Database
Web address
Description
WormBase
Gene summary pages containing functional, structural, and phylogenetic information. Links to other databases (i.e., interactome, microarray databases, expression patterns, etc.), related to query genes or ORFs. Links to published articles and C. elegans meeting abstracts as well
Caenorhabditis elegans WWW Server
Collection of hyperlinks to sites relevant to the study of C. elegans and other nematodes
WormBook
An online, open-access, site with peer-reviewed chapters describing nematode biology
WormAtlas
An online database of the structural anatomy and its relationship to C. elegans behavior, with a complete nervous system wiring diagram at the EM level
C. elegans also has a fully sequenced genome and shares approximately 50% of its genes with humans, and there are many bioinformatics resources available to worm researchers, many of which can be accessed through links described in Table 1. Notably, ∼70% of genes that are known to cause a genetic disease in humans have an ortholog in C. elegans. Application of C. elegans toward human disease research has already provided insights into the function of specific gene products linked to a variety of human movement disorders, such as dystonia (2), Huntington’s disease (3), ALS (4), and Parkinson’s disease (this volume).
C. elegans also exhibits a variety of behaviors that can be elicited using forward and reverse genetic strategies. As an example, Dr. Sydney Brenner, who pioneered the use of C. elegans as a model organism in the 1960s, initially worked with his group on a forward mutagenesis screen to identify mutants with abnormal locomotion (the uncoordinated, Unc, phenotype). Forward genetic screens, beginning with a phenotype of interest, often involve the use of a mutagen, such as EMS. This mutagen most often produces point mutations (G/C–A/T transitions) (5). Following successful screening for mutant phenotypes, identification for the genetic lesion(s) typically occurs. Their screen identified 77 unc genes that included both neuronal and muscular defects (6) in many genes that have since been identified as evolutionarily conserved components of muscle cells, synaptic transmission, or neurotransmitter release. Many forward genetic screens have been performed since this time, based on a variety of phenotypes (embryonic, morphological, reproductive, and neurological).
Reverse genetic screens require knowledge of the gene sequence of interest. Since the completion of the genome projects for C. elegans, humans, mouse, and other well-studied laboratory species, the field of comparative genomics has allowed C. elegans researchers to extensively use RNAi screening in their research to knockdown target genes and then screen for potential phenotypes. In nematodes, this method simply involves injecting, soaking, or feeding worms dsRNA that is complementary to the targeted gene of interest. The expression of the candidate gene will be greatly reduced, or silenced, in the next generation. Relevant to human movement disorders, whole genome-wide screens, as well as smaller, hypothesis-based screens, have been performed to examine a variety of phenotypes, such as aging (7), protein aggregation in ALS (4), and spinal muscular atrophy (8).
1.1 Overview of the C. elegans Nervous System
Despite its evolutionary distance from humans, the neurons of C. elegans display most of the hallmarks of mammalian neuronal function including ion channels, neurotransmitters (dopamine, serotonin, acetylcholine, GABA, etc.), vesicular transporters, receptors, and synaptic components (9, 10). Compared with the ∼100 billion neurons of the human brain or even the 10,000 neurons of Drosophila, C. elegans hermaphrodites have exactly 302 neurons. Thus, of the 959 somatic cells comprising the adult animal, approximately 1/3 of these cells are neurons. The nervous system has also been reconstructed in great detail to reveal the anatomy and complete connectivity of all the neurons (11). This is a remarkable accomplishment given that the cell bodies of worm neurons are approximately 2 μm in diameter (12). Worms have 118 different types of neurons that have been classified into groups based on differences in morphology, connectivity, and function, including mechanosensation, chemosensation, and thermosensation. Worms also have approximately 7,000 synapses.
Precisely, eight neurons within a C. elegans hermaphrodite are dopaminergic. Six of these neurons are located in the anterior region of the animal (Figs. 1 and 2a, b), and are identified by their lineage names as the four cephalic (CEP) neurons and the two anterior deirid (ADE) neurons, while the other two DA neurons are in the posterior of the animal are called posterior deirid (PDE) neurons (Fig. 2a). The two dorsal CEPs are post-synaptic to the ADE neurons, while the two ventral CEPs are not post-synaptic to the ADEs (Fig. 1).
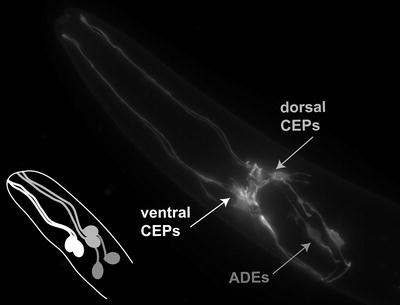
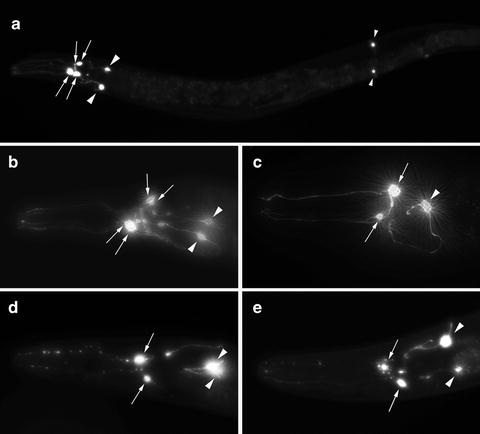
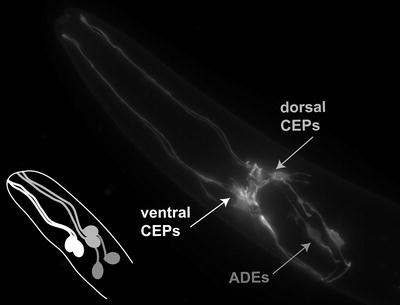
Fig. 1.
A fluorescent photomicrograph depicting the anterior-most region of a Caenorhabditis elegans hermaphrodite. The DA neurons are illuminated using GFP driven from the DA transporter promoter (P dat-1 ::GFP). The six cell bodies and neuronal processes include two pairs of cephalic (CEP) neurons and one pair of anterior deirid neurons (ADEs). The line drawing is a representation of the association of the DA neurons to each other; the dorsal CEPs (light gray ) are post-synaptic to the ADE neurons (darker gray ) while the ventral CEPs (white) are not post-synaptic to the ADE neurons.
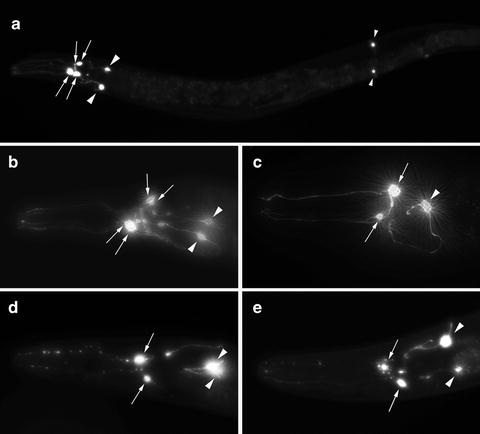
Fig. 2.
Representative images of Caenorhabditis elegans DA neurons in normal and degenerative states. (a) An image depicting all eight DA neurons in a C. elegans hermaphrodite. The six anterior DA neurons are visible on the left (two pairs of CEP neurons (arrows) and one pair of ADE neurons (large arrowheads)), where the cell bodies and processes are highlighted. Two posterior deirid (PDE) neurons are indicated with small arrowheads on the right side of this image. (b) A magnified view of the anterior region of a worm displaying the cell bodies of the four CEP neurons (arrows) and the two ADE neurons (arrowheads). (c) An image displaying a 7-day-old worm that is co-expressing GFP and α-syn in DA neurons; this animal has lost three out of six anterior DA neurons. In this example, only two of the four CEP neurons (arrows) and one of two ADE neurons (arrowhead) remain. Most worms within this population are missing one or more anterior DA neurons when they are adults. (d, e) Exposure to 6-OHDA causes progressive degeneration of CEP neurons. (d) In this example, two of the four CEP neurons have already degenerated, while the remaining CEP neurites display blebbing and cell body rounding (arrows). The two ADE neurons in this animal are still intact (arrowheads). (e) This worm is undergoing further degeneration, whereby the two remaining CEP neurites are almost invisible and the associated cell bodies are also degenerating (arrows); the two ADE neurons are also beginning to degenerate (arrowheads).
There is evolutionary conservation of the pathways involved in the processing, packaging, and transport of DA, thus enabling researchers to utilize the worm for studying various cellular aspects of DA neuron biology. In this regard, several behavioral phenotypes have been identified as being specific for DA signaling in C. elegans. For example, upon exposure to exogenous DA, worms exhibit a decrease in egg laying, locomotion, and defecation. Worms also normally exhibit decreased locomotion upon entering a bacterial lawn, a DA-associated behavior called the basal slowing response (13). Researchers identified that mutations in cat-2 resulted in no basal slowing response. This gene encodes worm tyrosine hydroxylase (TH), the rate-limiting enzyme for DA synthesis. These studies suggest that there is a role for DA in mechanosensation and food sensing (14). The basal slowing response phenotype is a potential readout for DA neuronal function and dysfunction for C. elegans DA models, however, it is time consuming and does not readily lend itself to screening procedures. Notably, a recently published article described an automated worm tracking system that could potentially be modified to quantify the basal slowing response in multiple individual worms in parallel (15).
1.2 C. elegans as a Model for Parkinson’s Disease
Orthologs of six familial Parkinson disease (PD) genes have been identified in C. elegans and mutant alleles are available for these C. elegans PD gene orthologs (Table 2), thus enabling genetic analyses. For example, in a recent paper, C. elegans researchers examined double mutants in LRRK2 and PINK1 (lrk1 and pink1, respectively), and determined that the gene products have an antagonist role in cellular stress response and regulation of neurite outgrowth (16). In this manner, single and double mutants of C. elegans PD gene orthologs provide an opportunity to evaluate pathways and interrelationships, as well as screen for potential modifiers of PD. Notably, the C. elegans genome does not have a homolog for human α-synuclein (α-syn) (Table 2). This exclusion has allowed researchers to overexpress wildtype (WT) or mutant α-syn in the nematode α-syn null genetic background without concerns for endogenous α-syn or the risk of eliciting a dominant negative effect (17–22).
Table 2
Summary of human familial PD genes, corresponding Caenorhabditis elegans orthologs and mutants
PD Gene | PD Protein | C. elegans ortholog | E-Value | C. elegans chromo-some location | C. elegans allele name | Type of mutation |
---|---|---|---|---|---|---|
PARK1 | SNCA/α-syn | n/a | ||||
PARK2 | PRKN/parkin | pdr-1 | 3.4e-38 | III | gk448 | Knockout allele; superficially wildtype |
PARK5 | UCHL-1 | ubh-1 | 1.2e-33 | V | n/a | |
PARK6 | PINK1 | pink-1 | 7.8e-53 | II | ok3538 | Knockout allele |
PARK7 | DJ-1 | djr-1.1 djr-1.2 | 1.6e-45 8.9e-36 | II V | tm918 tm1346 | Both are knockout alleles; double mutant created |
PARK8 | LRRK2 | lrk-1 | 5.5e-66 | I | tm1898 | Deletion; homozygous viable |
PARK9 | ATP13A2 | catp-6 | 2.5e-180 | IV | ok3473 | Knockout allele; homozygous viable |
PARK11 | GIGYF2 | n/a | ||||
PARK13 | HTRA2 | n/a |
1.2.1 Use of C. elegans as a Screening Tool to Identify Putative PD Susceptibility Genes
An expeditious route toward discerning genetic contributors to PD involves the use of animal systems amenable to screening methods to identify potential genetic modifiers. In this regard, the use of C. elegans has the potential to greatly accelerate the discovery of neuroprotective factors for PD (23) and other diseases of protein misfolding. These assays consist of forward and reverse genetic screens, as well as chemical screens. An example of a relevant forward genetic screen in C. elegans was performed for enhancers of polyglutamine aggregation in muscle cells, whereby it was determined that a general imbalance in protein homeostasis in post-synaptic muscle cells can occur if there is an increase in acetylcholine (ACh) signaling, or defective GABA signaling (24). Another example of a forward genetic analysis involved screening C. elegans DA neurons for insensitivity to 6-hydroxydopamine (6-OHDA), whereby new alleles of the DA transporter (DAT-1) were identified (25).
There are many examples of reverse genetic, RNAi, screens using the full genome in C. elegans. Several of these screens scored phenotypes related to protein misfolding. For example, worms expressing α-syn::YFP in body wall muscle cells were screened for a modulation in protein misfolding and 80 candidates were identified (18). The candidates clustered into a few biological categories. Notably, quality control and ER/Golgi vesicle trafficking gene products were identified as those that, when knocked down, increased protein aggregation. Another category of gene products identified were aging related, but these were suppressors of protein aggregation.
Recently, candidate RNAi screens, based on the mature bioinformatics available within the C. elegans field, have become more common. Our laboratory performed a hypothesis-based screen for genetic modifiers of α-syn::GFP misfolding in body wall muscle cells and identified 20 candidate genes. Five of these candidates were subsequently validated as having a protective role in DA neurons as well (17). The candidates identified from this screen included orthologs of known recessive PD genes, DJ-1 and PINK1, VPS41, a vesicular protein necessary for lysosomal trafficking and biogenesis, ATG7 an autophagy-associated regulatory gene, and ULK2, a conserved serine-threonine kinase also related to the yeast autophagy protein, Atg1p.
The outcome of this screen represented an exciting group of gene products with implications for PD, both as potential susceptibility markers and novel targets for therapeutic development. For example, knockout of the lysosomal protein ATG7 has been reported to produce a neurodegenerative phenotype in mice (26). Another neuroprotective gene product identified from our screen, VPS-41, is the nematode homolog of mammalian and yeast VPS41, a protein implicated in trafficking from the Golgi to the vacuole/lysosome in yeast (27). Little was known about the function of VPS41 in mammalian systems except that it is strongly expressed within DA neurons of the substantia nigra pars compacta. However, subsequent mammalian studies have since revealed that VPS41 over-expression is protective against rotenone- and 6-OHDA-induced toxicity in SHSY5Y cells (28). Finally, ULK2 was one of six genes significantly associated with single nucleotide polymorphisms in a genome-wide association study (GWAS) of PD patients (29). Therefore, it is clear that establishing a functional screening paradigm for modifiers of PD-related phenotypes in C. elegans has successfully yielded an intriguing collection of effectors that demonstrate the predictability of the nematode model to identify targets with high translational potential.
1.2.2 Use of C. elegans to Validate Therapeutic Targets and Chemicals
Our ability to successfully predict the probability of PD among individuals is dependent upon knowledge about genetic susceptibility factors that render certain populations at risk. In attempting to discern genetic factors associated with PD, scientists working with a variety of different organisms have generated many lists of candidate genes and proteins, but most of these leads remain mechanistically undefined. In this regard, application of C. elegans in a directed manner can facilitate the functional evaluation of leads originally identified in other species (yeast, cell culture, mouse, and human genomic studies). As described previously, a distinct advantage of using C. elegans for functional investigation of gene activity and validation is that large populations of isogenic animals can be propagated and analyzed, leading to an unequivocal level of accuracy when evaluating neurodegeneration. As shown in Fig. 2, we have established isogenic lines of transgenic C. elegans that overexpress human α-syn in DA neurons (P dat-1 ::α-syn + P dat-1 ::GFP) that enable rapid evaluation of factors (drugs and genes) that protect or enhance DA neurodegeneration. This worm strain has been successful in rapidly predicting genes that have significant consequences for neuronal survival in mammalian systems. For example, we investigated three Rab gene products, involved in regulating vesicular trafficking, that were originally identified from yeast screens as effectors of α-syn-dependent toxicity (30, 31). Suppression of cytotoxicity was recapitulated in yeast cells, Drosophila, rat neuronal cell cultures, and in C. elegans, where we showed that elevated expression of specific Rab GTPases rescue DA degeneration induced by α-syn overexpression. For example, overexpression of mammalian Rab1 successfully by-passed an α-syn-associated block of ER to Golgi trafficking (30). New molecules that impact this cellular mechanism have since been discovered in yeast and subsequently validated across multiple organisms, including C. elegans (32).
There is a growing body of literature demonstrating the utility of C. elegans for pharmacological research. A variety of compounds have been uncovered, ranging from those associated with modulating neurotransmitter activity (33, 34) anesthetics (35), lifespan extension (36), and a new calcium channel antagonist (37). Our laboratory has used C. elegans to conduct a small molecule screen to identify compounds that positively modulate the activity of the neuroprotective chaperone-like protein, torsinA, in vivo (38). Deficit in torsinA function is responsible for a human movement disorder termed early-onset torsion dystonia, and is linked to an in-frame 3-bp deletion in the human DYT1 gene. These same molecules were later shown to restore functional capacity of torsinA activity in human DYT1 dystonia patient fibroblasts and reverse behavioral abnormalities in a DYT1 knock-in mouse model of early-onset torsion dystonia (38). Of relevance to PD, worms are being exploited as a system for therapeutic drug screening (39) and functional validation of drugs with a therapeutic potential for PD that have been identified in other systems (32, 40, 41).
2 Large-Scale RNAi Screening Using an α-syn Misfolding Phenotype in Non-neuronal Cells
Central to the formation of Lewy bodies, a primary pathological hallmark of PD, is α-syn (42, 43). Importantly, it was demonstrated that genomic multiplication of the WT α-syn gene results in PD, indicating that overexpression of this protein alone could lead to the disease (44). A recent GWAS on approximately 800 familial PD cases further supports that the SCNA (α-syn) locus is a major susceptibility factor (45). The effects of overexpression, mutation, misfolding of α-syn has led to the hypothesis that the cellular clearance of this small, aggregation-prone protein is critical to avoiding the neurodegenerative state.
As displayed in Table 3, there are two models of α-syn misfolding in C. elegans body wall muscle cells that can be evaluated for the consequences of α-syn overexpression and misfolding in vivo. In both models, α-syn is fused at the C-terminus to either GFP or YFP, and inclusions are visualized in body wall muscle cells. These two transgenic models were used in independent RNAi screens for gene products that modulate protein misfolding (17, 18). In both transgenic models, the aggregates become more abundant as the animals get older and can be scored over time as worms develop and age (Fig. 3).
Table 3
Caenorhabditis elegans models of α-syn expression and their corresponding phenotypes
α-syn expression pattern | Transgenic construct(s) | Phenotypes | Reference |
---|---|---|---|
WT α-syn expression patterns | |||
Body wall muscle cells | P unc-54 ::α-syn::GFP P unc-54 ::α-syn::YFP | α-syn accumulation visualized by misfolded GFP; used in RNAi screen α-syn accumulation visualized by misfolded YFP; used in RNAi screen | (17) (18) |
Pan neuronal | P aex-3 ::α-syn P snb-1 ::α-syn P unc-51 ::α-syn | Reduced motor movement; DA neurodegeneration visualized by GFP Mitochondrial stress Endocytosis defects; motor/developmental defects | (19) (20) (21) |
DA neuron specific | P dat-1 ::α-syn P dat-1 ::α-syn P dat-1 ::α-syn | DA neurodegeneration visualized by GFP DA neurodegeneration visualized by GFP Slightly reduced DA levels; α-syn accumulation in DA neurons | (19) (22) (21) |
Mutant α-syn expression patterns | |||
Pan neuronal DA neuron specific | P aex-3 ::α-syn A53T P unc-119 ::α-syn A53T P unc-51 ::α-syn A30P and A53T P dat-1 ::α-syn A53T < div class='tao-gold-member'>
Only gold members can continue reading. Log In or Register a > to continue
![]() Stay updated, free articles. Join our Telegram channel![]() Full access? Get Clinical Tree![]() ![]() ![]() |