the literature and include low inspired oxygen partial pressure, hypoventilation, and venous admixture. Venous admixture represents all the ways venous blood can move from the right to the left side of the circulation without being oxygenated, including low ventilation–perfusion (V/Q) ratios, anatomic or physiologic shunt, and diffusion impairment. Some authors will also include reduced venous oxygen content secondary to either low cardiac output or high oxygen extraction by the tissues (e.g., seizures) [1]. The alveolar gas equation allows the calculation of the expected partial pressure of oxygen in alveoli (PAO2) and, subsequently, the evaluation of pulmonary gas exchange. It is commonly written as where FIO2 is the fraction of inspired oxygen (21% in room air), PB – PH2O is the estimated barometric pressure minus the partial pressure of water in fully humidified inspired gas, PaCO2 is the partial pressure of carbon dioxide in arterial blood, and R is the respiratory quotient. The respiratory quotient is an expression of the ratio of CO2 elimination over O2 uptake, and it varies slightly with diet and metabolic state but is typically estimated at 0.8. Knowledge of the expected PAO2 in comparison to the measured arterial partial pressure of oxygen (PaO2) allows for the evaluation of pulmonary gas exchange. Table 15.1 Comparison of the causes of tissue hypoxia (low oxygen concentrations in tissues) with causes of hypoxemia (low oxygen partial pressure in arterial blood). Venous admixture includes the various ways blood can move from the right to the left side of circulation without being oxygenated. V, ventilation; Q, perfusion. Areas of high V/Q do not cause venous admixture. The following equations are fundamental in the evaluation of oxygen transport and utilization. Oxygen content of arterial blood (CaO2) is the total amount of oxygen in arterial blood and is calculated as follows: where Hb is the total hemoglobin concentration and SaO2 is the arterial saturation of hemoglobin with oxygen (see discussion of functional versus fractional oxygen saturation below). The first term of the equation represents the amount of oxygen bound to hemoglobin, and the second term characterizes the quantity of oxygen dissolved in plasma. The vast majority of the oxygen in blood is carried by hemoglobin (~98%), whereas only a small amount is dissolved (~2%). Oxygen delivery ( where CO is cardiac output in L/min. It is important to note that adequate oxygen delivery is dependent on adequate cardiac output, hemoglobin concentration, and PaO2. This calculation does not consider abnormal hemoglobin species such as methemoglobin or carboxyhemoglobin. Oxygen consumption ( where C(a– The oxygen extraction ratio (O2ER) is simply Causes of increased O2ER include anything that increases oxygen consumption (e.g., seizures, hyperthermia, and sepsis) or decreases oxygen delivery (including hypoxemia and anemia). A low O2ER is usually due to decreased oxygen consumption as caused by anesthesia, mechanical ventilation, and hypothermia. A major factor limiting routine clinical use of O2ER is that it requires measurement of mixed venous O2 via a pulmonary arterial catheter. Gray to blue mucous membrane discoloration (cyanosis) indicates the presence of deoxygenated blood in the observed tissue. The detection of cyanosis may be unreliable as a measure of tissue oxygenation, however, as it depends upon both the visual acuity of the observer and the local concentration of deoxygenated hemoglobin; moreover, it may be affected by lighting conditions [2]. A minimal concentration of deoxygenated hemoglobin (5 g/dL) is required to cause a degree of cyanosis sufficient to be detected visually [3]. Therefore, an anemic animal might be hypoxemic without showing cyanosis because the decreased hemoglobin concentration would be saturated with oxygen even in the presence of considerably reduced partial pressures of oxygen in the blood. Drug‐induced peripheral vasoconstriction (e.g., as caused by α2‐adrenergic receptor agonists) can cause peripheral cyanosis. When peripheral blood flow slows down due to vasoconstriction, oxygen can diffuse into the tissues over a longer time (i.e., oxygen extraction increases), leaving more deoxygenated hemoglobin in the capillary bed [4]. The partial pressure of oxygen (PO2) can be measured in a liquid (e.g., blood), a gas (e.g., inspired gas), or at the skin using technology based on the polarographic Clark electrode, which is composed of a silver anode, a platinum cathode, and a gas‐permeable membrane through which oxygen molecules can diffuse freely. When a polarizing voltage is applied to the cathode, a current that is proportional to the partial pressure of oxygen is generated between the anode and the cathode, where oxygen is reduced. The following information focuses on the measurement of PO2 in arterial blood, mixed venous blood, or diffusing through the skin. Measurement of oxygen in inspired and expired gas will be discussed in a separate section later in this chapter. Analysis of arterial blood gases includes the measurement of partial pressures of oxygen and carbon dioxide in the arterial blood, reported in North America in mmHg. Partial pressures are often misinterpreted as a measure of how much oxygen and carbon dioxide are in the blood; however, instead, they indicate the pressure exerted by the dissolved, unbound molecules of each gas against the measuring electrode. The only parameter that quantifies the amount of oxygen in the blood, expressed as mL/dL, is the CaO2, which is calculated from both SaO2 and PaO2 values as outlined above. Arterial oxygen saturation of hemoglobin is a function of PaO2, and their relationship is expressed by the oxygen–hemoglobin dissociation curve. Arterial blood gas analysis remains the most popular method for advanced monitoring of oxygenation in animals, with PaO2, SaO2, and the calculated alveolar–arterial (A‐a) gradient used clinically to assess oxygenation. The A‐a gradient is the measured arterial PO2 (PaO2) subtracted from the PAO2, calculated from the alveolar gas equation. A normal A‐a gradient is less than 20 mmHg. As the A‐a gradient widens during oxygen supplementation, the ratio of PaO2 to fractional inspired oxygen (PaO2/FIO2), also known as the “Horowitz index,” may be calculated instead. At sea level, the normal PaO2/FIO2 ratio is approximately 400–500; values below 400 suggest the presence of gas exchange abnormalities. The PaO2/FIO2 ratio is a widely used clinical indicator of pulmonary function; nevertheless, its reliability is controversial. The main disadvantages of using the PaO2/FIO2 ratio to guide clinical decisions are its dependence on barometric pressure and its inability to distinguish alveolar hypoventilation from other causes of hypoxemia, such as V/Q mismatch and shunt [5,6]. Moreover, the PaO2/FIO2 ratio does not indicate CaO2, which depends on hemoglobin concentration, nor does it provide information on oxygen delivery to tissues [5,6]. The biggest limitation of arterial blood gas analysis is its inability to detect regional or local changes in tissue oxygenation. The non‐continuous nature of monitoring and the costs associated with repeated testing also represent clinically relevant limitations. In addition, arterial blood gas analysis requires anaerobic sampling and either immediate analysis or special handling. There are no unanimous guidelines with respect to the frequency of arterial blood sampling and analysis for proper monitoring of the oxygenation status in animals. In human patients undergoing thoracoscopy, it takes only 5, 10, or 20 min for the arterial partial pressure of oxygen to change by 10%, 20%, or 40% of its initial value, respectively [7]. This finding may suggest that frequent sampling would be necessary for prompt detection of severe changes in blood oxygenation in animals with compromised respiratory function. Another potential disadvantage of monitoring oxygenation through arterial blood gas analysis is the need for placing an indwelling arterial catheter to facilitate frequent blood sampling. Although this procedure is usually performed in animals with no or minor complications, hematoma formation, hemorrhage, vasculitis, and thrombus formation are possible sequelae. Moreover, obtaining arterial samples can be technically challenging in very small animals, and frequent blood sampling from small patients may result in iatrogenic anemia. Mixed venous blood refers to blood in the pulmonary artery and is representative of venous blood from all organs and tissues. The partial pressure of oxygen in mixed venous blood (P Transcutaneous blood gas monitoring systems are commercially available and have been used in dogs, sheep, mice, rats, and cats [9–12]. Blood gases diffusing through the skin can be detected and quantified by a sensor applied to the body surface. The digital sensors used to measure transcutaneous gases incorporate a Clark electrode for PO2 measurement and a Severinghaus‐type sensor to detect PCO2. There are models that incorporate pulse oximetry instead of the Clark electrode [13]. The PCO2 is measured from the pH of an electrolyte solution separated from the skin by a membrane that is highly permeable to CO2; the changes in pH of the electrolyte solution are proportional to the logarithm of the changes in PCO2. The sensor must be heated to 41 °C/105.8 °F prior to use to enhance local blood perfusion and CO2 diffusion though the intact skin. The main advantage of transcutaneous monitoring is that this technique is suitable for continuous and non‐invasive monitoring of oxygenation and ventilation. It provides real‐time information on partial pressures of oxygen and carbon dioxide, enabling clinicians to detect changes in respiratory status more quickly than with intermittent blood gas analysis. Moreover, its use avoids the drawbacks and costs associated with frequent arterial blood sample collection and analysis. Transcutaneous blood gas monitoring is widely used in human medicine, especially in neonatal patients, to obtain non‐invasive and continuous blood gas measurements during mechanical ventilation or procedures potentially resulting in impaired gas exchange, such as bronchoscopy and bronchial lavage [14]. Other applications include monitoring of respiratory function associated with sleep apnea or other conditions potentially resulting in hypoxemia and hypercapnia [15]. In the veterinary literature, however, information pertaining to transcutaneous blood gas monitoring is limited and seems to suggest that transcutaneous measurement of PCO2 lacks accuracy [10–12,16–19]. In dogs, transcutaneous PCO2 overestimated PaCO2 by more than 10 mmHg; moreover, quick changes in PaCO2 caused by alterations of the breathing pattern were detected by transcutaneous monitoring with a 6‐min delay [12]. It was, therefore, concluded that, while transcutaneous PCO2 monitoring might be useful for detecting changes or trends in PaCO2, it was not sufficiently accurate to be used as a valid surrogate of PaCO2. Very similar conclusions were drawn from a study conducted in rats, whereas in mice a good correlation was established between transcutaneous PCO2 and PaCO2 [10,11]. An experimental study conducted on anesthetized dogs demonstrated that decreases in cardiac output caused by iatrogenic hemorrhage resulted in less accurate PCO2 readings; however, correlation with PaO2 values improved after fluid resuscitation [16]. In critically ill dogs, the level of agreement between transcutaneous and arterial readings was lower than that reported in human patients, and the transcutaneous monitor consistently overestimated both PaO2 and PaCO2 [19]. In a slightly different application, some studies report the use of transcutaneous PCO2 to evaluate skin graft viability in experimental dogs [17,18]. One study investigated the use of transcutaneous oxygen monitoring in Louisiana pine snakes [20]. The percentage of hemoglobin saturated with oxygen is measured clinically either by CO‐oximetry or pulse oximetry. It is important to note that these methodologies report slightly different values. A CO‐oximeter can measure all forms of hemoglobin, including the abnormal forms that cannot bind oxygen: carboxyhemoglobin (COHb), methemoglobin (MetHb), and sulfhemoglobin (SulfHb). Consequently, CO‐oximeters report the fractional hemoglobin saturation (FO2Hb), that is, the percent of total hemoglobin that is saturated by oxygen:
15
Monitoring Oxygenation
Introduction
Hypoxia versus hypoxemia
Alveolar gas equation
Causes of hypoxia
Causes of hypoxemia
Hypoxemic hypoxia
Low arterial oxygen tension
Anemic hypoxia
Low hemoglobin concentration
Stagnant/ischemic hypoxia
Decreased perfusion
Histotoxic hypoxia
Inability of mitochondria to utilize delivered oxygen
Decreased inspired oxygen partial pressure
Hypoventilation
Venous admixture
Areas of low V/Q
Physiologic or anatomic shunt
Diffusion impairment
Oxygen content, delivery, and consumption
Oxygen content
Oxygen delivery
O2) is the amount of oxygen delivered to the tissues each minute and is calculated as follows:
Oxygen consumption
O2) is the amount of oxygen used for metabolic processes per minute and is calculated via the Fick equation:
)O2 is the difference between systemic arterial and mixed venous (i.e., pulmonary arterial) oxygen content. If both
O2 and
O2 are known, the oxygen extraction ratio can be calculated.
Oxygen extraction ratio
O2 divided by
O2:
Visual assessment of oxygenation
Measuring oxygen partial pressure
Arterial blood gas analysis
Mixed venous oxygen partial pressure
O2) reflects metabolic activity and oxygen consumption. The normal P
O2 while breathing room air is around 40 mmHg, corresponding to a saturation of around 70% (see discussion of mixed venous O2 saturation below). The P
O2 can be used to calculate mixed venous O2 content,
O2, and O2ER; however, the measurement of P
O2 requires placement of a pulmonary arterial catheter. This procedure is rarely performed in veterinary patients and has fallen out of favor in critically ill human patients [8].
Transcutaneous measurement of blood gases
Measuring hemoglobin saturation with oxygen
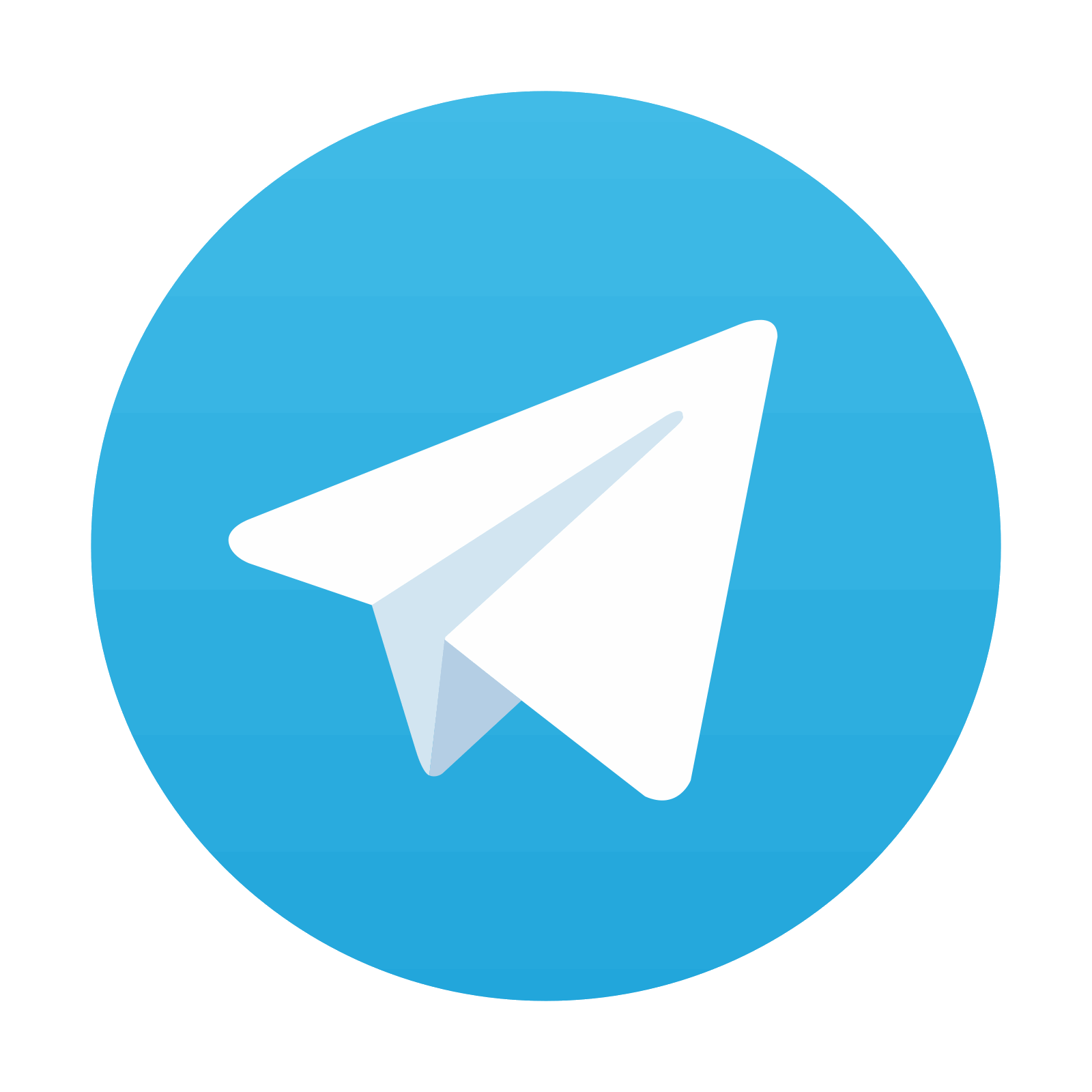
Stay updated, free articles. Join our Telegram channel
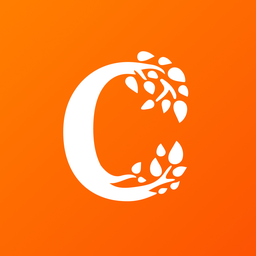
Full access? Get Clinical Tree
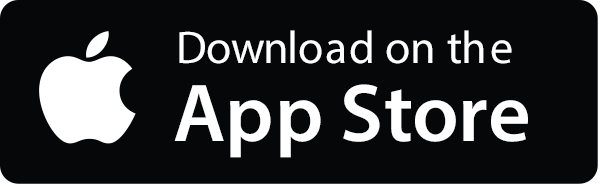
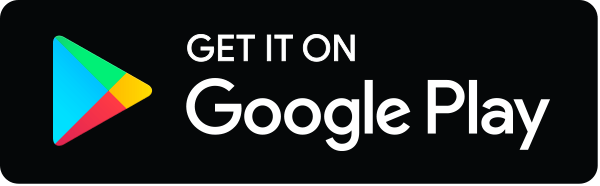