7 Meat Preservation and Processing Normally we do not eat carcass meat immediately after slaughter but, depending on the species concerned, wait for a period of between 1 and perhaps 21 days to elapse before doing so. During this time chemical changes in the musculature convert it into meat, generally improving its palatability. There are three main processes that are important: the muscles acidify, they go into rigor mortis and ‘conditioning’ takes place. In conditioning, also referred to as ageing, the meat becomes more tender and its flavour improves. These processes take place against a background of the carcass cooling, so that its surface becomes drier and the contained fat becomes harder. The hardening of the fat contributes to the general stiffening or firming of the carcass, facilitating the cutting of it into primal joints, and subsequent butchery. Traditionally, red meat carcasses are cooled by holding them overnight in refrigerated rooms operating at about 2°C. The muscle temperature therefore eventually falls from about 37°C to nearer 5°C in the first 24 hours post-mortem. The overall structure of muscle is determined by a connective tissue skeleton. Within this is a hierarchical arrangement consisting of bundles of muscle fibres, the fibres themselves (equivalent to muscle cells), the contractile elements within the fibres, the myofibrils and the myofilaments within these. The myofilaments are of two sorts: thick filaments consisting mainly of the protein myosin, and thin filaments consisting mainly of the protein actin. The thick and thin myofilaments interdigitate and form the basis of the contractile mechanism. Muscle contraction is caused by myosin and actin molecules reacting together such that the thick and thin filaments slide over one another, shortening the muscle’s length. The contraction is stimulated by calcium ions released from the sarcoplasmic reticulum membrane system within the muscle fibres, and requires adenosine triphosphate (ATP) as the energy source. ATP is also needed to maintain the working of the membrane systems. In the living animal ATP is produced from fatty acids or glucose delivered in the blood, or from glycogen stored in the muscle. Blood glucose and muscle glycogen are metabolized in very similar ways. First they are broken down to pyruvic acid (a process known as glycolysis), which produces a small amount of ATP, and then the pyruvic acid is oxidized completely to carbon dioxide and water (by oxidative decarboxylation and phosphorylation), which produces a lot more (a net yield of about 34 ATP molecules for every glucose molecule broken down). The oxygen for oxidation comes from the lungs via the blood. If it is not used immediately the ATP is stored in the muscle as creatine phosphate (CP). Muscles contain only relatively small amounts of ATP but much larger amounts of CP. However, immediately the available ATP is used, for example in supplying the energy for muscle contraction during exercise, it is replaced by the breakdown of CP. The reaction is reversible so that, in the period of recovery after exercise, ATP can be synthesized and used to replenish CP stores. When an animal is killed, glucose and oxygen are no longer available via the blood stream. However, there is a continuing need for ATP to maintain membrane ion pumps and cell integrity. To continue to make ATP the muscle breaks down stored glycogen to pyruvic acid. Because there is no oxygen to complete the breakdown to carbon dioxide and water, and under the conditions found in the muscle at this time, the pyruvic acid is reduced to lactic acid. There is no blood circulation to remove the lactic acid, which therefore accumulates, and the muscle tissue acidifies. The pH drops from about 7.2 to 5.5 in a typical muscle. Acidification proceeds, and the generation of ATP continues, until the enzyme systems will no longer work in the acid conditions, or all the glycogen is used up. In beef an initial concentration of muscle glycogen of about 10 mg/g or more leads to normal acidification. The rate of acidification varies between species. It is most rapid in pork, followed by lamb then beef. The pH in beef may continue falling for 36 to 48 hours, while in pigs ultimate pH values are usually reached within 4 to 8 hours. The acidification leads to denaturation of some of the muscle proteins, and changes the characteristics and appearance of the muscle. It becomes paler and more opaque, and its ability to bind water decreases. If the muscle is cut the surface will exude moisture. This produces the drip (known in North America as purge) seen in retail packs of meat. The pattern of acidification can have a large effect on meat quality. In extreme cases a very rapid acidification, caused by stress immediately before and at slaughter in pigs, can result in PSE (pale, soft, exudative) pork; a limited extent of acidification, caused by longer-term stress, that depletes muscle glycogen, can result in DFD (dark, firm, dry) meat in all species (often known as DCB – dark cutting beef – in cattle). Lesser variations in the pattern of acidification are the cause of much of the variation seen in the colour, particularly paleness or darkness, and in water-holding capacity in normal meat. After slaughter, the muscles also gradually stiffen, signifying the onset of rigor mortis. The time of onset varies between species and between individual animals. ATP present in living muscle keeps it in a relaxed state, as well as being needed for contraction. When ATP can no longer be generated by glycolysis and is eventually exhausted, the muscles lose this relaxed state and pass into rigor. Their length becomes fixed because the actin and myosin molecules bind together irrevocably so the thick and thin myofilaments will no longer slide over one another. The onset of rigor is therefore controlled solely by the availability, or not, of ATP. It is not necessarily related to muscle pH, although normally the muscle will enter rigor as it acidifies. Factors that affect the level of muscle glycogen around the time of slaughter can also affect the rate of rigor development because lower than normal levels of glycogen provide only a limited supply of potential ATP. So, animals that have been very stressed, or exhaustively exercised, before slaughter may go into rigor more quickly than normal. If all the glycogen is depleted before slaughter the muscle will not acidify at all and rigor will occur very rapidly in this relatively alkaline state. This is therefore known as alkaline rigor. The thick myosin filaments and thin actin filaments are organized in the muscle in functional units called sarcomeres. Each myofibril within the muscle cell is composed of thousands of sarcomeres arranged end-to-end. The sarcomeres are shorter in contracted muscles and longer in relaxed muscles. Sarcomere length is a major factor influencing meat toughness. If contracted muscles are cooked they tend to be tough, while relaxed muscles tend to be tender. Rigor is important because it fixes the lengths of the sarcomeres, and therefore the muscle’s length and the potential texture of the meat. Muscles entering rigor in a very contracted state will tend to produce tough meat; those entering rigor in a relaxed, or stretched, state will produce tender meat. This is taken advantage of in certain novel methods of hanging carcasses just after dressing has been completed. In these, by supporting the weight of the carcass from the pelvic girdle, rather than from the more usual hind leg, certain muscles are stretched before they go into rigor, so that the sarcomeres are extended. The longer sarcomere length is ‘fixed’ when rigor develops so that the resulting meat is more tender. Before the onset of rigor, stimuli that cause muscle contraction may result in changes in meat texture. One stimulus that is especially significant is a cold temperature. If the carcass is cooled too quickly then some of the muscles may contract and, if as is likely, the contraction is not followed by relaxation, the resulting meat is tough, a phenomenon known as cold shortening. It tends to be mostly a problem in lamb carcasses because these are small and so cool quickly. Sometimes it occurs in beef but rarely in pork. Rapid chilling is desirable in limiting bacterial growth and in reducing carcass weight loss. To prevent cold shortening carcasses are sometimes stimulated electrically soon after death of the animal. This speeds up muscle metabolism so that the muscles can no longer contract when subjected to any cold stimulus. Meat cooked immediately after the onset of rigor mortis is rather tough. With time the muscles soften and the meat becomes more tender when cooked. The rate of tenderization differs in the different species and increases with temperature. Chicken meat needs less than a day to achieve adequate tenderness but the texture of beef will improve with longer storage of up to 3 weeks or more. This variation leads to different recommended ageing times before meat is consumed: 4–10 days for pork, 7–14 days for lamb and 10–21 days for beef. Tenderization occurs through the action of proteolytic enzymes called calpains, that break down structural proteins in particular parts of the muscle cell and so weaken its structure. However, there is no dissociation of the actin–myosin of the myofilaments. In the live animal calpains are involved in the normal breakdown of proteins associated with the body’s protein turnover. Calpains are more active at higher pH values and are activated by calcium ions. These flood out of the muscle cell membrane systems when ATP is exhausted and energy for the membrane ion pumps is therefore no longer available, thus initiating proteolysis. For this reason, the infusion of meat with calcium salts (usually CaCl2) can promote tenderization, and this has been suggested as a way of tenderizing meat commercially. In the absence of calcium, calpains are inhibited by calpastatin, to which they are bound. Eventually, however, the calpastatin itself is broken down by the calpains. Longer ageing also improves the juiciness and flavour of the meat, as well as tenderness, but it is not clear how this occurs. Bendall, J.R. (1973) Post mortem changes in muscles. In: Bourne, G.H. (ed.) The Structure and Function of Muscle, 2nd edn, Volume II, Part 2. Academic Press, New York and London, pp. 243–309. Bendall, J.R. and Swatland, H.J. (1988) A review of the relationships of pH with physical aspects of pork quality. Meat Science 24, 85–126. Dransfield, E. (1994a) Optimisation of tenderisation, ageing and tenderness. Meat Science 36, 105–121. Dransfield, E. (1994b) Tenderness of meat, poultry and fish. In: Pearson, A.M. and Dutson, T.R. (eds) Quality Attributes and their Measurement in Meat, Poultry and Fish Products. Blackie Academic and Professional (Chapman and Hall), London, pp. 289–315. Fabiansson, S., Reutersward, A.L. and Libelius, R. (1985) Ultrastructural and biochemical changes in electrically stimulated dark cutting beef. Meat Science 12, 177–188. Farmer, L.J. (1992) Meat flavour. In: Johnston, D.E., Knight, M.K. and Ledward, D.A. (eds) The Chemistry of Meat-based Foods. Royal Society of Chemistry, Cambridge, UK, pp. 169–182. Warriss, P.D. and Brown, S.N. (1987) The relationship between initial pH, reflectance and exudation in pig muscle. Meat Science 20, 65–74. Fresh meat is a general term commonly used for chilled skeletal muscles with incorporated tissues (e.g. fatty tissue, connective tissue, lymph nodes, blood/lymph vessels, nerves), in which the main post-mortal changes have been completed but which have not been subjected to treatments such as freezing, salting/curing, drying and similar. Basic characteristics of meat include colour, water-holding capacity, aroma and texture. Muscle pigment is myoglobin, a chromoprotein which – together with other coloured compounds such as haemoglobin – gives meat its normal red colour. Myoglobin comprises a porphyrin ring with an iron atom in the centre (haem) and an albumin-type protein globin. The iron in myoglobin is in the Fe++ form, but during oxidation changes to Fe+++. The total myoglobin content, and hence intensity of red colour, in animal muscles varies with species (e.g. horse>cattle>pig), age (older>younger), sex (male>female) and diet. At slaughter, myoglobin is normally saturated with oxygen (oxy-Mb, Fe++, having a pink–red colour), but after slaughter oxygen is spent and meat predominantly contains myoglobin (Mb, Fe++, having a purple colour). Depending on conditions, e.g. partial oxygen pressure, pH and storage, met-myoglobin – in which iron is oxidized (met-Mb, Fe+++, having a brown colour) – can be formed. Therefore, meat colour depends on relationships between, and proportions of, the three forms of the main meat pigment. Higher levels of oxygen (e.g. >20%) lead to formation of oxy-Mb, whilst met-Mb is formed at lower (e.g. <4%) levels of oxygen. Lower meat pH and higher storage temperature usually contribute to met-Mb formation. Meat has the ability to hold (bind) naturally contained or added water when exposed to some treatments such as heating, freezing or pressure. The proportion of water that is separated/released from meat after such treatments is called ‘free water’, whilst the proportion that remains within meat is called ‘bound’ water. The former comprises mainly water located extra-cellularly, and the latter comprises water firmly immobilized, i.e. chemically bound (electrostatically) to meat protein molecules. The amount of immobilized water in meat is variable. At higher pH, meat has a higher WHC than at lower pH, so the highest WHC is immediately after slaughter, whilst the lowest occurs during post-mortem rigor. During meat tenderization, the WHC increases. Meat aroma is a combination of sensations due to actions of hundreds of chemical compounds from meat on our taste and smell senses. The aroma-inducing meat compounds include sodium and potassium salts, lactic acid, ribose, nucleic acids, amines, glycogen and fatty acids. Meat aroma is affected by animal species, age, sex, diet and the extent of post-mortem meat ageing. A particular problem with meat aroma can be caused by the presence in meat of higher levels of compounds related to the male hormones androsterone, skatole and indole, which give meat (particularly fatty tissue) a sexual odour called ‘boar taint’. It can become a problem in meat from uncastrated (‘entire’) male pigs slaughtered older than 6–8 months and >80 kg live weight. If too strong, boar taint can make meat unfit for human consumption, but individual tolerance can vary with culture, eating habits and sex; women appear to be more sensitive than men. Sensorially detectable concentrations of androsterone in meat are > 0.2–0.5 mg/g. The physical properties of meat that can be registered visually, by touch and hearing, as well as during mastication, are called meat texture. Meat structure comprising relationship and connection between different tissues can be sensed visually. Tenderness/toughness of meat can be sensed by touch and also during mastication. Sound produced during chewing of meat also can be sensed aurally. Meat texture is affected by animal species, age, sex, breed and condition; it is determined by muscle structure, characteristics of connective tissue, amount of intramuscular fatty tissue, and how all these are interconnected. In addition, texture is affected by the extent of post-mortem changes, i.e. ageing, intensity of rigor mortis and WHC. Immediately after slaughter, meat texture is soft and elastic, but loses elasticity and becomes much firmer after a few hours. Subsequently, meat becomes more tender only after sufficient ageing. Meat having a very high WHC (e.g. DFD meat) has a firm and dry texture, whilst meat with a low WHC (e.g. PSE meat) is soft and with loose structure. Artificial meat refrigeration as a means to extend meat shelf life has been known for a long time; commercial use started in the late 19th century in the USA. Its effects are based on inhibition of multiplication and/or metabolic activity of contaminating microorganisms. Carcass refrigeration technologies differ, but can be divided into dry (i.e. air) refrigeration used for red meat and poultry carcasses, and wet (i.e. water) refrigeration used for poultry. Air refrigeration of red meat carcasses includes three main types: slow, rapid and ultra-rapid (i.e. shock) refrigeration. Slow air refrigeration is used rarely, in small abattoirs with traditional technologies, and comprises three steps: carcass ‘draining’ or ‘drying’ at ambient temperature lasting a few hours, pre-refrigeration (around 10°C; 75% relative air humidity – RH) and refrigeration (4–7°C, 85–90% RH). The resulting dry carcass surface is beneficial in terms of surface microflora supression, but the weight loss is relatively high (around 3%). In the case of rapid air refrigeration, carcasses are exposed to air at −1 to +1°C, 90% RH and 1–3 m/sec circulation for 24–36 h (cattle) or 18–24 h (pigs, lambs); weight loss is around 1.5–2.0%. Ultra-rapid air refrigeration is two-phased. Carcasses are first exposed to intensive circulation (2–4 m/sec) of very cold (−4 to −6°C) and humid (90–100%) air in special tunnels for around 1–3 hours. This is followed by refrigeration at −1 to 2°C for 18–22 h (cattle) or for 14–16 h (pigs) with circulation of only 0.1 – 0.3 m/s. Weight loss is around 1%. Whilst as rapid as possible refrigeration of carcasses is desirable from microbial safety and practical perspectives, too rapid cooling can lead to some meat quality problems. If muscle is cooled below 10°C before rigor is complete, i.e. before the pH drops below 6.0 and while glycogen and ATP are still present, meat becomes very tough (see also Chapter 7.1). This phenomenon is in practice called ‘cold shortening’. The following biochemical explanation for cold shortening has been offered. Contraction-stimulating calcium is stored in mitochondria and in the sarcoplasmic reticulum, and a ‘pump’ (ATP-powered) removes it from the cytoplasm. However, at cell temperatures below 10°C this pump is less efficient, which enables leakage of calcium from its stores. If ATP is still present at those low temperatures, i.e. if they are reached before rigor, the leaked calcium will stimulate shortening of the myofilaments, i.e. contraction. To prevent cold shortening, the refrigeration regime should be adjusted to ensure that meat temperature does not fall below 10°C in 10 h (for beef) or 7 h (for pork). Another way to prevent cold shortening is to ‘electrostimulate’ carcasses before refrigeration. The treatment is based on passing pulses of electrical current through muscles, which stimulates a large number muscle contractions in a short time so that glycogen and ATP are quickly used up. This accelerates rigor onset and enables rapid cooling without cold shortening. Electrical stimulation treatment can be either of the high-voltage type (e.g. peak 700–1000V; 14 Hz pulses frequency; 90 sec duration) or the low-voltage type (e.g. 90 V; 14 Hz; 60 sec). The former can be applied within 1 h post-slaughter, whilst the latter only immediately after slaughter. In addition, there are indications that electrical stimulation can improve meat tenderness, to a lesser extent, even in situations where cold shortening is unlikely, e.g. in slow-refrigerated meat. A potential third way of reducing meat toughness during chilling is by hip suspension of carcasses, which mechanically reduces shortening of the main muscles. The storage shelf life of chilled red meat carcasses can be very variable, depending on both storage conditions and levels of initial microflora. Under good conditions (e.g. 2°C), carcasses can have a shelf life of 3–4 (cattle) or 1–2 (pigs, lambs) weeks. However, under the same conditions, offal has a much shorter storage shelf life (e.g. 3 days). Wet refrigeration is used mainly for poultry and is based on either spraying poultry carcasses with cold water or on submerging them in basins (spin-chillers) with running cold water for around 1 h. The former system is hygienically beneficial from the perspective of preventing cross-contamination, but uses more water (up to 10–12 l/carcass) than the latter (up to 4 l/carcass). In modern times and in developed countries, most chilled meats are sold packaged. The main intentions with packaging of meat are to protect the product from secondary contamination during the handling–retail display–consumer chain of events, or to suppress microflora, or both. Microbial spoilage of meat can be caused by various organisms (Table 7.1), but the most important group is bacteria. Under conditions suitable for growth of both bacteria and fungi (yeast and mould) on a given food, bacteria always out-compete and outgrow fungi, and thus are the cause of spoilage. Normally, fungi cause food spoilage only where bacteria are suppressed, e.g. in very acidic or very dry foods. Table 7.1. The three main categories of microorganisms that can spoil meat. Packaging meat in oxygen-permeable materials has beneficial effects with respect to the stability of meat colour, as oxygen helps formation of redcoloured oxygenated forms of myoglobin. On the other hand, as oxygen is available on the product surface, after sufficiently long storage the meat will undergo an aerobic type of microbial spoilage. During aerobic spoilage, various meat compounds are degraded by meat enzymes (minor contribution) and microbial enzymes (major contribution), which can result in numerous end products having unpleasant sensory characteristics. Proteinaceous elements of meat are first degraded to lower molecule compounds in the order: proteins–polypeptides–peptides–free amino acids. Such changes occur normally during meat maturation (ageing) and, up to this stage, no negative effects on sensory qualities are noticeable. However, when the changes include degradation of free amino acids resulting in accumulation of compounds such as ammonia, hydrogen sulphide and amines, this produces characteristic, putrid off-odours (so-called putrefactive type of spoilage). In addition, protein degradation includes changes of myoglobin into oxidized forms, which results in a change of the red meat colour to grey, brown or green. On the other hand, if the meat contains more carbohydrates – natural or added – their degradation will result in accumulation of acids, which characterizes the so-called souring type of spoilage. Chemical changes during meat spoilage also include oxidation of fats, along the following chain of events: fats–free fatty acids–aldehydes/ketones. This results in rancidity. Aerobic spoilage of chilled meat can be caused by a range of psychrophilic or psychrotrophic microorganisms. Nevertheless, at <5°C, Pseudomonas is normally a dominant spoilage organism, particularly if the meat pH is lower (e.g. beef). At refrigeration temperatures >5°C, however, Brochothrix thermosphacta can become more dominant, particularly if the meat pH is higher (e.g. pork, lamb). Signs of spoilage become noticeable at microbial levels between 107/g (off-odour) and 108/g (slime). Nevertheless, the composition of microflora and the metabolic patterns of dominant microorganisms are more relevant for spoilage than are the microbial numbers. Also, it seems that microbial metabolites from amino acids appear in higher-pH meat sooner (i.e. at microbial level of 106/g) than in lower-pH meat (i.e. at level of 108/g). The unpleasant sensory characteristic of aerobically spoiled meat is a consequence of the accumulation of a complex mixture of microbial metabolites. The first stage of spoilage is characterized by a sweet, fruity odour (ethyl esters). In the advanced stage, a putrid odour is noticeable due to accumulation of sulphur compounds, ammonia and amines – particularly if Pseudomonas is dominant – and of acetoin, diacetyl and 3-methylbutanol, particularly if Brochothrix is dominant. Ultimately, signs of spoilage include meat greening (with or without fluorescence) and slime layer. The general course of spoilage in comminuted, aerobically stored meat is similar to that of meat cuts, but is faster. The reasons for this includes poorer microbial status due to usually higher initial microbial levels, distribution of surface meat contamination throughout the product during mincing, and to the higher growth rate of bacteria due to damaged tissue membranes, finer structure and larger surface area in minced meat. Dominant spoilage organisms include Pseudomonas and psychrotrophic Enterobacteriaceae, but if the former is suppressed (e.g. by certain added preservatives) spoilage can be caused by Brochothrix thermosphacta. Surface spoilage of aerobically stored joints is similar to spoilage of other raw meats, but spoilage can also develop in deep meat where anaerobic conditions exist even if the joint is stored aerobically. Deep meat spoilage (commonly called ‘bone taint’) is associated particularly with joints including cured – such as shoulder, gammon – and is characterized by off-odours in deep meat close to bones and/or in bone marrow. It occurs more frequently during summer and in higher-pH meat. The causes are not yet fully understood, but possible explanations include internal contamination (invasion) by bacteria during the agonal phase of pig slaughter and poor distribution of curing agents. Bone taint can take the course of either a souring- or a putrefaction-type of spoilage, and a range of bacteria – including particular types of psychrotrophic Clostridia – have been isolated from such meat. Generally, poultry meat is packaged only aerobically as its colour is more sensitive than that of red meats. Bacteria on poultry carcasses occur in feather holes and on cut surfaces, but their distribution on carcasses can vary considerably depending on the abattoir technology. Aerobic spoilage of chilled (<5°C) eviscerated poultry is very similar to that of red meat, but is usually faster. With this technology, gas-impermeable packaging materials – such as an aluminium-foil layer – are used. After removal of the air from the packaging by vacuum, the bag is filled with a sufficient volume of 100% CO2 gas and sealed. A proportion of carbon dioxide dissolves in water on the meat surface (‘meat saturation’) and produces carbonic acid, which lowers the surface pH of the meat. This, in combination with CO2-induced damage of bacterial cells’ membranes’ permeability and inhibition of bacterial enzymes, causes extension of the bacterial lag phase and generation times. Because of the lack of oxygen, the dominant microflora on saturated carbon dioxide-packaged meat include Lactobacillus, Leuconostoc and Carnobacterium (similar to vacuum packaging), producing lactic acid and having a more acceptable ‘cheesy’ odour. The net result is prevention of growth of aerobic spoilage flora, selection for lactic acid bacteria and extension of the meat shelf life. In addition, CO2 eliminates oxidative rancidity of fats. Therefore, meat packaged in saturated carbon dioxide can be cold-stored incomparably longer (several months) than aerobically packaged meat (1–2 weeks), and significantly longer than vacuum-packaged meat (several weeks). However, packaging of raw meat in saturated CO2 poses one meat quality-related problem: 100% CO2 causes a brown colour of the meat, which is unacceptable for consumers. However, when the meat is removed from such packaging and exposed to oxygen, its normal red colour recovers. Therefore, packaging in a saturated carbon dioxide atmosphere is used primarily for long-term bulk storage of raw meat, such as in the case of lengthy transport to remote markets, where it is subsequently removed and packed in oxygen-containing packaging for retail sale. On the other hand, cured meat products having a stable red colour (nitrites) can be, and often are, packaged and retailed in a CO2 atmosphere. Modified atmosphere meat packaging technology is aimed at simultaneously using the advantages, and avoiding the disadvantages, of oxygen and carbon dioxide. Modified atmospheres in the packages comprise a gaseous mixture of lower concentrations of oxygen (to maintain red colour) and higher concentrations of carbon dioxide (to inhibit microflora), but also can contain certain proportion of inert gas(es) to maintain targeted concentrations of the former two. Meat companies usually find the atmosphere composition that suits their own products best through trial and error. Nevertheless, generally, MAPs for raw meat comprise 60–75% CO2, 10–25% oxygen and 15–30% nitrogen. The type of dominant flora in MAP-packaged meat depends on the gas composition. If high levels of oxygen are used, meat spoilage will be similar to aerobically stored meat – putrefactive (Pseudomonas). If lower levels of oxygen, but higher levels of CO2, are used, the meat spoilage will be caused by lactic acid bacteria – similar to vacuum-packaged meat. This type of packaging is aimed at incorporation of certain indicators either of meat spoilage or of health hazards, or of both, that would easily and visibly warn when the meat is no longer acceptable. A number of various indicators have been researched: • storage time–temperature indicators; • leak indicators (e.g. for oxygen); • freshness indicators that detect microbial metabolites, e.g. diacetyl, amines, ammonia, ethanol, hydrogen sulphide; • indicators for activity of microbial enzymes; • indicators of consumption of certain nutrients by microflora; • food safety indicators based on detection of microbes as such or their toxins. Although ‘intelligent’ packaging of foods probably has a great potential, the technology is still largely in the research/development stage. With this technology, certain antimicrobial compounds – such as chemical preservatives or bacteriocins – are incorporated into, and subsequently are slowly released from, packaging material onto the food to suppress the microflora. Alternatively, such antimicrobials can be introduced (e.g. sprayed, injected) and contained within the package, i.e. between the packaging material and the food. Freezing of meat in industrial abattoirs is normally carried out in so-called ‘freezing tunnels’ with air at temperatures of between −20 and −40°C, a relative humidity of 95–100% and a circulation of 2–4 m/s. These rooms must have effective insulation from the outside environment, and walls/doors made of appropriate materials to withstand freezing. Due to associated occupational health and safety risks, appropriate security measures are necessary in these rooms, including an appropriate locking system and door heaters which preventing their freeze-blockage in the shut position. Meat freezes (cryoscopy points) at temperatures of between −1.5 and −1.8°C (muscle tissue) or –2.2°C (fatty tissue). Only the water in meat is actually frozen, and initially only a proportion of it. The concentration of various compounds dissolved in the water increases in the remaining unfrozen water which, in turn, decreases its freezing point. Consequently, approximately only 75% of water in meat is frozen at −5°C, around 90% at −30°C and 100% at −60°C. Various freezing regimes are used in the meat industry, with freezing rates determined by factors such as air temperature and circulation, as well as by size, shape and fat content of the meat. The meat freezing rate can be expressed by at what depth, measured from the surface, meat becomes frozen within a given time unit. For example, air-mediated (convection-based) freezing regimes can be slow (freezing rate <1 cm/h), rapid (1–5 cm/h) or very rapid (>5 cm/h). In the case of contact freezing where the meat is in direct contact with cold plates (conduction-based), even higher freezing rates are achieved. Freezing and defrostation have some profound effects on meat quality. Normal post-mortal biochemical processes are very inhibited. If the meat is frozen before the onset of rigor mortis, rigor will be delayed but will resume on defrostation – sometimes called ‘defrostation rigor’. The volume of meat increases with freezing due to ice formation. Ice crystals cause physical damage to muscle cell membranes; this is more so during slow freezing, where larger crystals are formed, than during rapid freezing. Partial, freezing-induced denaturation of meat proteins also occurs which, in combination with damage to membranes, reduces the water-holding ability of the meat after defrostion. Consequently, a certain amount of meat juice (drip) is released from defrosted meat, usually causing weight loss of around 1–1.5%. The amount of defrostation drip depends on various factors, including the defrosting method. If frozen meat is defrosted slowly by placing it at refrigertion temperatures (e.g. 4–7°C), the drip will be less than if quickly defrosted at higher temperatures. As a simplified, practical guidance for frozen storage, it could be said that the rapid freezing–slow thawing system best preserves the quality of raw meat. Microbial spoilage of meat during frozen storage is very inhibited or totally prevented. As indicated in Chapter 7.3, this inhibition is temperature-dependant. It can be assumed that bacterial activity ceases at temperatures of −7°C, whilst that of yeasts and moulds ceases at −12°C. Therefore, to prevent microbial spoilage, meat should be stored at −12°C (frozen meat) or preferably at −18°C or less (deep-frozen meat). However, that will not prevent physical and chemical changes in the frozen meat resulting in slow, undesirable changes in the sensory qualities of the meat that will make it ultimately unacceptable, i.e. unfit for human consumption. If the frozen meat is stored non-packaged, the ice on the meat surface can evaporate (sublimation) and the exposed meat can oxidize producing dry, discoloured patches – popularly called ‘freezing burns’. The main cause of chemical spoilage of frozen meat is oxidation of the fat, i.e. free fatty acids resulting in compounds (e.g. aldehydes, ketones) characteristic of rancidity. Fatty tissues with a higher content of unsaturated and polyunsaturated fatty acids (‘soft fat’) become rancid sooner, so they have a shorter frozen shelf life than fatty tissues containing mainly saturated fatty acids (‘hard fats’). As the saturation degree of fatty acids in meat of different animal species is in decreasing order: beef–lamb–pork–poultry–fish, their frozen shelf lives are in correlated decreasing order as well. They depend on pre-freezing freshness and composition of meat, storage temperature and the packaging material but, as a general idea, it could be presumed that frozen beef can be stored for up to 12 months, lamb 9 months, pork 6–7 months, poultry 4–5 months and oily fish 2–3 months. The terms ‘salting’ and ‘curing’ of meat are often erroneously used as synonyms, not only among consumers. In fact, meat salting means treatment of meat with salt (sodium chloride) only, whilst in meat curing, the product is treated with cure (also called brine) – a mixture containing salt, nitrites and/or nitrates and possibly some other compounds (e.g. phosphates, reducing agents, sugars). Salting and curing of meat are carried out in a similar manner, and the practical applications can be grouped into: (i) dry methods (mainly for dry meat products); (ii) wet methods (mainly for cooked products); and (iii) combined methods. WET METHODS. With wet methods, salting or curing can be conducted by submerging the meat in a solution of salt/brine in water for certain periods of time (with refrigeration), allowing the salt/brine ingredients to diffuse from the solution into the meat. The process can be speeded up by using machines with multiple, hollow needles to inject a more concentrated solution deeply into the meat, followed by mechanical treatments of the meat (in ‘massaging’ or ‘tumbling’ machines). This further enhances the diffusion and uniform distribution of the salt/brine. COMBINED METHODS. With combined methods, meat is first dry-salted/cured and then additionally treated by salt/cure solution. DRY SALT. Dry salt normally contains >95% sodium chloride. Its main role is to lower the water activity (aw) of meat so as to inhibit spoilage and pathogenic microorganisms, as well as to achieve desirable taste. Optimal concentrations of salt targeted in the meat depend on the type of the intended meat product, and range from 1.5–2.2% in different types of cooked sausages or 2.5–7% in dry meat products. DRY BRINE. Dry brine can comprise: (i) salt with 0.5–0.6% sodium nitrite; (ii) salt with 1–3% potassium nitrate; (iii) salt with 0.5–0.6% sodium nitrite and 1% potassium nitrate; or (iv) salt with 0.9–1.2% potassium nitrate. Dry brines are normally pre-mixed and are commercial products, rather than composed on the plant’s production line. Using commercial mixes avoids public health risks associated with either handling of concentrated toxic compounds (nitrites), their overdosing, or both, by the plant’s staff. SODIUM NITRITE. Sodium nitrite (NaNO2) is a reactive, unstable, toxic compund, so is added to meat in concentrations of less than 100–200 mg/kg (i.e. <0.01–0.02%). For potential public health concerns related to use of nitrites in meat see Chapter 1.3. The roles of nitrites in meat processing are multiple: (i) meat safety-orientated: inhibition of spoilage and pathogenic bacteria (particularly of Clostridia, including Cl. botulinum); and (ii) commercial/meat quality-orientated: stabilization of meat colour, inhibition of fatty acid oxidation and improvement of aroma. POTASSIUM NITRATE. Potassium nitrate (KNO3) is much less toxic than nitrites and is a very weak inhibitor of microorganisms. It contributes to the desirable flavour of meat products, but in higher concentrations is bitter, so is not added to meat at levels greater than 0.5–0.6%. The main role of nitrates is to serve, through reduction of NO3 to NO2, as a source (precursor) for the formation of nitrites. PHOSPHATES. Phosphates are chain molecules comprising two (diphosphates) or three (triphosphates) or several tens (polyphosphates) of phosphorus atoms linked with oxygen. Their role is to enhance both the water-holding capacity of meat proteins and the mixing of fat and water (emulsion). Therefore, phosphates are normally used for commercial/meat quality reasons in meat products that contain added water (e.g. hot dogs, cooked hams, etc.). There are numerous commercially available phosphate preparations that can be of a neutral, basic or acidic nature. Phosphates are added in concentrations of up to 0.3% (calculated as P2O3) in the finished product. REDUCING AGENTS. Reducing agents include primarily sodium salts of ascorbic acid or isoascorbic acid. They are added to meat in concentrations of up to 500 mg/kg (i.e. 0.05%), normally towards the end of the production process. Their role is to reduce meat redox potential so to enhance both reduction of nitrites to nitrogen monoxide and the formation of nitrosylmyoglobin (i.e. the red colour) in cured meat. SUGARS. Sugars, usually a mixture of dextrose, saccharose and starch, are added to some meat products with the aim of improving the taste/aroma, including masking saltiness or bitterness (from nitrates), to contribute to red colour formation, as a reducing agent, and to enhance the growth of useful microorganisms in some products such as fermented meats (e.g. salami). Smoke is a result of aerobic or anaerobic pyrolysis of woods, their polysaccharides: cellulose, hemi-cellulose and lignin. It starts at around 170°C, and at temperatures up to 270°C is endothermic, and above that has an intensive exothermic nature. Anaerobically, pyrolysis results in smoke containing a large number of chemical compounds such as organic acids, aldehydes, alcohols, furans and phenols, that are useful for meat production. At higher temperatures (>300–400°C), undesirable toxic polycyclic aromatic hydrocarbons (see Chapter 1.3) are formed, in increasing concentrations as the temperature increases. Aerobically, wood burns intensively, with smoke containing largely water vapour and carbon dioxide. Therefore, for food-smoking purposes, smoke is produced mainly endothermically, from ‘damp’ wood chippings and by limiting the air supply. Meat smoking can be based on traditional or modern technologies. In the former case (small plants), wood is pyrolysed in open containers placed inside or outside smoking rooms where products are hung; the conditions (and the smoke) are very difficult to control. In the latter case, smoke is produced in industrial generators, with wood either being spread on heated metal plates or being pressed against a fast-rotating plate (friction). With both type of generators, the conditions, and hence the smoke temperature (around 200°C) and composition, are controllable. The smoke generated can be treated in various ways to achieve desirable characteristics, e.g. be cooled or heated within a pipe system, or dissolved/condensed in water or other liquids to produce ‘liquid smoke’, and/or specially filtered to remove particles or unwanted compounds, before its application to the product. Applications include cold-smoking in the air (e.g. fermented sausage, dried meats), hot-smoking in the air (e.g. cooked sausage), electrostatically-aided smoking in the air, incorporation of liquid smoke into brine or product mixture and submerging/spraying of the product with liquid smoke. The two main reasons for smoking meats are: (i) commercial/meat quality-orientated, to achieve popular sensory qualities of the product; and (ii) meat safety-orientated, to inhibit spoilage and pathogenic microorganisms. Product quality effects of meat smoking include typical colour due both to attachment–polymerization of smoke compounds on the product surface and to reactions between smoke carbonyls and meat amines (Maillard reaction); typical aroma; and better texture, i.e. physical structure of the product due to coagulation of meat proteins (e.g. collagen). Product safety effects of smoking include inhibition of microorganisms on product surface by a number of chemical compounds present in smoke (see above). However, the antimicrobial effects of smoke are relatively limited and, alone, are insufficient to control bacterial pathogens or toxigenic moulds. Nevertheless, smoking can contribute to the antimicrobial effects of other preservative factors acting in/on the product. Drying of meat is based on removal of the water, through two simultaneous processes: internal and external diffusion of moisture. Through the former, moisture migrates from the inner layers of the product towards the product surface, whilst through the latter it evaporates from the surface. Drying can occur only if the partial water vapour pressure on the product surface is greater than the relative air humidity at a given temperature. During meat drying, loosely bound moisture diffuses first – initially only water located between myofibrils, but later also from within the muscle cells. A proportion of water firmly bound to the meat proteins can be removed under special conditions, e.g. under vacuum, but a proportion of water very firmly bound (hydrated water) cannot be removed even under vacuum. The net result of drying is lowering of water activity (aw) in the product to values inhibitory for spoilage and pathogenic microorganisms (see Chapter 7.3). However, excessive drying to very low aw values can result in a product with unacceptable sensory qualities. Meat drying is most often carried out by keeping the product suspended in air. Initially, whilst the product’s aw is relatively high and non-inhibitory for pathogens, drying is carried at lower temperatures, e.g. 10–15°C. As drying progresses and the product’s aw decreases, air temperature can be increased. At higher temperatures relative air humidity is lower, which speeds up drying. Nevertheless, the drying process should be gradual because, if it is too rapid, a hard, very dry layer of coagulated proteins could be formed on the product’s surface, which would prevent further drying of deeper layers. Generally, to achieve balanced meat drying, the relative air humidity should be 2–3 units lower than the aw value of the product. Another, less common drying method is lyophilization, in which the meat is first frozen and then exposed to a very low pressure of around 5–6 mbar (‘in vacuum’) at 20–40°C. In this way, even firmly bound water is removed (90% of the total) from meat, resulting in a very dry product – retaining the same volume but having only around 70% of the original weight. Lean meat is selected for lyophilization, to avoid the rapid rancidity to which it is prone, and later the product is used either for further processing into various industrially prepared dishes (e.g. soups) or for strategic reserves. Based on their final aw values, foods can be divided into three global groups: 1. High-moisture products having an aw value of 0.9–1.0 (e.g. cooked sausage, cooked ham); those with aw ≥0.95 and pH ≥5.2 have to be refrigerated at ≤5°C, those with aw 0.91–0.95 and pH ≤5.2 have to be refrigerated ≤10°C, and those with aw 0.9–0.95 and pH ≤5.0 can be stored without refrigeration. 2. Intermediate-moisture products having an aw value of 0.6–0.9 (e.g. dried ham, salami), that can be stored without refrigeration. 3. Low-moisture products having an aw value of <0.6 (e.g. lyophilized meat) that are self-stable. As indicated in previous chapters, generally it is considered that the lowest aw value for growth of pathogenic bacteria is 0.88–0.9, but moulds can grow at an even lower aw. Meat can be heat treated either for product quality- or for product safety-orientated purposes, but in most cases the two are combined. Heating has multiple and complex effects on meat. Heat induces change in meat pigments typical for raw meat (myoglobin and oxymyoglobin, with Fe++, to metmyoglobin, with Fe+++), resulting in a change of meat colour from red (raw) to brown–grey (cooked), e.g. in well-cooked steak. Only if the meat was cured (nitrites) before cooking, will the product still have a red colour post-cooking, due to the presence of nitrosyl-myoglobin, e.g. in cooked ham. On the other hand, heating denatures proteins and decreases their water-holding capacity. As a result, during cooking of untreated meat a significant proportion of water is released, with weight loss – depending on species, composition and pH of meat – of up to 40%. However, when meat has been treated with water-retaining additives (e.g. phosphates), the cooking weight loss is much reduced or prevented. Heat-induced changes in proteins also cause reduction of both length and diameter of myofibrils, resulting in a firmer texture of the cooked meat. Heating meat also contributes to the formation of a large number of compounds and changes in both protein and lipids, resulting in taste, smell and aroma typical of cooked meat that is also meat species-specific. Compounds that particularly contribute to these sensory characteristics include products of the Maillard reaction (occurring between reduced sugars and amino-compounds), aliphatic (aldehydes, ketones, amines, carboxyl acids, etc.) and aromatic (lactones, furans, thiazoles, pyridines, pyroles, etc.) carbohydrates. At pasteurization temperatures (e.g. cooking in water at 60–95°C) mainly aliphatic, whilst at high temperatures (e.g. frying, roasting, barbecuing at 150–190°C) mainly aromatic compounds are formed. Heat treatments generally reduce the nutritional (biological) value of meat, but the reduction degree depends on the cooking temperature. This is primarily due to heat inactivation of vitamins (e.g. riboflavin by 80%, niacin by 75%) and of some amino acids such as methionine, cysteine, etc. With respect to practical application of heat treatments of meat, they can be divided into: 1. Pasteurization in water or steam at temperatures <100°C as applied, for example, to various sausages (e.g. 70–80°C), canned hams (e.g. 62–68°C) and hot-smoked meats; this normally destroys all vegetative cells of psychrophilic and mesophilic microorganisms, but some vegetative cells of thermophilic bacteria – as well as all spores – can survive. Pasteurized products are stored with refrigeration. 2. Boiling in water at 100°C, with the temperature at the product’s centre reaching 80–90°C, e.g. with liver paté, black pudding, sausage, etc.; this kills all vegetative forms of microorganisms, but not spores. The products need refrigeration. 3. Commercial ‘sterilization’ at >100°C, usually in pressurized steam autoclaves, e.g. with canned meats sealed in a metal container with a non corrosive surface and treated at 105–130°C; this kills all vegetative forms; spores are either destroyed or injured to such an extent that they are rendered unable to germinate in the product. Sterilized cans, such as so-called botulinum-treated (e.g. 121°C for 20 min in the centre), can be stored for years with no refrigeration. Food safety aspects of heat treatment relate to efficacy in killing of pathogenic microorganisms and inactivation of meat enzymes, e.g lipolytic enzymes contributing to fat rancidity. For details of the nature, dynamics and quantitive parameters of the effects of heat on microorganisms see Chapter 7.3. It should be kept in mind that antimicrobial effects of heating meat depend on a number of variable factors and their inter-relations, including: (i) temperature and duration of heating; (ii) product substrate characteristcs such as lean–fat ratio, pH, aw and nitrites; and (iii) microflora characteristics such as species, type, growth phase and initial counts. In practice, substrate pH is a very relevant factor and, as a general guidance, it could be presumed that efficient killing (elimination) of pathogenic bacteria can be achieved in low-acid foods (pH >4.5, e.g. in meats) only by sterilization, in acid foods (pH 4–4.5) by boiling, and in very acid foods (pH <4.5) by pasteurization. Fermentation of meat is used only in the production of raw (uncooked) meat products, primarily fermented sausage. Fermentation can be defined as a phase of intensive growth and metabolism of lactic acid bacteria accompanied by production of lactic acid, causing a rapid fall in pH, which acts as a preservative. Natural fermentation occurs when indigenous lactic acid bacteria in the raw materials proliferate and anaerobically metabolize sugars, producing acid. The fermentation takes place normally over 2–5 days, during which Gram-positive facultative anaerobes and micro-aerophiles (Micrococcus, Staphylococus, Lactobacillus – the lactic acid bacterium) rapidly increase in numbers and lower the pH, until it is typically 4.6–5 at the end of fermentation. The microorganisms which initially dominate chilled meat (the Gram-negative aerobes, e.g. Pseudomonas) become reduced in numbers and pathogens are suppressed. To speed up fermentation and suppress undesirable bacteria/pathogens as soon as possible, selected lactic acid bacteria (called ‘starter cultures’) can be artificially added. In addition to contributing to suppression of pathogens via low pH, some starter cultures also produce antimicrobial metabolites such as bacteriocins – sometimes called ‘protective cultures’. Bacteriocins are low-molecular weight proteins with antibacterial activity similar to, while not being, antibiotics. They are effective primarily against Gram-positive bacteria (e.g. Listeria monocytogenes, Staphylococcus aureus). Some purified bacteriocins are commercially available (e.g. nisin) and are used in dairy and bakery products. Starter/protective cultures have antimicrobial potential only when the product contains low numbers of indigenous bacteria, i.e. is produced under good hygienic practice. The effectiveness of protective cultures, when acting alone, is insufficient to guarantee food safety; rather, their use provides an additional safety factor working in parallel with other factors (e.g. low pH, low aw, nitrites) within the hurdle concept. Numerous factors affect the competitiveness of starter cultures during meat fermentation, since fermentation is a sophisticated and complex food preservation process. In practice, the suitability of starter or protective cultures can be assessed according to critical criteria and desirable criteria, described below. Critical criteria: 1. Effectively compete against indigenous bacteria. 2. Produce adequate quantities of lactic acid. 3. Tolerate at least 6% NaCl and 100 mg/kg NaNO2. 4. Grow in the temperature range 15–40°C. 5. Homofermentative – do not produce gas or undesirable metabolites. 6. Non-proteolytic, thus avoiding production of free amino acids, resulting in the lowest possible biogenic amine levels. 7. Do not produce biogenic amines (moulds or yeasts must not produce mycotoxins or aflatoxins). 8. Do not produce large quantities of peroxides (H2O2). Peroxides oxidize fats and produce undesirable rancid flavours and odours; peroxides also produce free radicals, which are undesirable in modern diets. In addition, products ultimately resulting from rancidity may cause liver damage in consumers. Desirable criteria: 1. Catalase positive, to ensure that any peroxide produced is largely destroyed. 2. Nitrate reducing. 3. Flavour enhancing. 4. Do not produce slime. 5. Antagonistic to pathogenic and undesirable organisms. 6. Synergistic with other components in the raw materials and starter. 7. Produce MAO or DAO. Finally, although starter organisms have the potential to be genetically modified to reduce undesirable and enhance desirable characteristics, at the present time, this approach would not be supported by consumers. Bauer, F. and Burt, S.A. (1995) Shelf Life of Meat and Meat Products: Quality Aspects, Chemistry, Microbiology, Technology. ECCEAMST Foundation, Utrecht, The Netherlands. Davies, A. and Board, R. (1998) The Microbiology of Meat and Poultry. Blackie Academic, London. Varnam, A.H. and Sutherland, J.P. (1995) Meat and Meat Products. Chapman and Hall, London. Bacteria, moulds, yeasts, parasites and viruses can all be detected in foods. Parasites and viruses do not proliferate in foods, but may survive. In contrast, bacteria, moulds or yeasts can grow in foods under permissive conditions. Limiting microbial growth in food can: • extend food shelf life; • reduce the risk of food-borne bacterial pathogens proliferating to infectious dose levels; and • reduce the risk of toxin production at toxic levels. Techniques and methods used in food harvesting, processing and packaging can limit or control microbial contamination of food. Control of contamination, both pre- and post-harvest, is a pre-requisite for food safety and hygiene, but is not discussed further in this chapter. A wide variety of foods that are a direct result of microbial growth are produced throughout the world. Safe production of such foods is dependent in part or wholly on correct microbial proliferation and on production of suitable metabolic products during processing. Foods produced and traded in large volumes which are traditionally produced as a direct result of microbial growth include fermented meat (sausage), fermented fish, fermented dairy products (hard cheese, yoghurt, sour cream, kefir, koumiss, cultured butter), pickled vegetables (gherkins, olives, sauerkraut), sourdough breads, soy sauce, shrimp paste, fish sauce, vinegar and alcoholic drinks (wine, spirits). Although these foods are all traditionally produced by the growth of microorganisms in the product, some of them can be produced using chemical and/or enzymatic methods. Four phases of bacterial growth are recognized during in vitro growth that are relevant to bacterial growth in foods (Fig. 7.1). The lag phase is the time taken for the population to adjust to the new environment, produce enzymes to exploit it, and to repair any damage resulting from previous injury (e.g. freezing, desiccation, heating), and bacterial numbers remain constant. Bacteria reproduce after chromosome replication, doubling in cell size, with subsequent division of the cell in two; the time taken for this to occur is the generation time. During the exponential growth phase (logarithmic phase), bacterial numbers increase exponentially. The generation time (doubling time) is the time taken for the cell number to double. The maximum specific growth rate (μmax) occurs during the exponential phase, and is equal to the slope of the tangent of the exponential part of the bacterial growth curve (Fig. 7.1). During the stationary phase, the number of cells dividing becomes equal to the number dying, as key nutrients become depleted or toxic metabolites accumulate. Finally, bacterial cultures enter the death phase. In vitro, bacterial numbers decrease as cells die from factors including starvation, toxin accumulation and inability to maintain homeostasis.
7.1 Conversion of Muscle to Meat
Introduction
Muscle Structure
Muscle Function
Post Mortem Changes in the Muscles
Meat quality problems associated with abnormal patterns of acidification
Development of rigor mortis
The importance of rigor for meat quality
The resolution of rigor mortis
Conditioning
Further Reading
7.2. Basic Methods Used in Food Preservation and Processing
Basic Characteristics of Meat
Meat colour
Water-holding capacity (WHC) of meat
Meat aroma
Meat texture
Meat Refrigeration
Packaging of Chilled Meat and its Spoilage
Aerobic packaging of raw meat and its spoilage
Chemical aspects
Microbial aspects
Sensory aspects
Comminuted meats
Bone taint
Poultry
Vacuum-packaging of raw meat and its spoilage
Packaging of raw meat in saturated (100%) carbon dioxide atmosphere and its spoilage
Packaging of raw meat in a modified atmosphere (MAP) and its spoilage
‘Intelligent’ packaging of food
‘Active’ packaging of food
Freezing of meat
Salting and curing of meat
Methods for salting or curing
Salting or curing preparations and roles of the ingredients
Smoking of meat
Drying of meat
Heat treatment of meat
Fermentation of meat
Further Reading
7.3 Basics of Food Microbiology
Introduction
Microorganisms in Food Production
Bacterial Growth
Stay updated, free articles. Join our Telegram channel
Full access? Get Clinical Tree
Meat Preservation and Processing
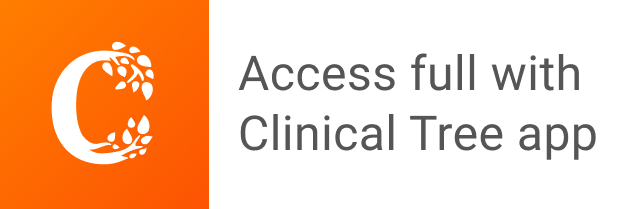