Eva Rioja Garcia Optivet Referrals, Havant, Hampshire, UK Local anesthetics reversibly block the generation and propagation of electrical impulses in nerves, thereby causing sensory and motor blockade. Their use dates from the late 1880s when cocaine was first used for ophthalmologic procedures by Carl Köller and Sigmund Freud. However, cocaine was found to be highly toxic and addictive and, since then, new agents with better pharmacologic profiles and less potential for systemic toxicity have been developed. Today, local anesthetics are the mainstay analgesic drugs used for local and regional anesthetic techniques in the perioperative period. These techniques cause complete desensitization of a localized area of the body, allowing a reduction in general anesthetic requirements and promoting greater cardiorespiratory stability. Sustained analgesia when a long‐acting local anesthetic is used is also beneficial in the recovery period. Apart from these advantages, there is new evidence in veterinary anesthesia that locoregional techniques, when used as part of a balanced anesthetic protocol, may reduce the risk of perianesthetic mortality [1]. There is also moderate‐quality evidence in humans that regional anesthesia may reduce the risk of developing persistent postoperative pain for up to 18 months in some type of surgeries [2]. In recent years, the surge in interest in locoregional anesthesia in veterinary medicine has meant the introduction of numerous new techniques and increased the use of local anesthetics worldwide. In addition to locoregional applications related to nerve impulse blockade, the local anesthetic lidocaine may also be administered systemically for a variety of indications, including management of ventricular arrhythmias, improvement of intestinal motility following ischemia‐reperfusion‐injury, reduction in general anesthetic requirements, and systemic analgesia. Local anesthetics are considered primarily ion channel blockers, acting mainly on voltage‐gated Na+ channels. However, they also block voltage‐dependent K+ and Ca2+ channels, though with lower affinity [3–7]. Some studies also suggest that local anesthetics act on intracellular sites involved in the signal transduction of G‐protein‐coupled receptors [8]. This range of molecular targets may explain some of the adverse and toxic effects that this group of drugs produces on various organ systems. Ten different genes encode voltage‐gated Na+ channels and they are differentially expressed throughout the major excitable tissues. For example, sensory neurons express mainly NaV1.7 and NaV1.8 Na+ channels, whereas cardiac cells, breast, and colon cancer metastatic cells express NaV1.5 [9]. While all of the current clinically available local anesthetics are nonselective, it is possible that in the future there will be subtype‐selective Na+ channel blockers [9]. The most important mechanism of action leading to local anesthesia involves the blockade of inward Na+ currents through voltage‐gated Na+ channels, thereby impeding membrane depolarization and nerve excitation and conduction [10]. The Na+ channel is a multimolecular complex with a large α‐subunit composed of almost 2000 amino acids that traverses the cell membrane several times. This subunit forms the channel’s pore and gating apparatus [10]. Some smaller auxiliary β‐subunits influence the activation–inactivation states of the channel [11]. The α‐subunit consists of four domains (I to IV) each containing six helical segments (S1 to S6) that wrap around and form a bell‐shaped central channel. Segments S5 and S6, and the P loop between them, form the channel or pore. The S4 segments in each domain have positively charged arginine or lysine molecules and form the voltage‐sensing module. The inactivation gate is formed by a short intracellular loop between domains III and IV (Fig. 29.1) [9,12]. The binding site for local anesthetic, antiarrhythmic and anticonvulsant drugs, is formed by amino acid residues in the S6 segments of domains I, II, and IV, which contribute to a single drug‐binding site within the pore [12]. Because this binding site is localized within the pore, it is only accessible when the Na+ channel is open [13]. Figure 29.1 Structure of the voltage‐gated Na+ channel. I through IV represent distinct domains of the α‐subunit. S1 through S6 represent helical segments within each domain that wrap around and form a bell‐shaped central channel. Segments S5 and S6, and the P loop between them, form the channel or pore. The S4 segments in each domain have positively charged arginine or lysine molecules and form the voltage‐sensing module. The inactivation gate is formed by a short intracellular loop between domains III and IV. Source: Taylor and McLeod [9], with permission of Elsevier. The channel is a gated conduit for Na+ ions and exists in three different gating states: resting (closed), open, and inactivated (Fig. 29.2), depending on the membrane potential and timing. At resting membrane potential (–70 mV), the S4 segments are in the “down” position, making the channel non‐conductive. During depolarization, the channel opens by outward spiral rotation of the S4 segments allowing rapid influx of Na+ ions down the electrical and chemical gradients. This conformational change exposes the receptor site of the inactivation gate, located between domains III and IV, which leads to channel inactivation after only a few milliseconds. Only by repolarization of the cell membrane does the channel recover to the resting state from the inactivated state [11,14]. There is evidence that local anesthetics may interact with the lipid membrane, altering its fluidity, causing membrane expansion, and thus reducing Na+ conductance [15]; however, this does not completely explain the mechanism of action of modern clinically used local anesthetics. The “modulated‐receptor hypothesis” proposes that local anesthetics have high binding affinity for the Na+ channel in its open and inactivated states, but low affinity for the resting state [10,16]. Lipid‐soluble drug forms are thought to enter and leave the receptor via a hydrophobic region of the membrane, while charged less lipid‐soluble forms pass via a hydrophilic region (the inner channel pore) [16]. The hydrophilic pathway is open only when the gates of the channel are open, which causes cumulative binding of local anesthetics to the Na+ channel when the channels are active. The “guarded‐receptor hypothesis” proposes that the receptor for local anesthetics is located inside the channel and that the drug binds to this receptor with constant affinity [17]. Access to the receptor is regulated by the channel gates and therefore the channel needs to be open for the receptor to be accessible to the local anesthetic. Increasing the frequency of stimulation increases the number of Na+ channels in the open and inactive states, which increases the binding of local anesthetics. Figure 29.2 The Na+ channel has two gates: an activation (“voltage sensor”) and an inactivation gate. At resting membrane potential, the channel exists in its resting (closed) state. Depolarization of the membrane causes outward rotation of the voltage‐sensing module causing opening of the channel, allowing Na+ ions to flow intracellularly. Within 1–2 ms, the inactivation gate closes automatically (inactivated channel), allowing repolarization to occur. Repolarization leads to conformational changes with closure of the activation gate and opening of the inactivation gate within 2–5 ms (i.e., the refractory period). Following repolarization, the channel is in a resting state again. Table 29.1 Classification of nerve fibers by Erlanger and Gasser and order of blockade. Source: Adapted from Khurana et al. [28]. Both of these hypotheses explain the property of tertiary amine local anesthetics whereby the depth of the block increases with repetitive membrane depolarization, which has been termed “use‐dependent block” or “phasic block” [18,19]. On the other hand, blockade obtained on unstimulated nerves is constant, which is termed “tonic block” [18,19]. Local anesthetics show a differential pattern of sensory and motor blockade that can be observed clinically when applied to peripheral nerves and the central neuraxis [20,21]. Vasodilation occurs first, followed by loss of sensation of temperature, sharp pain, light touch, and finally loss of motor activity (Table 29.1) [22]. This property is called “differential block” and was first described by Gasser and Erlanger in 1929 when they observed that, within myelinated A fibers, cocaine reduced compound action potentials from slower and smaller fibers more rapidly than from faster and larger fibers [23]. However, this differential block in vivo cannot be simply explained by the size of the fiber, but rather is influenced by numerous factors, including type of fiber (size and myelination), frequency of stimulation, length of nerve exposed to the local anesthetic, and choice and concentration of local anesthetic drug. Initially, it was hypothesized that the differential block produced by local anesthetics when applied to peripheral nerves was due to a greater susceptibility of small, unmyelinated C fibers compared to larger, myelinated A fibers. However, in vitro [24] and in vivo studies [18,25] have shown that A‐fiber susceptibility to phasic and tonic block is actually greater than that of C fibers, with the order of blockade from fastest to slowest being Aγ > Aδ = Aα > Aβ > C. This would suggest that motor and proprioceptive deficits should occur prior to loss of nociception, but this is opposite to what is clinically observed. Anatomic features such as myelination may also account for some differences in susceptibility, since myelin can effectively pool anesthetic molecules close to the axon membrane [26]. Experimental studies have found that unmyelinated fibers are less sensitive to lidocaine than myelinated fibers [27]. This is contrary to clinical observations of differential block, which is manifested by the loss of small fiber‐mediated sensation (e.g., temperature) two or more dermatomes beyond the sensory limit for large fiber‐mediated sensations. Exposure length of the nerve to the local anesthetic may in part explain differential block in vivo, as smaller fibers need a shorter length exposed than larger fibers for block to occur [29]. This has been called the “critical length” to completely block conduction which, in myelinated fibers, corresponds to three or more nodes of Ranvier [30]. Therefore, larger fibers with greater internodal distances are less susceptible to local anesthetic blockade. The somatotopic fascicular organization of peripheral nerve fibers may also influence the order of blockade. Early investigations of the brachial plexus in humans observed that nerve fibers in the mantle or peripheral bundles innervate primarily motor fibers of the proximal limb, whereas fibers localized more internally within the nerve innervate the distal limb, with sensory fibers being localized in the center bundles [31,32]. This could partially explain why in certain nerve trunks such as the brachial plexus, onset of anesthesia proceeds from proximal to distal as the local anesthetic penetrates the nerve down a concentration gradient. This is especially true when high concentrations of local anesthetics are used [32]. The recovery from the block may not follow the same pattern due to the intrinsic vascularization of nerves, which may contribute to the observed recovery of distal portions of the limb occurring before more proximal areas [32]. However, this somatotopic distribution of nerve fibers within nerves may not be consistent in different nerves or across species. What seems to be clear is that there is a high degree of somatic organization within mammalian nerves: each fascicle contains motor or sensory fibers innervating very specific areas of skin or specific muscles (Fig. 29.3) [33]. This distribution of fibers may also explain the partial blockade that is sometimes observed clinically. Another important mechanism of local anesthetic blockade is the phenomenon of decremental conduction, which describes the diminished ability of successive nodes of Ranvier to propagate the impulse in the presence of a local anesthetic [35]. This principle explains why the propagation of an impulse can be stopped even if none of the nodes has been rendered completely inexcitable [30], as occurs, for example, with low concentrations of local anesthetics. Concentrations of local anesthetic that block 74–84% of the sodium conductance at successive nodes cause a progressive decrease in amplitude of the impulse, until it eventually decays below the threshold [30]. Higher concentrations that block more than 84% of the sodium conductance at three consecutive nodes will prevent impulse propagation completely [30]. This explains why blocks of greater extent and duration result from injection of small‐volume/high‐concentration solutions versus large‐volume/low‐concentration solutions, despite the same total drug dose [36]. Figure 29.3 Camera lucida drawings of the median nerve from a Macaca mulatta monkey in which the nerve fibers of an individual digital nerve (in this case, the radial digital nerve of the thumb) were exposed to horseradish peroxidase. Sections from the nerve from the carpal tunnel to proximal arm show that these nerve fibers mainly remain closely packed together (black, dense labeling), but there is some scattering of a few of the nerve fibers (stippled areas, sparse labeling). Source: Brushart [34]. Some authors suggest that a large portion of the sensory information transmitted by peripheral nerves is carried via coding of electrical signals in after‐potentials and after‐oscillations [37]. Sub‐blocking concentrations of local anesthetics can suppress these intrinsic oscillatory after‐effects of impulse discharge without significantly affecting action potential conduction [38]. Thus, another possible mechanism of blockade of nerve function, especially at low concentrations of local anesthetics, is by disruption of coding of electrical information [19]. When local anesthetics are administered in the central neuraxis (epidurally or intrathecally) or systemically, they may possess other mechanisms of analgesic action at the level of the spinal cord in addition to those previously discussed. Local anesthetics inhibit other ion channels such as K+ or Ca2+ channels at the level of the dorsal horn of the spinal cord. This may affect central neuroprocessing of sensory information, thereby contributing to their antinociceptive effects [39–41]. In addition to ion channels, nociceptive transmission is mediated by several neurotransmitters in the dorsal horn, such as the tachykinins (e.g., substance P). Local anesthetics have been shown to inhibit substance P binding and evoked increases in intracellular Ca2+ [42]. Additionally, local anesthetics also inhibit glutamatergic transmission in spinal dorsal horn neurons, reducing N‐methyl‐D‐aspartate (NMDA)‐ and neurokinin‐mediated postsynaptic depolarizations [43,44]. Clinically useful local anesthetic drugs are composed of a lipophilic, benzene ring with different substitutions (aromatic ring), and a hydrophilic amine group (tertiary or quaternary amine), which are linked through an intermediate chain, either an ester or an amide. Depending on the type of link, local anesthetics are classified as (1) aminoesters which are hydrolyzed by plasma cholinesterase (also known as “butyrylcholinesterase” or “pseudocholinesterase”), or (2) aminoamides which are metabolized by the liver. The physicochemical properties influencing local anesthetic activity include molecular weight, pKa, lipid solubility, and degree of protein binding (Table 29.2) [45,46]. The molecular weight of clinically used local anesthetics is very similar, ranging between 220 and 288 Da. The diffusion coefficient is thus not significantly affected and molecular weight does not seem to be an important factor determining differences in activity of local anesthetics [45]. However, changes in molecular weight due to alkyl substitutions may influence other properties such as lipid solubility and pKa. Table 29.2 Physicochemical properties and relative potencies of clinically used local anesthetics. Source: Data obtained from Liu [46]; Strichartz et al. [48]. ND, no data. a Measured with spectrophotometric method at 36 °C, except prilocaine and ropivacaine measured at 25 °C. b Partition coefficients expressed as relative concentrations (mol/L) in octanol and buffer at 36 °C, except prilocaine and ropivacaine measured at 25 °C. c Potency relative to procaine. d Potency relative to lidocaine. e Cardiovascular (CV) to central nervous system (CNS) toxicity ratio. CV denotes the disappearance of pulse and CNS denotes the onset of seizures. All clinically useful local anesthetics are weak bases, and as such they exist in equilibrium between the neutral, non‐ionized, lipid‐soluble form (B) and the ionized (charged), water‐soluble form (BH+). They are formulated as acidic solutions of hydrochloride salts (pH 4–7), which are more highly ionized and water soluble. The receptor for local anesthetics appears to be located within the pore of the Na+ channel close to the cytoplasm [13] and only the ionized, charged form of the local anesthetic can interact with this receptor [47]. Local anesthetics can cross the cell membrane in two different ways: (1) the ionized form can pass through the open sodium channel, and (2) the non‐ionized, lipophilic form can penetrate through the lipid membrane. The latter seems to be the main method for local anesthetics to access the cell (Fig. 29.4). The pKa of a drug is the pH at which the two forms exist in equal concentrations and is alkaline (pH > 7.4) for all clinically used local anesthetics. The higher the pKa, the greater the degree of ionization or proportion of local anesthetic in the ionized, charged hydrophilic form at physiologic pH, and the slower the onset of action. On the other hand, a local anesthetic with a low pKa will have a greater proportion of the non‐ionized, lipid‐soluble form at physiologic pH and a more rapid onset of action. Lipid solubility is the main determinant of intrinsic local anesthetic potency, and it will determine the clinically relevant concentrations needed to produce effective conduction blockade [48–50]. Increasing lipid solubility facilitates the penetration through lipid membranes, potentially hastening onset of action; however, highly lipid‐soluble agents will also become sequestered within the myelin and other lipid compartments. Thus, the net effect of increasing lipid solubility is delayed onset of action of local anesthetics [51]. On the other hand, sequestration of local anesthetic in myelin and other lipid compartments creates a depot for slow release of the drug, increasing the duration of the effect [51]. It has also been postulated that more lipophilic drugs such as bupivacaine may be less likely to travel by bulk flow than less lipophilic drugs such as mepivacaine, and therefore, more hydrophilic local anesthetics may have greater ability to spread further [52]. Figure 29.4 The cell membrane lipid bilayer with the Na+ channel. Local anesthetics exist as a neutral base (B) and an ionized form (BH+) in equilibrium. The neutral form is lipid soluble and easily crosses the cell membrane. The ionized form is more water soluble and can cross through the open channel. The neutral form can cause membrane expansion and closure of the Na+ channel. The ionized form interacts with its receptor on the intracellular side of the Na+ channel. The degree of protein binding also influences activity of local anesthetics, as only the unbound, free fraction is pharmacologically active. Higher protein binding is associated with increased duration of action. This cannot be explained by slower dissociation kinetics from the Na+ channel, as this dissociation occurs within seconds regardless of the degree of protein binding [53]. Increased duration of action of highly protein‐bound local anesthetics is probably associated with other membrane or extracellular proteins [54]. Lidocaine, procaine, and tetracaine are achiral molecules, while levobupivacaine and ropivacaine are pure S(–)‐enantiomers [45,55]. The rest of the clinically available local anesthetics are racemic mixtures of the R(+)‐ and S(–)‐enantiomers in a 50:50 mixture. Although both enantiomers have the same physicochemical properties, they have different affinities for the ion channels of Na+, K+, and Ca2+, with the R(+)‐enantiomer having greater in vitro potency and thus greater therapeutic efficacy but also greater potential for systemic toxicity [56,57]. There is less potential for nervous and cardiac toxicity with the S(–)‐enantiomer compared with the R(+)‐enantiomer or the racemic mixture [58]. Studies in vitro have characterized the relative potencies of local anesthetic agents, which depend not only on their physicochemical properties (i.e., lipid solubility) but also on the individual nerve fibers and frequency of stimulation [59]. However, in vivo potencies do not necessarily correlate with in vitro studies [60], because of the complex interaction of factors, including site of administration, dose, and volume of local anesthetics, and other environmental factors. Local anesthetics with an amide group, high pKa, and lower lipid solubility show greater differential blockade, with more potent blockade of C fibers than of fast‐conducting A fibers [51,61]. This is believed to be due to the slower diffusion across permeability barriers present in A fibers. The relative order of differential rate of blockade is chloroprocaine > ropivacaine > bupivacaine, levobupivacaine > lidocaine, mepivacaine > etidocaine [62,63]. This is especially true at low concentrations and the differential rate of blockade tends to disappear as local anesthetic concentrations increase. Disposition of local anesthetics within the body after local administration is governed by several competing factors, including bulk flow, diffusion and binding to neural and non‐neural structures, and vascular uptake (Fig. 29.5). The rate and extent of systemic absorption of the local anesthetic are important as toxic plasma concentrations may be achieved. Therefore, local anesthetics with lower systemic absorption will have a greater margin of safety. Systemic absorption depends on several factors, including the site of injection (i.e., vascularity), the intrinsic lipid solubility and vasoactivity of the agent, the dose administered, the presence of additives such as vasoconstrictors, other formulation factors that modify local drug residence and release, the influence of the nerve block in the region (i.e., vasodilation), and the (patho)physiologic state of the patient [45]. In general, areas with greater vascularity will have more extensive and rapid systemic drug absorption than areas with more fat, regardless of the agent used [19]. Areas with greater vascularity will have a greater peak plasma concentration (Cmax) and a shorter time‐to‐peak plasma concentration (Tmax). In an experimental study in pigs, lidocaine rate of absorption following subcutaneous administration was highest in the pectoral region, followed by the face and neck, with the slowest being the abdomen [64]. With regard to specific blocks, the degree of systemic absorption is as follows, in decreasing order: intercostal > epidural > brachial plexus > sciatic/femoral [65]. Following administration of lidocaine in an inverted L nerve block in cows, the serum Cmax was 572 ng/mL, which occurred at Tmax 0.52 h, while lidocaine via a caudal epidural block was undetectable in serum [66]. Systemic absorption of local anesthetic drugs is much lower after spinal (intrathecal) than after epidural administration [67,68]. Normally, the greatest risk of systemic toxicity coincides with Tmax in arterial blood, which will vary from 5 to 45 min after injection, depending on the site of the block, speed of injection, and drug injected [45]. However, Tmax is independent of the dose injected [69]. Faster speed of injection is associated with greater Cmax, and therefore with increased risk of systemic toxicity [70]. Figure 29.5 Disposition of local anesthetics within the body following peripheral administration. Physicochemical properties of local anesthetics will also influence systemic absorption. In general, drugs with greater lipid solubility and protein binding will result in lower systemic absorption and Cmax [45]. Therefore, shorter‐acting amide drugs such as lidocaine and mepivacaine will be absorbed into the systemic circulation more readily than longer‐acting bupivacaine, ropivacaine, and levobupivacaine, probably because of binding of the latter to neural and non‐neural lipid‐rich tissues [19]. Another factor influencing the rate of absorption is the intrinsic vasoactivity of the local anesthetic. Most clinically used local anesthetics cause vasodilation when applied locally, with the exceptions of ropivacaine and levobupivacaine [71,72]. The vasoconstrictive activity of ropivacaine and levobupivacaine results in slower absorption and therefore longer Tmax values [73,74]. The addition of a vasoconstrictor, such as epinephrine, will counteract the inherent vasodilating effects on the local vasculature induced by most agents, delaying their systemic absorption. Hyaluronidase is another additive occasionally added to local anesthetics to improve their anesthetic effect by causing depolymerization of interstitial hyaluronic acid and thus increasing the permeability of the tissues; however, hyaluronidase also enhances systemic absorption and the risk of systemic toxicity (see the section on Additives later in this chapter). There are some formulations, such as local anesthetic‐loaded liposomes, polylactide microspheres, or cyclodextrin inclusion complexes, among others, which are designed to cause a slow release of the drug, providing a local depot of local anesthetic which significantly decreases systemic absorption and prolongs the duration of effect [69,75,76]. When liposome‐encapsulated lidocaine was administered epidurally to dogs, the Cmax was lower while the Tmax and the duration of effect (170 versus 61 min) were significantly longer compared to regular lidocaine [75]. In sheep, intercostal administration of bupivacaine–dexamethasone microspheres prolonged the duration of the block up to 13 days, with plasma concentrations remaining 10 times below the convulsive concentration [77]. Liposome‐encapsulated lidocaine has also been administered topically to cats at a dose of 15 mg/kg, which proved to be safe, with Cmax well below the toxic plasma levels for that species [78]. Administration of different slow‐release lidocaine formulations for sciatic nerve block in postoperative pain models in rats produced analgesia from 3 days up to 1 week and inhibited the development of hyperalgesia [79,80]. A number of newer formulations of local anesthetics have been approved for use in animals that greatly reduce systemic absorption, thereby limiting the extent of their effect to the surgical area, prolonging the analgesic effect, and reducing Cmax and the possibility of systemic toxicity. These are discussed later in this chapter in the Special formulations section. After absorption into the bloodstream, aminoester local anesthetics are rapidly hydrolyzed by plasma cholinesterases, and their distribution into body tissues is limited. Aminoamide local anesthetics are widely distributed into different body organs and tissues. The degree of tissue distribution and binding is normally represented by the pharmacokinetic parameter known as the “apparent volume of distribution at steady state” (Vdss), which is usually paralleled by the degree of protein binding [45]. Only the free, active fraction of the drug, and not the protein‐bound fraction, governs tissue concentration and degree of entry into the central nervous system (CNS) [81]. Amide‐type local anesthetics bind primarily to α1‐acid glycoprotein (AAG) in the plasma and to a lesser extent to albumin [82,83]. In dogs, increasing concentrations of AAG caused an increase in total serum concentration but a decrease in the free fraction, Vdss, and elimination half‐life of lidocaine [81,84]. Because AAG is an acute‐phase protein, its circulating levels will be increased during trauma, surgery, cancer, or any inflammatory state. Therefore, although the total concentration of local anesthetic in plasma will be greater, reflecting the increase in AAG, the unbound (active) drug fraction will remain similar [45,85]. Amide‐type local anesthetics in venous blood undergo first‐pass pulmonary uptake, which effectively decreases the plasma concentration of the drug temporarily [86,87]. Consequently, the lungs are able to attenuate the toxic effects after accidental intravenous injections of local anesthetics. In animals with right‐to‐left cardiac shunts, the pulmonary first‐pass effect is absent and there is an increased risk of toxicity. The pulmonary uptake of a local anesthetic is mostly dependent on its physicochemical properties, mainly lipid solubility and pKa. More lipid‐soluble agents undergo greater pulmonary uptake and those with lower pKa values will have a greater fraction of the non‐ionized base form, which is the form that accumulates in the lung [88]. Decreasing blood pH (i.e., acidemia) decreases the degree of pulmonary uptake of local anesthetics, which may contribute to increased plasma concentrations and promote toxicity [86,88]. The rank order of pulmonary uptake in rat lung slices was found to be bupivacaine > etidocaine > lidocaine [88]. Others have also found greater uptake of prilocaine compared to bupivacaine and mepivacaine in isolated perfused rat lungs, with little evidence of pulmonary metabolism [89]. The mean pulmonary uptake of lidocaine after IV administration in dogs has been calculated to be 63.6% [87]. There is evidence of a pulmonary contribution to lidocaine metabolism using rat pulmonary microsomes in vitro [90]. After an intravenous bolus injection in rabbits, the pulmonary uptake of levobupivacaine was greater than that of ropivacaine (31% versus 23%) [91]. Local anesthetic agents also distribute rapidly and extensively into milk and muscle at concentrations proportional to those in the bloodstream. Drugs that diffuse most readily into milk are those that are relatively lipophilic, non‐ionized, not strongly protein‐bound, and with low molecular weights [92]. Following an inverted L nerve block with 100 mL of 2% lidocaine in adult Holstein cows, the lidocaine Cmax in milk was 300 ng/mL compared with serum Cmax of 572 ng/mL; Tmax in milk was 1.75 h compared with serum Tmax of 0.52 h [66]. The last measurable time of lidocaine detection in milk after inverted L block was 32.5 h with a mean concentration of 46 ng/mL [66]. On the other hand, following caudal epidural administration of 0.22 mg/kg of lidocaine, there was no detectable lidocaine concentration present in any serum or milk sample [66]. The current Food Animal Residue Avoidance Database (FARAD) withdrawal intervals reflect these differences, with a 4‐day meat withdrawal and 72 h milk withdrawal following infiltrative lidocaine administration, but only a 1‐day meat withdrawal and 24‐h milk withdrawal following epidural lidocaine administration. The European Medicines Agency (EMA), on the other hand, establishes a 28‐day withdrawal period for meat and 15‐day milk withdrawal after lidocaine administration by any route. Local anesthetic drugs also cross the placenta and appear in the fetus following administration to the pregnant animal. Ester‐linked local anesthetic agents are rapidly metabolized and placental transfer is limited [45]. Amide‐linked local anesthetic agents can become “trapped” in their ionized forms on the more acidotic fetal side of the placenta, and therefore their net transfer across the placenta is increased [93]. In pregnant ewes, as fetal blood pH decreased from 7.35 to 7.10, the fetal–maternal ratio (F:M) for lidocaine increased from 0.76 to 1.21 [93]. Apart from the pH, the degree of local anesthetic binding to both maternal and fetal plasma proteins is also an important determinant of placental transfer of local anesthetics, as only the unbound, free drug crosses the placenta [94]. Since fetal AAG content and binding are less than maternal [95], the F:M of highly protein‐bound local anesthetics such as bupivacaine (F:M = 0.36) is lower than less protein‐bound drugs such as lidocaine (F:M = 1) [94,96]. The placental transfer of levobupivacaine and ropivacaine is similar to bupivacaine in pregnant ewes [97]. An important consideration when choosing a local anesthetic agent in the pregnant animal is the ability of the neonate to metabolize and excrete the drug after birth. Studies in sheep show that back‐transfer of bupivacaine, but not of lidocaine, from the fetus to the mother occurs [94,96]. Lidocaine and its metabolites monoethylglycinexylidide (MEGX) and glycinexylidide (GX) were detected in fetal urine within 1–2 h following intravenous infusion of lidocaine to pregnant ewes [94]. These studies suggest that lidocaine might be a better option in pregnant animals since the fetus/neonate will be able to readily eliminate the drug. They also suggest that if high plasma concentrations of local anesthetic in maternal blood are likely (i.e., large volumes used for local blockade or inadvertent intravenous administration), it would be beneficial to delay delivery in the case of bupivacaine, but there would be no benefit in doing so in the case of lidocaine [94]. Ester‐linked local anesthetics are cleared mainly in the blood by plasma cholinesterase (also known variably as butyrylcholinesterase, non‐specific cholinesterase, or pseudocholinesterase), where they undergo ester hydrolysis. Esterases present in the liver, red blood cells, and synovial fluid also contribute to the clearance of these drugs [98–100]. Among the ester agents, chloroprocaine is cleared most rapidly due to its faster hydrolysis rate. In vitro half‐lives tend to be very short for the ester‐linked drugs, ranging from 11 s for chloroprocaine in human plasma [101] to 9 s and 12 s for procaine in equine whole blood and plasma, respectively [98], and up to several minutes for tetracaine [45]. In vivo terminal half‐lives are typically longer, probably reflecting slow uptake from the site of administration and/or wide distribution within the body [45,102]. The terminal half‐life of procaine in horses after intravenous administration is 50 min with an apparent volume of distribution of 6.7 L/kg [102]. The hydrolysis products of procaine, chloroprocaine, and tetracaine appear to be pharmacologically inactive. Procaine and benzocaine are hydrolyzed to para‐aminobenzoic acid (PABA) which may, however, cause rare allergic reactions [45]. Cocaine undergoes ester hydrolysis in plasma and liver, but also N‐demethylation in the liver to norcocaine, which subsequently undergoes further hydrolysis [100]. Cocaine is rarely used in veterinary medicine, but illegal use in horses or dogs before races to increase performance and delay the time to exhaustion is possible [103]. Procaine also possesses CNS stimulatory effects and its use is banned in racehorses [102]. Amide‐linked local anesthetics are almost exclusively metabolized in the liver by microsomal enzymes (CYP450). Phase I reactions involve hydroxylation, N‐dealkylation, and N‐demethylation, followed by Phase II reactions where the metabolites are conjugated with amino acids or glucuronide into less active and inactive metabolites. Clearance values differ among species, but typically the rank order of clearance is prilocaine > etidocaine > lidocaine > mepivacaine > ropivacaine > bupivacaine [45]. In humans, prilocaine is cleared most rapidly, with blood clearance values that exceed liver blood flow, indicating extrahepatic metabolism in this species [45]. Hydrolysis of prilocaine produces ortho‐toluidine (o‐toluidine), a metabolite that oxidizes hemoglobin to methemoglobin (MHb) [104]. Lidocaine undergoes hydroxylation and N‐demethylation in the liver. Its two main metabolites are monoethylglycinexylidide (MEGX) and glycinexylidide (GX) in dogs [105], rabbits [106], rats [107], cats [108], horses [109,110], goats [111], and chickens [112], but these metabolites have not been detected in cows [113]. Of these metabolites, MEGX in particular has significant activity (approximately, 70% that of lidocaine) and could potentially contribute to its toxicity during prolonged intravenous infusions [45,110]. Another metabolite of lidocaine, 2,6‐xylidine, is of interest in food‐producing animals as it has been shown to have genotoxic and carcinogenic potential following ingestion in humans. Other amides such as mepivacaine, bupivacaine, and ropivacaine undergo mainly N‐dealkylation and hydroxylation. These agents produce the less toxic metabolite pipecoloxylidide (PPX) [114]. The N‐dealkylated metabolite of bupivacaine, N‐desbutylbupivacaine, is about half as cardiotoxic as bupivacaine, but less toxic to the CNS in rat studies [115]. Some amide metabolites are further conjugated to glucuronide before they are eliminated in the urine or bile [116]. Local anesthetics are poorly water soluble, which limits renal excretion of the unchanged drug. The hydrolysis metabolites of ester‐linked local anesthetics are mainly excreted in urine [117]. Similarly, the metabolites of amide‐linked local anesthetics are eliminated in urine or bile. A small portion of amide‐type local anesthetics is excreted unchanged in urine (4–7% for lidocaine, 6% for bupivacaine, and 16% for mepivacaine in humans; 1.7–2.9% for lidocaine in horses) [118–120]. Patient factors, such as age, may influence the pharmacokinetics of local anesthetics. Absorption of lidocaine from laryngeal spray was higher in dogs less than 20 days of age compared to 2–3‐month‐old puppies [121]. The volume of distribution and the elimination half‐life of lidocaine were greater in neonatal lambs compared with adult sheep [122]. In a pharmacokinetic study of lidocaine in puppies, the elimination rate constant from the central compartment (k10) was lower, and the elimination half‐life was longer in 3–16‐day old compared with 6‐month‐old puppies [123]. When comparing neonatal lambs with adult sheep, hepatic clearance of lidocaine was similar but renal clearance of unchanged drug was greater in the neonate, probably due to decreased protein binding, lower urine pH, and decreased tubular reabsorption because of higher urine flow rates [122]. Plasma hydrolysis of ester‐linked local anesthetics is also affected by age, as observed in human neonates and infants where plasma cholinesterase activity was half that of adults [124]. In geriatric animals, hepatic clearance of local anesthetics may be decreased, and half‐life increased [125,126]. Increased nerve sensitivity to local anesthetics seems to be present during pregnancy with faster onset of conduction blockade [127]. Acute progesterone treatment had no effect on bupivacaine‐induced conduction blockade in the isolated rabbit vagus nerve; therefore, this effect is unlikely to be a direct effect of progesterone on the cell membrane but may involve hormonal effects on protein synthesis [128]. Pregnant ewes were found to clear lidocaine more rapidly, but bupivacaine and ropivacaine more slowly than non‐pregnant ewes [129,130]. This difference may be explained by lidocaine’s clearance being more dependent on hepatic blood flow, which is increased during pregnancy, whereas clearance of bupivacaine and ropivacaine is more dependent on hepatic enzymatic activity, which may be inhibited during pregnancy [45]. Hepatic disease can decrease the rate of metabolism of amide‐linked local anesthetics. Plasma cholinesterase activity is also reduced in the presence of liver disease and during pregnancy, which will decrease the rate of hydrolysis of ester‐linked local anesthetics [131,132]. In general, standard doses may be administered to animals with hepatic disease for single‐dose neural blockade but repeated doses, dosing intervals, and continuous rate infusions need to be adjusted to avoid accumulation and toxicity [45]. A decrease in hepatic blood flow, as can occur during general anesthesia, cardiac disease, or any condition decreasing cardiac output, will decrease hepatic clearance of local anesthetics, especially those more dependent on hepatic blood flow such as lidocaine [45,133]. The Vdss and clearance (Cl) of intravenous lidocaine were significantly decreased in anesthetized compared to awake horses (0.4 versus 0.79 L/kg and 15 versus 29 mL/kg/min, respectively) [134], and in anesthetized compared to awake cats (1.4 versus 1.9 L/kg and 21 versus 26 mL/kg/min, respectively) [108]. Hepatic clearance of other amide‐linked local anesthetics such as mepivacaine or bupivacaine is more dependent on activity of hepatic enzymes and the effect of reduced hepatic blood flow is less pronounced. Renal failure decreases plasma cholinesterase activity by 40% in humans [135]. Aminoamides are excreted mainly as water‐soluble metabolites, which may accumulate in animals with renal failure and contribute to CNS toxicity if they are active (e.g., MEGX and GX) [133]. Fasting has been shown to decrease hepatic clearance of lidocaine in horses [120]. Gastrointestinal disease (e.g., equine colic) may also affect clearance of aminoamide local anesthetics that depend mainly on hepatic blood flow, such as lidocaine, especially if cardiac output is significantly reduced. However, pharmacokinetic parameters of intravenous lidocaine in horses undergoing abdominal surgery for colic are similar to those of healthy, awake horses, with Vdss and Cl values of 0.7 L/kg and 25 mL/kg/min [136]. It was hypothesized that the cardiac output of the horses included in that study might have been increased, rather than decreased [136]. Interestingly, diabetes mellitus increases hepatic clearance of lidocaine, although the excretion of the metabolite MEGX is impaired [137,138]. Concomitant administration of local anesthetics with other drugs may affect their distribution and elimination kinetics. Drugs that decrease plasma or red cell esterase activity, such as neostigmine or acetazolamide, will prolong half‐life of ester‐linked local anesthetics [139,140]. When CYP1A2 and CYP3A4 inhibitors, such as erythromycin, are co‐administered with aminoamide local anesthetics, their hepatic clearance may decrease [141]. β‐Adrenergic receptor blocking drugs reduce liver perfusion and inhibit the activity of hepatic microsomal metabolizing enzymes responsible for the metabolism of aminoamide local anesthetics; hence, greater plasma concentration and decreased elimination will occur when these drugs are co‐administered [142]. Co‐administration of different classes of local anesthetics may also affect their pharmacokinetic parameters. The rate of hydrolysis of chloroprocaine is reduced by concomitant administration of bupivacaine or etidocaine, but not when it is co‐administered with lidocaine or mepivacaine [139,143]. Temperature may also affect the pharmacokinetics and pharmacodynamics of local anesthetics. Lidocaine’s ability to block nerve impulses, both in vitro and in vivo, is potentiated by cooling [144,145]. Conversely, lidocaine uptake by mammalian sciatic nerve is reduced by cooling, with a 45% decrease when the temperature falls from 37 °C to 20 °C [146]. Some clinical studies in humans have observed an increase in the speed of onset of various types of blocks when the temperature of the local anesthetic solution was increased to 37 °C [147–150], although this effect has not been consistent [151,152]. Cooling of the local anesthetic solution increases the pKa and the relative amount of ionized active form, while warming the solution decreases the pKa and increases the amount of non‐ionized lipid‐soluble form [146]. These pKa changes may explain the increased potency of local anesthetics with cooling and the hastening of onset of action with warming. Baricity is one of the most important physical properties of local anesthetics during subarachnoid or intrathecal administration as it will affect the distribution and spread of the solution, and therefore impact the characteristics of the block [153]. Baricity of a local anesthetic solution is the calculated ratio of the density of the solution to the density of the cerebrospinal fluid (CSF), both measured at the same temperature, which is normally 37 °C. Density is the weight in grams of 1 mL of the solution, and it is inversely related to its temperature [154]. An isobaric solution has a baricity ratio of 1. If the ratio is > 1, the solution is hyperbaric, and if it is < 1, it is hypobaric. At room temperature, most commercially available local anesthetic solutions are isobaric with respect to the CSF, but when they are warmed to body temperature, they become hypobaric [154]. The densities of commercial 2% lidocaine and 0.5% and 0.75% bupivacaine are lower than that of human CSF at 37 °C, which makes them relatively hypobaric [155]. Dilution of these solutions with water makes them increasingly hypobaric [155]. When local anesthetics are mixed with dextrose or hypertonic saline, the resulting solution is hyperbaric [154,156]. Neurotoxicity has been observed with hyperbaric bupivacaine when high concentrations and doses are administered intrathecally in dogs (≥ 10 mg of 1% or 2% bupivacaine in 10% glucose solution), but not with low concentrations and doses (5 mg of 0.5% bupivacaine in 10% glucose solution) [157]. High concentrations and doses of hypobaric bupivacaine (20 mg of 2% bupivacaine in water) were not associated with neurotoxicity when administered intrathecally to dogs [157]. Hypobaric solutions, when injected into the subarachnoid space, will migrate to non‐dependent areas, because their density is lower than that of the CSF, while hyperbaric solutions will migrate to dependent areas. This migration allows preferential blockade to occur on the surgical side, with unilateral spinal anesthesia being possible when low doses of local anesthetics are used [158]. Isobaric solutions will migrate to both sides of the spinal cord, causing bilateral spinal block. In humans, it is reported that unilateral spinal block results in a four‐fold reduction of the incidence of clinically relevant hypotension with more stable cardiovascular parameters as compared with conventional bilateral spinal block [159]. Because only small amounts of local anesthetic solution are injected, the extent of spinal block is reduced, and the resolution of sensory and motor blockade is faster [159]. Mixtures of local anesthetic agents with additives such as solvents or vasoconstrictors, or with other drugs such as opioids, may alter the density and baricity of the solution. When 0.125–0.5% bupivacaine solutions are mixed with fentanyl (0.005%), sufentanil (0.005%), or morphine (0.1%), the resultant solutions are hypobaric [160]. The mixture of 2% lidocaine and epinephrine (1:200,000) results in a hyperbaric solution [160]. There is some variation in the density of the CSF among individuals [161]. Density of the CSF may also be influenced by physiologic status (e.g., density is decreased during pregnancy in humans) [162]. Therefore, there may be some interindividual variation in clinical response to intrathecal solutions, especially with those that are marginally hypo‐ or hyperbaric [153]. Epinephrine has been used as an adjunct to local anesthetics for more than a century. The rationale behind its use is that it causes vasoconstriction and therefore decreases the systemic absorption of the local anesthetic agent, which decreases the dose of local anesthetic required and prolongs its duration of effect [163,164]. Decreased systemic absorption also reduces the local anesthetic Cmax, which decreases the probability of systemic toxicity. Several studies have demonstrated decreased Cmax of local anesthetics when administered with epinephrine both in peripheral and neuraxial blocks [164–167]. In general, the greatest effects are observed with shorter‐acting rather than with longer‐acting agents. In addition to this pharmacokinetic interaction, epinephrine seems to have analgesic effects on its own when administered epidurally or intrathecally by stimulating α2‐adrenergic receptors, thereby inhibiting presynaptic neurotransmitter release from C and Aδ fibers in the substantia gelatinosa of the spinal cord dorsal horn [168–170]. It has also been shown that α2‐adrenergic receptors can modify certain K+ channels in the axons of peripheral nerves, potentiating the impulse‐blocking actions of local anesthetics [171,172]. A later study in rats also showed that local infiltration of epinephrine causes cutaneous anesthesia mediated by activation of local α1‐adrenergic receptors [173]. Therefore, it seems that pharmacokinetic and pharmacodynamic interactions between epinephrine and local anesthetics are responsible for the increased duration and intensity of the block when administered in combination. A potential concern when epinephrine is co‐administered with local anesthetics is a decrease in peripheral nerve or spinal cord blood flow, which could cause nerve or spinal cord ischemia. However, research studies with radiolabeled microspheres in dogs and cats show that epinephrine injected intrathecally causes regional dural vasoconstriction but does not reduce spinal cord or cerebral blood flow [174,175]. This is supported by many years of clinical experience using epinephrine‐containing solutions for neuraxial anesthesia and the absence of observed detrimental effects on spinal cord function [172,176]. In vitro studies in rats showed that sciatic nerve blood flow is reduced by injection of lidocaine without epinephrine, but that the reduction is more pronounced when epinephrine (5 μg/mL) is added [177]. However, a more recent in vivo rat study using radiolabeled microspheres showed that lidocaine with or without epinephrine (10 μg/mL) does not reduce sciatic nerve or surrounding skeletal muscle blood flow [178]. The authors of this study concluded that mechanisms other than local vasoconstriction might contribute to the prolongation of lidocaine peripheral nerve blockade by epinephrine. Systemic absorption of epinephrine administered in combination with local anesthetics can also cause cardiovascular effects characterized by an increase in heart rate, stroke volume, and cardiac output, and a decrease in peripheral vascular resistance [179]. A study in humans also showed an improvement in left ventricular diastolic function with epinephrine added to local anesthetics, in contrast with norepinephrine, which impaired it [179]. Excessive plasma concentrations of epinephrine could precipitate tachycardia and arrhythmias. The recommended concentrations of epinephrine for addition to local anesthetic solutions for clinical use range between 1:400,000 (1 mg/400 mL or 2.5 μg/mL) and 1:200,000 (1 mg/200 mL or 5 μg/ mL] [172]. A 1:200,000 concentration can be obtained by adding 0.1 mL of a 1:1000 epinephrine solution (0.1 mg) into 20 mL of local anesthetic solution. Concentrations in excess of 5 μg/mL do not provide any additional decrease in Cmax and should therefore be avoided in light of the potential for systemic side effects [45]. Market preparations of local anesthetics that contain epinephrine have lower pH values than plain or freshly prepared solutions. The lower pH of these epinephrine‐containing preparations could potentially decrease the amount of non‐ionized form, thereby slowing the onset of action. Other vasoconstrictors such as phenylephrine or methoxamine may be added to prolong the duration of the effect of local anesthetics by decreasing their systemic absorption [180]. Some degree of pharmacodynamic interaction may also exist as the infiltration of these α1‐adrenergic receptor agonists caused cutaneous anesthesia in rats [173]. But in contrast with epinephrine, these other agents lack α2‐adrenergic receptor effects and, therefore, potential interactions with local anesthetics mediated by these receptors are not possible. Moreover, phenylephrine, but not epinephrine, caused a significant decrease in sciatic nerve and skeletal muscle blood flow when administered in combination with lidocaine [178], which could potentially cause complications due to nerve ischemia. Vasoconstrictors should be avoided for blockade of areas with erratic blood supply or without good collateral perfusion (e.g., intravenous regional anesthesia, teat blocks, or large areas of skin) because of the possibility of vasoconstriction‐induced tissue ischemia and necrosis. Phentolamine, a non‐selective α‐adrenergic receptor antagonist, has been shown to reverse prolonged local anesthetic‐induced block when administered in combination with vasoconstrictors [181]. A commercial preparation of phentolamine mesylate (OraVerse®) has been approved for the reversal of soft tissue anesthesia and the associated functional deficits resulting from local dental anesthesia in humans. Hyaluronidase, an enzyme that depolymerizes hyaluronic acid, the main cement of the interstitium, may be added to local anesthetics to improve tissue penetration and thereby shorten onset and increase spread of the block [182]. The addition of hyaluronidase raises the pH of the anesthetic solution to a slightly more physiologic level, which may contribute to the shortening of onset by increasing the amount of non‐ionized drug. However, local anesthetic Cmax and the risk of systemic toxicity may also increase. Human studies show diverse results with respect to improved efficacy. Some human studies show better quality of peribulbar or retrobulbar block and shorter onset of action when hyaluronidase is added at concentrations as low as 3.5 IU/mL to mixtures of 0.5% or 0.75% bupivacaine and 2% lidocaine [183–185], while others show no benefit when hyaluronidase is added at concentrations as high as 150 IU/mL to a mixture of 0.75% bupivacaine and 2% lidocaine for peribulbar block [186,187]. Hyaluronidase added to bupivacaine with epinephrine (1:200,000) for brachial plexus block did not increase the speed of onset of anesthesia or reduce the incidence of inadequate nerve block in humans [188]. When used in infiltration anesthesia, hyaluronidase added at 15 IU/mL to 1% lidocaine increased the area of desensitized skin, but pain on injection also increased compared with plain 1% lidocaine in humans [189]. The addition of hyaluronidase seems particularly advantageous in ophthalmic blocks in humans, as it has been shown to limit the acute intraocular pressure increase secondary to periocular injection and seems to have a protective effect against local anesthetic‐induced myotoxicity resulting in postoperative strabismus [190]. In dogs, the addition of 400 IU of hyaluronidase to 1.06 mg/kg of 0.5% levobupivacaine for lumbosacral epidural block decreased the onset from 15 to 5 min, but it also decreased the duration of block, while dermatomal spread was unchanged [191]. Another study in dogs looking at the effects of hyaluronidase added to ropivacaine or bupivacaine for femoral–ischiatic blocks showed highly variable onset and offset times and no difference compared to the addition of saline [192]. The addition of hyaluronidase to lidocaine for infiltration block does not delay wound healing [193]. The pH of commercially available local anesthetic solutions is normally acidic to enhance stability and solubility and extend shelf‐life [194,195]. Alkalinization of the solution by addition of sodium bicarbonate causes an increase in the amount of local anesthetic in the non‐ionized form, which is the lipid‐soluble fraction able to cross the axonal membrane, thereby shortening the onset of the block. The intensity and duration of the block may also increase due to an increase in the transmembrane pH gradient, causing ion trapping of the ionized active form inside the nerve. The efficacy of alkalinization depends on the local anesthetic solution, the site of the block, and the concurrent addition of epinephrine. Buffering of 1% lidocaine, 1% mepivacaine, or 0.5% bupivacaine with sodium bicarbonate for intradermal administration does not affect the onset, extent, and duration of skin anesthesia in humans [196,197]. Addition of bicarbonate to lidocaine for median nerve block in humans increased the rate of motor block without changing the onset or extent of sensory block [198]. Similarly, alkalinization of 1% lidocaine or 0.25% bupivacaine to a pH of 7.4 did not prolong infraorbital nerve block duration in rats [199]. Studies using buffered local anesthetics during epidural anesthesia show conflicting results. Some studies show shorter onset of epidural block when 1.5–2% lidocaine, 2% mepivacaine, or 0.5% bupivacaine solutions are alkalinized with bicarbonate [200–202], while others show no shortening of onset with buffered 2% lidocaine or 0.5% bupivacaine [203–205]. The alkalinization of 0.75% ropivacaine solution does not decrease sensory or motor block onset but increases the duration of the epidural block [206]. In femoral and sciatic nerve blocks, the effects of alkalinization on the onset of sensory analgesia and motor block were more evident with 2% mepivacaine, but for brachial plexus axillary block, the greatest effect was observed with 2% lidocaine [202]. Nonetheless, in studies where hastening of block onset occurred with alkalinization, the decrease was less than 5 min when compared with commercial preparations. In addition, it seems that the effect of alkalinization is mainly observed when epinephrine is also added to the solution [207]. Thus, the value of alkalinization of local anesthetics appears debatable as a clinically useful tool to improve anesthesia [19]. Alkalinization has a greater effect when the local anesthetic is administered into an acidic environment, as with intravesicular instillation where the urine is normally acidic. Intravesicular instillation of 5% lidocaine buffered with an equal volume of 8.4% sodium bicarbonate to a pH of 8.0 provided local anesthesia of the bladder submucosa as indicated by the rapid decrease in pain scores in human patients with interstitial cystitis [208]. However, intravesicular administration of alkalinized lidocaine for up to three consecutive days had no apparent beneficial effect on decreasing recurrence rate and severity of clinical signs in cats with obstructive idiopathic lower urinary tract disease [209]. Buffered local anesthetics also have a greater effect when topically administered to the cornea. The corneal permeability of topically applied lidocaine increased when the pH of the solution was buffered from 5.2 to 7.2, with greater concentrations of lidocaine found in the aqueous humor [210,211]. Buffering of the local anesthetic solution with bicarbonate decreases pain on injection when administered subcutaneously and also decreases pain when an epidural catheter is inserted [194,195,212]. Reduction of pain on injection seems to be enhanced by additional warming of the solution to body temperature [212]. The most common dose of sodium bicarbonate used is 0.1 mEq per mL of local anesthetic solution. The addition of bicarbonate may cause precipitation of the solution, especially with bupivacaine and etidocaine when the pH rises above 7.0 [213]. Mepivacaine may also precipitate at a pH above neutral within 20 min [214]. Therefore, it is recommended to use the mixed solution immediately after the addition of sodium bicarbonate. Carbonation of local anesthetics by adding carbon dioxide (CO2) is sometimes used to decrease onset and improve quality of the block. The addition of CO2 to a solution of lidocaine decreased the amount of lidocaine needed to achieve conduction block in vitro [215]. This potentiation of local anesthetic block is possibly due to a decreased intracellular pH, causing ion trapping. Carbonated lidocaine administered epidurally shortened the onset and improved the block in humans [216,217]; however, it did not offer any advantage over the hydrochloride salt for caudal epidural anesthesia in horses [218]. The addition of α2‐adrenergic receptor agonist drugs to local anesthetics during regional blocks is being increasingly used in veterinary medicine. Clonidine has been extensively used in humans to prolong the duration of intrathecal, epidural, and peripheral nerve blocks. Meta‐analyses and systematic reviews clearly show an analgesic benefit from the addition of clonidine to local anesthetics [219]. In large animals, epidural or intrathecal xylazine has been used in combination with lidocaine since the early 1990s to prolong the analgesic effect [220]. In dogs, a study showed that xylazine administered epidurally in combination with lidocaine produced the longest analgesic effect compared with clonidine, detomidine, romifidine, and dexmedetomidine, which may be attributed to xylazine’s local anesthetic‐like properties and its lower lipophilicity compared with the other α2‐adrenergic receptor agonists [221]. Medetomidine administered either perineurally or systemically (0.01 mg/kg) in combination with mepivacaine for radial nerve block in dogs prolonged the duration of sensory and motor blockade, with residual sensory blockade persisting beyond the observable sedative effects [222]. Dexmedetomidine is now the most widely used α2‐adrenergic receptor agonist regional anesthesia adjuvant in humans and animals. Studies in rats showed that administration of dexmedetomidine in combination with bupivacaine or ropivacaine enhances sensory and motor blockade in sciatic nerve block without inducing neurotoxicity [223,224]. In fact, addition of dexmedetomidine to ropivacaine for sciatic nerve block in rats not only prolonged the duration of sensory and motor block, but also markedly reduced ropivacaine‐induced neurotoxicity dose‐dependently [225]. Evidence in humans supports block prolongation from 1–8 h when dexmedetomidine is added depending on the type of block and local anesthetic used, but it also increases the incidence of bradycardia and sedation intraoperatively [226]. Several studies involving the addition of dexmedetomidine to hindlimb nerve blocks in dogs, both experimental and clinical, report contradictory results. Some studies showed that dexmedetomidine, at doses ranging between 0.15 and 2 μg/kg, combined with ropivacaine for sciatic and saphenous or femoral nerve blocks prolonged the duration of sensory but not motor blockade [227,228]. Another study showed that sciatic and femoral nerve blocks using bupivacaine plus dexmedetomidine (0.1 μg/kg) had a similar degree and duration of analgesia (up to 24 h) compared with epidural bupivacaine plus buprenorphine [229]. However, a two‐center study using 0.5 μg/kg dexmedetomidine in combination with ropivacaine for sciatic and femoral nerve block did not reduce the use of postoperative methadone compared with ropivacaine alone [230]. Epidural administration of dexmedetomidine and bupivacaine in dogs undergoing pelvic limb surgery provided a similar degree of analgesia compared with bupivacaine plus morphine; however, the increased duration of motor blockade was a clinical concern [231]. In cats, dexmedetomidine has also been used in combination with local anesthetics. A clinical study showed that dexmedetomidine combined with bupivacaine intraperitoneally during ovariohysterectomy produced a similar duration and degree of analgesia than bupivacaine combined with epinephrine [232]. An experimental study in cats using dexmedetomidine combined with bupivacaine for sciatic and femoral nerve blocks could not demonstrate a benefit in terms of degree and duration of analgesia compared with bupivacaine alone, although the results may have been influenced by the administration of atipamezole [233]. The increased duration of analgesia caused by adding α2‐adrenergic receptor agonists to local anesthetics is postulated to be due to hyperpolarization of C fibers through blockade of the so‐called “hyperpolarization‐activated cation current” [234,235]. α‐Adrenergic receptors do not seem to be implicated as administration of α‐adrenergic receptor antagonists neither reverses the conduction block nor decreases the duration of the block [234–236]. Local anesthetic mixtures of aminoamide agents consisting of an intermediate‐acting agent, such as lidocaine or mepivacaine, combined with a long‐acting agent, such as bupivacaine, are used in the belief that the combination will provide a shorter block onset and a similar duration than the long‐acting agent administered alone. However, these mixtures produce unpredictable and variable clinical results. Clinical studies in humans using a 50:50 mixture of lidocaine or mepivacaine with bupivacaine or ropivacaine for peripheral nerve blocks show a shorter onset of effect, but also a shorter duration of action, compared with the administration of bupivacaine or ropivacaine alone [237–239]. The epidural or intrathecal combination of lidocaine and bupivacaine in cows [240], cats [241], and humans [242–245] produced similar sensory block onset than either agent alone, and the duration was intermediate between the two agents or similar to bupivacaine. When chloroprocaine, a short‐onset, short‐duration‐of‐action aminoester local anesthetic, is administered prior to bupivacaine, the duration of bupivacaine‐induced blockade is decreased. This may be due to an inhibitory effect caused by chloroprocaine metabolites on the Na+ channel receptor site for bupivacaine [246]. Mixing commercial preparations of chloroprocaine and bupivacaine resulted in a pH of 3.6 and nerve blockade with characteristics of a chloroprocaine block [247]. When the pH of the mixture was increased to 5.56, the nerve block resembled that of bupivacaine. Therefore, mixing commercially available solutions of local anesthetics results in unpredictable blockade that will depend on a number of factors, including the pH of the final mixture [247]. Furthermore, local anesthetic toxicity of combinations of drugs is additive [248]. Tachyphylaxis to local anesthetics is defined as a decrease in duration, segmental spread, or intensity of a regional block despite repeated constant dosages [249]. In 1969, Bromage described that repeated injection of a constant dose of epidural lidocaine led to a reduction in both the number of dermatomes blocked and the duration of the block [250]. The incidence of this phenomenon in veterinary medicine is unknown, and probably is largely unrecognized. Tachyphylaxis appears neither to be linked to structural or pharmacologic properties of the local anesthetics, nor the technique or mode of administration, as it can occur with both ester‐ and amide‐linked local anesthetics and with either neuraxial or peripheral nerve blocks [250–253]. Bromage found that tachyphylaxis to local anesthetics is promoted by longer interanalgesic intervals between injections [250]. If local anesthetic injections were repeated at intervals short enough to prevent return of pain or at intervals with pain of less than 10 min duration, tachyphylaxis did not occur and augmentation of the analgesic effect was noted. Conversely, if the patient experienced pain between local anesthetic administrations for more than 10 min, tachyphylaxis occurred more rapidly. The mechanisms underlying tachyphylaxis may involve both pharmacokinetic and pharmacodynamic aspects. Suggested pharmacokinetic mechanisms include local edema, increased epidural protein concentration, changes in local anesthetic distribution in the epidural space, a decrease in perineural pH (limiting the diffusion of local anesthetic from the epidural space to binding sites at the Na+ channel), an increase in epidural blood flow, or an increase in local metabolism (favoring clearance of local anesthetics from the epidural space) [249]. Other pharmacodynamic mechanisms have also been suggested, such as antagonistic effects of nucleotides or increased Na+ concentration, increased afferent input from nociceptors, or receptor downregulation of Na+ channels [249]. A human study with repeated injections of epidural lidocaine showed lack of changes in the distribution or rate of elimination of lidocaine from the epidural space [253] and another study in rats failed to show an effect of tissue pH on the development of tachyphylaxis to bupivacaine [251]. Tachyphylaxis to local anesthetics does not result from reduced drug effectiveness at the nerve itself [254], but it seems to be mainly mediated by a spinal site of action [255]. Tachyphylaxis and central hyperalgesia seem to be related, as evidenced by studies in rats, where tachyphylaxis occurred only under conditions where they concurrently developed hyperalgesia in the tested paw [256]. If only non‐noxious motor tests were used to test the duration of the block, tachyphylaxis did not occur. Moreover, it has been shown that drugs that prevent hyperalgesia at spinal sites, such as NMDA receptor antagonists [256] and nitric oxide synthase inhibitors [257], prevent the development of tachyphylaxis. Therefore, it seems that a spinal nitric oxide pathway is involved in the development of tachyphylaxis to local anesthetics [243]. Additionally, descending pathways do not seem necessary for the development of tachyphylaxis since it occurs even after spinal cord transection at the tenth thoracic level in rats [255]. Local anesthetic switching has also been proposed when tachyphylaxis to a local anesthetic agent develops. This approach has been successful in humans with cancer‐related pain in whom intrathecal morphine and bupivacaine were not effective and substitution of bupivacaine with lidocaine improved analgesia [258]. This aminoester is used as a topical anesthetic for the cornea and is commercialized as a 0.5% preparation. It is reported to produce quick desensitization of the cornea (within 1 min) and a duration of maximal corneal anesthesia of 25 min in dogs [259] but only 5 min in cats [260]. Reduced corneal sensitivity lasts up to 55 min in dogs and 25 min in cats after 2 drops of the commercial preparation. One of the reported side effects of topical local anesthetics in the eye is that they reduce tear production due to reduced corneal sensation. Also, if used repeatedly, topical local anesthetics may slow corneal healing [261]. This ester of para‐aminobenzoic acid is also used for topical anesthesia of the cornea and is commercialized as a 0.4% ophthalmic solution. It is reported to cause rapid desensitization of the cornea (within 1 min) and to have a duration of up to 45 min in dogs [262]. This agent has a quick onset and short duration of effect (30–60 min) because of its rapid hydrolysis in blood [19]. Epinephrine may be added to prolong its duration of effect. Its potential for systemic toxicity is minimal, but it occasionally causes allergic reactions due to a hydrolysis metabolite (PABA). Procaine is used in veterinary medicine for infiltration and nerve blocks at concentrations of 1–2% [263]. It is rarely used for topical anesthesia, as it is not very effective via this route [263]. In humans, it is sometimes administered intrathecally for short procedures [264]. Intravenous procaine is a CNS stimulant in horses [102]. Because of this property, and its analgesic effect when used for peripheral nerve blocks, it has been illegally used in racehorses [263]. Procaine is sometimes added to drug formulations (i.e., procaine penicillin) to prolong the duration of effect. This agent is also a fast‐acting and short‐lasting local anesthetic, available exclusively for topical anesthesia. It causes methemoglobinemia in several animal species and therefore it is no longer used in clinical practice. Benzocaine is also an anesthetic for fish when added to water [265]. This agent is similar to procaine, with a fast onset and short duration of action (30–60 min). It is available in concentrations of 1% to 3%. It is not widely used in veterinary medicine, but in humans, its use has re‐emerged for short‐duration epidural and intrathecal anesthesia because it is associated with a lower incidence of transient neurologic symptoms compared with lidocaine [266]. It may also be used for local infiltration blocks when a short duration of effect is required. Tetracaine is also called amethocaine. It is rarely used in veterinary medicine. In humans, it is most commonly used for intrathecal anesthesia because it has a fast onset (3–5 min) and its effect lasts 2–3 h [267]. It is rarely used for other forms of regional anesthesia due to its extremely slow onset and potential for systemic toxicity [267]. It is an excellent topical anesthetic and because of this property, it is included in topical anesthetic solutions such as ophthalmological preparations (0.5% and 1.0% aqueous solutions, or a 0.5% viscous formulation). A study in horses showed that the viscous formulation resulted in the greatest decrease in corneal sensitivity and the longest duration of action (30 min) compared with aqueous solutions of proparacaine or tetracaine (20 min) [268]. The absorption of tetracaine from mucous membranes is very rapid and several fatalities have been reported after its use for endoscopic procedures in humans [269]. Human studies have shown that tetracaine topical preparations, including a lidocaine–tetracaine patch (Synera®, Rapydan®), provide faster and better dermal anesthesia than the eutectic mixture of lidocaine and prilocaine (EMLA® cream) [270,271]. Lidocaine remains the most versatile and most widely used local anesthetic in veterinary medicine because of its fast onset, moderate duration of effect, and moderate toxicity. It is available as 0.5%, 1%, 1.5%, and 2% solutions. Lidocaine is used for infiltration anesthesia, peripheral nerve blocks, epidural and intrathecal blocks, and intravenous regional anesthesia. The duration of plain lidocaine is approximately 1 h, which can be prolonged up to 3–4 h with the addition of epinephrine [19]. It is also commonly used topically for laryngeal desensitization before tracheal intubation (2%, 4%, or 10% spray solution). For dermal anesthesia, it is available in different formulations such as the eutectic mixture with prilocaine (2.5% EMLA® cream), as patches of lidocaine alone (5% Lidoderm®), mixed with tetracaine (Synera®, Rapydan®), or mixed with bupivacaine (Tri‐Solfen®) (see Special formulations section later in this chapter). Lidocaine has numerous non‐anesthetic uses when administered intravenously. It is a Class Ib antiarrhythmic drug. It also reduces the requirements for inhalational anesthetics when administered intravenously in different species, including dogs [272–274], cats [275], goats [111], horses [276,277], and calves [278]. It is also an analgesic drug for different types of pain when administered systemically, as shown in human patients [279,280] and experimental studies in laboratory animals [281,282]. Administration of intravenous lidocaine (2 mg/kg bolus followed by 0.05 mg/kg/min) produced thermal antinociception in horses [283]. However, it did not affect the thermal threshold in cats (plasma concentrations up to 4.3 μg/mL) [284], or the electrical threshold in dogs (2 mg/kg IV bolus followed by an infusion of up to 0.1 mg/kg/min) [285]. The mechanism by which systemically administered lidocaine produces analgesia is uncertain but is thought to include action at Na+, Ca2+, and K+ channels and the NMDA receptor [39–41,43,44]. Lidocaine also possesses anti‐inflammatory effects, which may be important in producing analgesia because inflammatory mediators augment neuronal excitability [286]. In addition, some studies show that lidocaine administered as an intravenous infusion perioperatively may improve gastrointestinal motility, reducing reflux, jejunal distension, and peritoneal fluid accumulation, and preventing the development of postoperative ileus in horses with colic of varying etiologies [287–289]. This beneficial effect on motility may be especially true in cases with small intestinal lesions and ischemia‐reperfusion injury, and it may be enhanced if lidocaine is administered early during the intraoperative period [290]. However, a single‐center study showed no effect on the prevalence of postoperative reflux, total reflux volume, and duration of reflux, or postoperative survival in horses undergoing surgical management of small intestinal disease [291]. In healthy horses, lidocaine administration as an intravenous infusion has no effect on gastrointestinal transit time [292] or may prolong it [293]. The disposition of lidocaine after intravenous administration has been described for sheep [122], dogs [105,123,294], cats [108], horses [134], cows [113], and chickens [112] (Table 29.3). This agent has a pharmacologic profile very similar to lidocaine, with a slightly longer duration of effect (up to 2 h), probably because of slightly less intrinsic vasodilatory properties. It is available at concentrations from 0.5% to 2%. Its use in clinical practice is similar to lidocaine, except that it is not routinely used for intravenous regional anesthesia or for obstetric procedures due to its very slow metabolism in the fetus and newborn [267]. Unlike lidocaine, mepivacaine is not an effective topical anesthetic [267]. It is the preferred agent for diagnostic peripheral nerve blocks in horses because of its lower neurotoxicity compared with other local anesthetics [295]. This is a highly lipophilic agent, about four times as potent as lidocaine, and with slow onset of action (20–30 min) and a long duration of effect (3–10 h) [267]. It is used in concentrations, ranging from 0.125% to 0.75%. Its clinical uses include infiltrative, peripheral nerve, epidural, and intrathecal blocks. Bupivacaine is not used for topical anesthesia, and it is not recommended for intravenous regional anesthesia because of its high cardiotoxicity potential. It possesses intrinsic differential blocking properties, especially at low concentrations, and therefore is indicated when sensory blockade accompanied by minimal motor dysfunction is desired. Table 29.3 Pharmacokinetic parameters (mean ± SD) of intravenous lidocaine in domestic species. t 1/2, terminal half‐life; Vdss, apparent volume of distribution in steady state; Cl, total body clearance. This is the S(–)‐enantiomer (“levorotatory” isomer) of bupivacaine, with slightly less cardiotoxic potential than the racemic mixture. Its physicochemical properties and clinical uses are the same as bupivacaine. This agent is structurally related to mepivacaine and bupivacaine, but it is marketed as the pure S(–)‐enantiomer to reduce the cardiotoxicity associated with the R(+)‐enantiomer. It is slightly less potent than bupivacaine and is available in concentrations of up to 1%. Its clinical uses are the same as bupivacaine, with similar onset of effect, a marginally shorter sensory blockade (up to 6 h), and a slightly lower degree of motor blockade at equipotent doses [251]. It has a biphasic effect on peripheral vasculature, causing vasoconstriction at concentrations below 0.5% and vasodilation at concentrations over 1% [296]. In addition to standard local anesthetic preparations, there are several special formulations that improve topical absorption and/or prolong their effects (Table 29.4). These formulations include encapsulation in liposomes, association with biopolymers, complexation in cyclodextrins, incorporation into transdermal non‐liposomal carriers, and other carrier systems. These carriers present in different physical states, including solids (patches), semisolids (gel, cream, and ointment), and liquids (emulsion and dispersion). Table 29.4 Clinically relevant local anesthetic commercial preparations, indications, and doses. a Intrathecal dose is one‐tenth of epidural dose. Ca, Canine; Fe, Feline; Eq, Equine; Bo, Bovine; Ov, Ovine; Cp, Caprine; Sw, Swine. Lidocaine patches are made of a nonwoven polyester felt backing with adhesive material, and commercially available products contain 5% lidocaine (50 mg of lidocaine per gram of adhesive, with a total of 700 mg lidocaine per patch measuring 10 × 14 cm). They consist of a single‐layer matrix that allows lidocaine to diffuse across the different layers of the skin via passive diffusion down a concentration gradient. The patch itself is an occlusive delivery system that prevents water loss from the skin, increasing the hydration of the stratum corneum, and therefore enhancing the absorption of the drug through the skin [297]. The pharmacokinetics of lidocaine patches have been described in different species, with a low systemic absorption observed in cats [298], dogs [299,300], and horses [301,302] when they were applied to intact, clipped, and surgically prepared skin. When they were applied on a surgical incision in dogs, the area under the curve lidocaine plasma concentration was significantly higher than when applied on intact skin [303], although these levels were well below the reported concentrations measured when lidocaine is administered intravenously at clinically recommended doses [273]. The efficacy of lidocaine patches to provide analgesia in clinical conditions has been evaluated in dogs undergoing ovariohysterectomy and hemilaminectomy surgery, with no improvement of postoperative pain scores when they are used in combination with other analgesic therapies [304,305]. A study in horses showed a reduction in the visual analog scale scores to pinprick when a 5% lidocaine cream was used, but not when lidocaine patches were applied proximal to the carpus [302]. Another later study in horses showed that lidocaine patches applied over the withers and saddle area increased local thermal and mechanical nociceptive thresholds under experimental conditions [306]. This study also tested eutectic cream mixtures of lidocaine with prilocaine and lidocaine with tetracaine and found a greater intensity and longer duration of local thermal and mechanical antinociception compared to the lidocaine patches in horses [306]. A commercially available topical anesthetic in a gel base (Tri‐Solfen®) consisting of 5% lidocaine hydrochloride and 0.5% bupivacaine hydrochloride, mixed with epinephrine 1:2000 for hemostasis, and 0.5% cetrimide as an antiseptic, has been registered in Australia and New Zealand since 2006 to provide pain relief in lambs and calves. It has also been approved in 2022 in the United Kingdom for the alleviation of pain in pigs. When applied directly over the disbudding wound, this preparation was reported to be effective to mitigate postoperative pain in calves [307,308], causing no toxic effects or negative impact on wound healing at doses up to five times the recommended dose, and with evidence of lower levels of bacterial colonization [309]. It has also been shown to reduce pain in lambs following mulesing, castration, and tail docking [310,311], in dairy cows during treatment of hoof lesions [312], and in piglets following castration [313]. Liposome‐encapsulated bupivacaine (Nocita®) is a sterile, non‐pyrogenic, preservative‐free, aqueous suspension of multivesicular lipid‐based particles containing bupivacaine at a concentration of 13.3 mg/mL. The liposomes are microscopic structures made of nonconcentric lipid bilayers resembling a honeycomb‐like matrix designed to allow a gradual release of bupivacaine from the vesicles for 72 h, as the lipid bilayers break down. Liposome‐encapsulated bupivacaine was approved by the United States Food and Drug Administration (FDA) in 2016 in dogs for single‐dose infiltration into the surgical site for cranial cruciate ligament surgery at a dose of 5.3 mg/kg. In 2018, it also obtained FDA approval for peripheral nerve blocks for onychectomy in cats at a dose of 5.3 mg/kg per paw (10.6 mg/kg total dose). If a greater volume is needed, it may be mixed with 0.9% saline or another isotonic solution (e.g., Lactated Ringer’s solution) in a 1:1 ratio [314]. Mixing the product with sterile water or another hypotonic solution is not recommended as this may disrupt the liposomes. Several studies have shown that locally infiltrated liposome‐encapsulated bupivacaine is more effective than placebo (significantly lower pain scores) in dogs undergoing stifle surgery [315], and at least as effective as local infiltration with 0.5% bupivacaine hydrochloride (similar postoperative pain scores) in dogs undergoing tibial plateau leveling osteotomy [316] and in cats undergoing ovariohysterectomy [317]. Dogs administered liposome‐encapsulated bupivacaine, however, were less likely to require rescue analgesia and received lower amounts of opioids than dogs administered 0.5% bupivacaine hydrochloride [316]. There are also some pre‐clinical studies in horses using liposome‐encapsulated bupivacaine for perineural analgesia in experimentally induced lameness. Compared with bupivacaine hydrochloride at milligram‐equivalent doses, liposome‐encapsulated bupivacaine ameliorated forelimb lameness in most horses (5/6), and with a similar duration of effect (median 4.5 h) [318]. However, in another study using a different experimental model of lameness, horses treated with bupivacaine hydrochloride had reduced mechanical nociceptive thresholds and improved objective lameness parameters for only 1 h post‐block compared with a duration of up to 24 h in horses treated with liposome‐encapsulated bupivacaine [319]. A novel formulation of bupivacaine hydrochloride combined with a low dose of meloxicam (HTX‐011) using extended‐release polymer technology was approved for use in humans in 2021 by the FDA, and in several other countries, including the European Union and United Kingdom (ZynrelefTM). It is instilled locally around the incision site after surgery to produce postoperative analgesia up to 72 h. Pre‐clinical studies in pigs showed the ability of meloxicam to reduce local tissue inflammation, thereby lessening the drop in tissue pH in the incision after surgery compared with control, which was associated with potentiated and prolonged analgesic activity of bupivacaine [320]. Local anesthetic drugs may cause CNS and cardiac toxicity at high plasma concentrations. Plasma concentrations are determined by the rate of drug absorption into the systemic circulation, but the most common reason for excessive plasma concentrations is the inadvertent direct intravascular injection of the local anesthetic solution while performing peripheral or neuraxial blocks. Local anesthetics vary considerably in their potency at causing systemic toxic reactions, and generally, this potency follows the same rank order as their potency at producing nerve blockade [321]. More lipid‐soluble drugs (i.e., bupivacaine) are more potent at causing systemic toxicity than less lipid‐soluble agents (i.e., lidocaine or mepivacaine), and the S(–)‐enantiomers (i.e., levobupivacaine or ropivacaine) are less toxic than the R(+)‐enantiomers, or than the racemic mixture of both [322–325]. The dose of a local anesthetic causing systemic toxicity will depend on the route and speed of administration (rapid intravenous administration will be more likely to cause high plasma levels), the species involved, and patient factors (such as acid–base balance, serum potassium levels, and whether the animal is under anesthesia). The clinical incidence of systemic toxicity in domestic animals is unknown. The incidence of systemic toxicity associated with regional anesthetic blocks in humans is estimated to be around 1 in 10,000, with peripheral blocks having the highest incidence (7.5 in 10,000) [326,327]. CNS toxic signs follow a progression as the plasma concentration of local anesthetic increases (Fig. 29.6). At low doses, all local anesthetics are effective anticonvulsants, and they also have sedative effects [328]. In conscious, unsedated humans, the initial signs of local anesthetic CNS toxicity include tongue numbness, light‐headedness, dizziness, drowsiness, paresthesia of sight and sound, and acute anxiety, or even fear of death [329]. In horses, this has been described as alteration in visual function, rapid eye blinking, anxiety, mild sedation, and ataxia [287,288,330]. As the plasma level rises, local anesthetics inhibit inhibitory cortical neurons in the temporal lobe or the amygdala, allowing facilitatory neurons to function in an unopposed fashion, resulting in increased excitatory activity, which first leads to muscle twitching followed by grand mal seizures [321,328]. As the plasma concentration increases further, local anesthetics can inhibit both inhibitory and facilitatory pathways, resulting in CNS depression, unconsciousness, and coma [321,328]. Figure 29.6 Progressive signs of lidocaine systemic toxicity with increasing plasma concentrations. Note that concentrations are approximate and depend on various factors (see text for details). Not all local anesthetics produce signs of aura, such as drowsiness or excitement, before the onset of seizures. With highly lipophilic, highly protein‐bound agents such as bupivacaine, the excitement phase can be brief and mild, and the first signs may be bradycardia, cyanosis, and unconsciousness [331]. CNS toxicity is generally assumed to precede cardiovascular toxicity. This derives from studies in conscious sheep where the doses and plasma drug concentrations associated with cardiovascular collapse (defined as the disappearance of pulsatile blood pressure) and CNS toxicity (signified by the onset of seizures) were calculated as the CV:CNS ratio. Values for the CV:CNS ratio for various local anesthetics in conscious sheep are much greater than 1, supporting this notion [323,332]. In humans, the frequency of seizures and accompanying cardiovascular changes associated with various regional block techniques have been reviewed. There is a significant difference between the rate of seizure development with caudal > brachial plexus > epidural blocks, with no adverse cardiovascular or pulmonary effects occurring during seizures [333]. In conscious dogs, the mean cumulative dose required for convulsive activity was 4 mg/kg for tetracaine, 5 mg/kg for bupivacaine, 8 mg/kg for etidocaine, and 22 mg/kg for lidocaine in one study [13]. In another study, administration of intravenous infusions of lidocaine (8 mg/kg/min), bupivacaine (2 mg/kg/min), or ropivacaine (2 mg/kg/min) caused generalized seizures at an average dose of 21 mg/kg of lidocaine, 4 mg/kg of bupivacaine, and 5 mg/kg of ropivacaine in conscious dogs [334]. The first seizure activity observed with lidocaine toxicity in dogs was tonic extension at an infused dose of 12 mg/kg, followed by running activity after 23 mg/kg, and with tonic‐clonic seizures occurring at an infused dose of 33 mg/kg [335]. The plasma concentration of lidocaine causing muscle tremors was 2.7 μg/mL after administration of a total dose of 11.1 mg/kg IV to conscious dogs [336]. The onset of seizures occurred when lidocaine plasma concentrations reached 8.2 μg/mL in another study involving awake dogs [337]. In conscious horses, the mean toxic plasma concentration of lidocaine causing muscle fasciculations was determined to be 3.24 μg/mL (range 1.85–4.53 μg/mL), and it did not change regardless of speed of administration [330]. Such plasma concentrations may be achieved during prolonged lidocaine IV infusions of greater than 12 h in postcolic surgery horses [338,339]. In lightly anesthetized and ventilated cats, seizures occurred after administration of 12 mg/kg of lidocaine and 5 mg/kg of bupivacaine given at intravenous infusion rates of 16 mg/kg/min and 4 mg/kg/min, respectively [340]. The onset of seizures occurred with lidocaine plasma concentrations of 19.6 μg/mL [341]. The CV:CNS toxicity ratio for drug dosage was 4.0 with lidocaine and 4.8 with bupivacaine in cats [340]. In conscious sheep, the doses of infused lidocaine, bupivacaine, and ropivacaine necessary to produce convulsions were 6.8 mg/kg, 1.6 mg/kg, and 3.5 mg/kg, respectively [342]. Therefore, the ratio of the mean convulsant doses (lidocaine/bupivacaine/ropivacaine) was approximately 5:1:2 [342]. There is an inverse relationship between the seizure threshold dose of local anesthetics and the arterial CO2 tension [343]. This may be due to an increase in cerebral blood flow during hypercapnia causing increased delivery of drug to the brain and/or a decrease in plasma protein binding of local anesthetics causing an increase in free drug [344]. Hypoxemia also increases the CNS and cardiovascular toxicity of local anesthetics [345]. At low concentrations, most local anesthetics have an antiarrhythmic effect, but at higher concentrations, they produce cardiac toxicity. Local anesthetics block cardiac Na+ channels and decrease the maximum rate of rise of Phase 0 of the action potential, leading to a pronounced and evolving inhibition of cardiac conduction [346]. Electrocardiographic changes include prolonged PR and QRS intervals and a prolonged refractory period [346–348]. The cardiovascular effects of local anesthetics are complex and non‐linear, including direct effects on cardiac conduction, cardiac contractility, and vascular smooth muscle, as well as indirect effects mediated by the CNS [321]. All local anesthetics cause myocardial depression with small intravenous doses that cause no overt CNS toxicity [325,349]. At subconvulsant doses, heart rate may increase slightly and the QRS complex may widen, but there are no major effects on blood pressure and cardiac output [325,349]. These effects are mild and rapidly reversed, with no qualitative differences among local anesthetics [325]. At the onset of convulsions, there is a profound sympathetic response associated with all local anesthetics, which reverses the induced myocardial depression causing tachycardia and increased blood pressure and cardiac output [324,325,342]. Convulsant doses of all longer‐acting local anesthetics cause marked arrhythmias, typically ventricular tachycardia, that may progress to ventricular fibrillation or cardiovascular collapse [324,350]. Supraconvulsant doses of lidocaine cause profound hypotension, bradycardia, decreases in myocardial contractility, respiratory arrest, and ultimately asystole [324,342]. It has been postulated that the CNS toxic effects may be involved in the production of serious cardiotoxicity because of the onset of respiratory failure accompanied by hypoxia, bradycardia, hypercapnia, and acidosis [328]. While all local anesthetics cause direct negative inotropic effects, the shorter‐acting local anesthetics such as lidocaine and mepivacaine are less arrhythmogenic than the longer‐acting ones, such as bupivacaine or ropivacaine [324,350]. These differences are caused by differences in the kinetics of binding and unbinding from various ion channels [351,352]. While both shorter‐ and longer‐acting agents have similar rates of binding to cardiac Na+ channels, the longer‐acting agents have slower unbinding rates, hence predisposing to cardiac arrhythmias [321]. The R(+)‐enantiomers of the more lipophilic local anesthetics have slower unbinding rates than the S(–)‐enantiomers, thereby making them even more arrhythmogenic [351,352]. No ventricular arrhythmias were observed with cardiotoxic doses of lidocaine in conscious dogs [324]. Ventricular tachycardia with no hemodynamic impairment was observed in only one of eight conscious sheep with lidocaine and one of seven with mepivacaine [350]. In contrast, ventricular arrhythmias occurred in one of six conscious dogs with cardiotoxic doses of ropivacaine and five of six with bupivacaine [324]. Polymorphic ventricular tachycardia accompanied by decreased cardiac output occurred in 7 of 10 conscious sheep receiving bupivacaine, 4 of 11 with levobupivacaine, and 5 of 12 with ropivacaine [350]. Even though the newer local anesthetics ropivacaine and levobupivacaine appear to be less cardiotoxic than bupivacaine (judging by the larger doses tolerated before the onset of serious arrhythmias), they must not be regarded as totally safe [353]. General anesthesia has a substantial impact on toxicity, mortality, and pharmacokinetics of various local anesthetics and distorts pharmacokinetic–pharmacodynamic relationships. In a study in halothane‐anesthetized sheep, the pre‐existing myocardial depression from halothane was markedly exacerbated by infusions of lidocaine, mepivacaine, prilocaine, bupivacaine, levobupivacaine, or ropivacaine [350]. The cardiovascular toxic effects of each local anesthetic were also prolonged in anesthetized sheep compared with conscious sheep, and concurrently, the blood drug concentrations were markedly increased under general anesthesia. However, no serious arrhythmias occurred in any anesthetized sheep. Despite the exaggerated cardiovascular effects of the local anesthetics when the sheep were anesthetized, none of them died, whereas approximately 15% died from fatal cardiac arrhythmias when conscious [350]. As the K+ gradient across cardiac myocyte membranes is the most important factor in establishing the membrane potential, hyperkalemia can markedly increase local anesthetic toxicity. Under conditions of hyperkalemia (5.4 mEq/L) in dogs, the cardiotoxic doses of both lidocaine and bupivacaine were halved compared to conditions of normokalemia, while the seizure‐inducing doses did not change for either agent [354]. Conversely, hypokalemia decreases local anesthetic cardiotoxicity [354]. When signs of systemic toxicity are noted, the administration of local anesthetic should be discontinued. Treatment of systemic toxicity is primarily supportive (Box 29.1). Oxygenation and ventilation are the main goals. It may be necessary to intubate the trachea and mechanically ventilate the animal to avoid or reverse hypoxemia, hypercapnia, and acidosis, all of which promote toxicity. If grand mal seizures are present, an anticonvulsant drug may be administered. If cardiovascular depression is also present, barbiturates or propofol are not recommended and treatment with a benzodiazepine is preferable.
29
Local Anesthetics
Introduction
Pharmacology
Molecular mechanism of action
Classification
Diameter (μm)
Myelin
Conduction (m/s)
Location
Function
Order of blockade
Aα
12–20
+
70–120
Afferent/efferent for muscles and joints
Afferent proprioception; efferent motor
5
Aβ
5–12
+
30–70
Efferent to muscle; afferent sensory nerve
Motor and sensory (touch and pressure)
4
Aγ
3–6
+
15–30
Efferent to muscle spindles
Muscle tone
3
Aδ
2–5
+
12–30
Afferent sensory nerve
Pain (fast), touch, temperature (cold)
2
B
< 3
+
3–15
Preganglionic sympathetic
Autonomic
1
C
0.4–1.2
–
0.5–2
Postganglionic sympathetic; afferent sensory nerve
Autonomic, pain (slow), temperature, some mechanoreceptors and reflex responses
2
Mechanism of blockade of neural tissue
Chemical structure
Physicochemical properties
Local anesthetic
pKaa
% Ionized (at pH 7.4)
Lipid solubilityb
% Protein binding
Relative anesthetic potencyc
Relative potency for CNS toxicityd
CV:CNS ratioe
Ester‐linked
Low potency, short duration
Procaine
8.89
97
100
6
1
0.3
3.7
Chloroprocaine
9.06
95
810
7
1
0.3
3.7
High potency, long duration
Tetracaine
8.38
93
5822
94
8
2
ND
Amide‐linked
Intermediate potency and duration
Lidocaine
7.77
76
366
64
2
1
7.1
Mepivacaine
7.72
61
130
77
2
1.4
7.1
Prilocaine
8.02
76
129
55
2
1.2
3.1
Intermediate potency, long duration
Ropivacaine
8.16
83
775
94
6
2.9
2
High potency, long duration
Bupivacaine
8.1
83
3420
95
8
4
2
Levobupivacaine
8.1
83
3420
> 97
8
2.9
2
Etidocaine
7.87
66
7317
94
6
2
4.4
Clinical pharmacology
Pharmacokinetics
Absorption
Distribution
Metabolism
Excretion
Factors affecting pharmacokinetics and activity
Additives
Epinephrine (adrenaline)
Phenylephrine and methoxamine
Phentolamine
Hyaluronidase
Sodium bicarbonate
Carbon dioxide
α 2‐Adrenergic receptor agonists
Mixtures of local anesthetics
Tachyphylaxis
Specific drugs and clinical uses
Aminoesters
Proparacaine (proxymetacaine)
Oxybuprocaine
Procaine
Benzocaine
Chloroprocaine
Tetracaine
Aminoamides
Lidocaine
Mepivacaine
Bupivacaine
t1/2 (minutes)
Vdss (L/kg)
Cl (mL/kg/min)
Dog [294]
68.1 ± 10.9
1.38 ± 0.08
27.5 ± 6
Cat [108]
100 ± 28
1.39 ± 0.37
26 ± 2.7
Horse [134]
79 ± 41
0.79 ± 0.16
29 ± 7.6
Cattle [113]
63.6 ± 42
3.3 ± 1.6
42.2 ± 20.5
Sheep [122]
30.9
NA
41.2
Levobupivacaine
Ropivacaine
Special formulations
Local anesthetic
Commercial preparations
Indications
Doses
Procaine
Maximum dose:
Lidocaine
Topical:
Injectable:
Maximum dose:
IV infusion dose:
Mepivacaine
Maximum dose:
Intra‐articular dose:
Bupivacaine
Topical:
Injectable:
Maximum dose:
Levobupivacaine
Chirocaine
Maximum dose:
Ropivacaine
Naropin
Maximum dose:
Lidocaine dermal patches and eutectic creams
Lidocaine–bupivacaine gel (Tri‐Solfen®)
Liposome‐encapsulated bupivacaine (Nocita®)
Dual‐acting bupivacaine–meloxicam extended‐release polymer
Adverse effects
Systemic toxicity
Central nervous system toxicity
Cardiovascular toxicity
Treatment of systemic toxicity
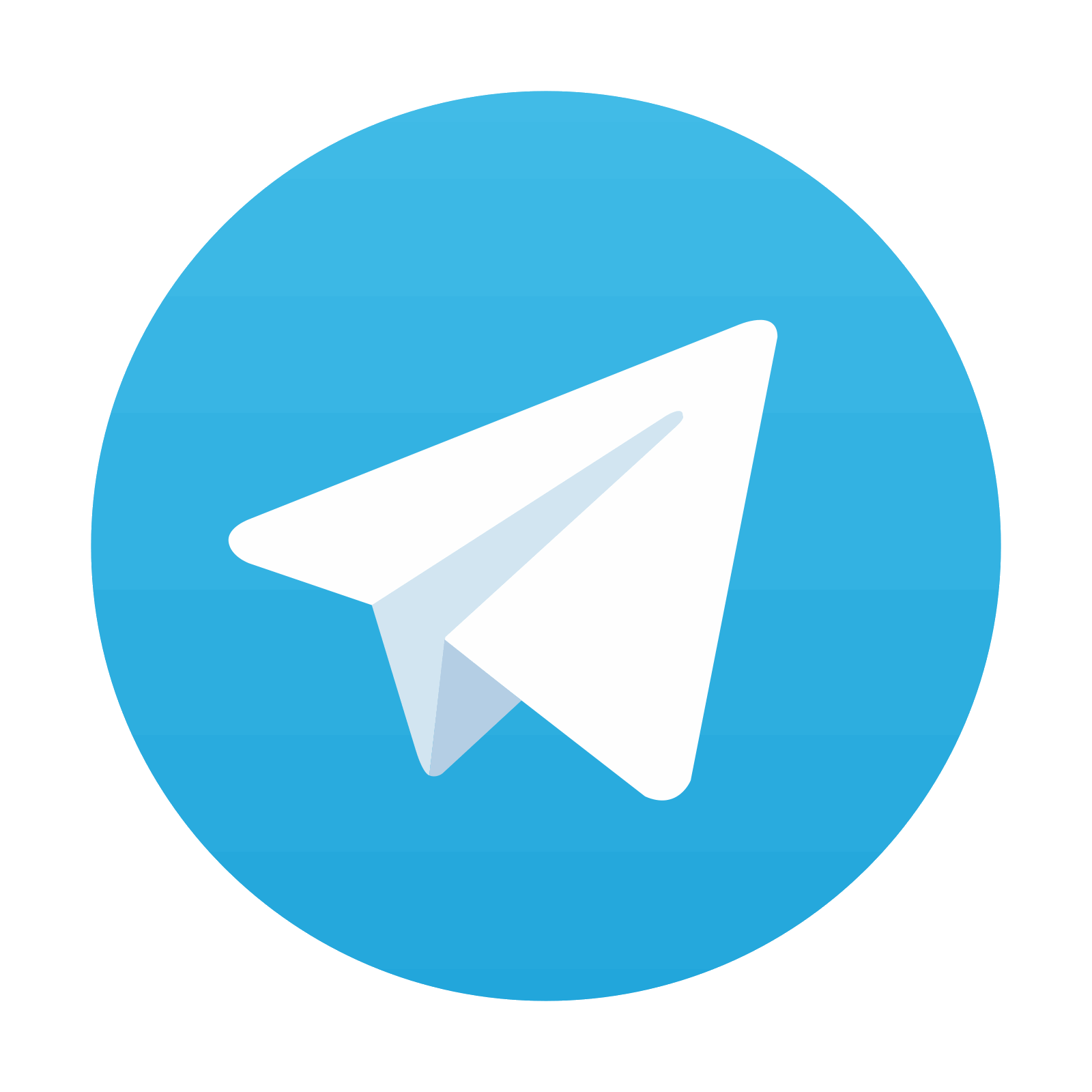
Stay updated, free articles. Join our Telegram channel
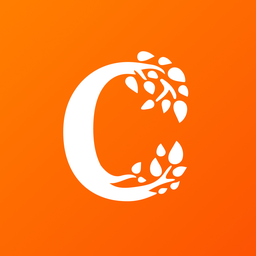
Full access? Get Clinical Tree
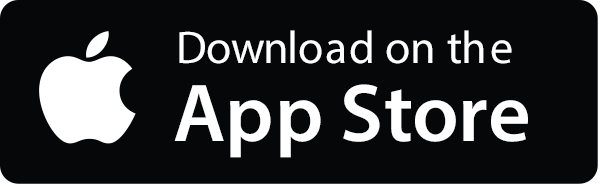
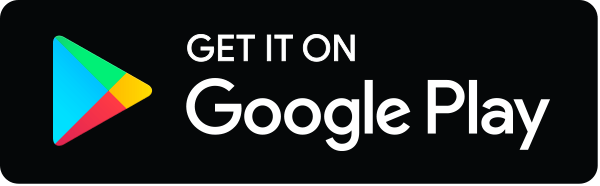