Allison Billings1, Jennifer K. Quinn2, and Melanie S. Spoor2 1 Veterinary Clinical Pathology, IDEXX Laboratories, Inc., Portland, OR, USA 2 Veterinary Clinical Pathology, IDEXX Laboratories, Inc., Wetherby, UK Most laboratory tests directed to the evaluation of muscle measure the serum activity of enzymes released from muscle tissue after injury. Extramuscular factors contributing to increases in serum enzyme activity should be considered when interpreting changes in these enzymes. Other constituents released from muscle such as myoglobin and troponin I are also useful biomarkers of muscle injury. The cardiac isoform of troponin I is currently a valuable clinical tool in the assessment of myocardial injury. The basis of clinical enzymology of muscle is the measurement of enzyme activities that occur when muscle tissue has been damaged. A range of enzymes and biomarkers are available for use in clinical investigations to monitor the onset or progress of muscle disease. The concentrations of enzymes in serum or plasma can be determined by using the chemical reactions they catalyze or by an immunoassay. The concentration of muscle enzymes in serum or plasma is usually low in healthy horses because the enzymes are located within the myofiber, with most present in the sarcoplasm. However, certain enzymes also have a mitochondrial form. In general, an increase in serum enzyme activity may be secondary to increased production of the enzyme (e.g., hyperplasia), increased release from damaged cells, or due to decreased removal of the enzyme from the blood. The major mechanism by which serum enzyme activity increases is through release from damaged myofibers at a rate that exceeds the rate of enzyme inactivation or removal from blood [1]. Cell necrosis and irreversible cell damage lead to release of enzymes and increased serum enzyme concentrations. Minor cell injury that causes reversible damage may also create increases in serum enzyme activities through formation of membrane blebs containing cytoplasmic enzymes. These blebs may rupture or be released into blood and lyze, producing an increase in serum enzyme activity [2]. The rate of enzyme loss (or its rate of rise in plasma) is affected by the severity of tissue damage, the enzyme concentration within the cell, the location of the enzyme within the cell, and how the enzyme enters the blood. Thus, the magnitude of increase does not depend only on the extent of muscle injury. The common muscle enzymes are cytoplasmic enzymes and their rate of increase is likely to be greater with more severe muscle damage. Because enzymes from myofibers are released into the interstitial space and enter the plasma via the lymphatics, there is a slower rate of enzyme increase than direct release into blood [3]. Aspartate aminotransferase (AST), formerly known as glutamic oxaloacetic transaminase (GOT), catalyzes the reversible transamination of L‐aspartate and 2‐oxoglutarate (alpha‐ketoglutarate) to oxaloacetate and glutamate and requires pyridoxal‐5′‐phosphate (PP) as a cofactor. The requirement of a cofactor is of note because assays including the cofactor may generate different results from those lacking the cofactor. Poor saturation of serum alanine aminotransferase (ALT) with endogenous PP caused underestimation of the total enzyme activity in a study of horses post exercise; however, the same study showed the majority of AST (94%) was saturated with endogenous PP and thus not subject to the same underestimation [4]. AST is reported to be stable for days in serum at room temperature, refrigerated, or frozen [5]. A study of equine clotted blood and serum stored at room temperature revealed significant increases in enzyme activity after 48 hours [6]. Results are reported in international units per liter (U/L). The half‐life for equine AST is generally reported to be 7–8 days [7]. However, other studies suggest a shorter half‐life of 3–4 days [7–9]. In either case, serum AST has a longer half‐life than creatine kinase (CK). Peak values in the horse are reached within 24–48 hours [9–11]. Aspartate aminotransferase is present within the cytoplasm and in the mitochondria. In horses, the ratio of cytoplasmic AST (cAST) to mitochondrial AST (mAST) is greater than in other mammals [4, 12]. It is postulated that with severe or irreversible cell injury, there may be a greater magnitude of serum AST increase due to the release of mAST as well as cAST. Studies to confirm this suspicion are lacking. In addition to skeletal muscle, AST is found within cardiac muscle cells, hepatocytes, and erythrocytes, therefore increases in serum AST activity can occur with myocyte injury, hepatocellular injury, and hemolysis [13]. Although an increase in AST activity may reflect some degree of myocyte injury, it does not specify a particular disease or disorder. Given the nonspecific nature of AST, assessment of additional organ‐specific enzymes is often required to distinguish between myocyte and hepatocellular injury. Serial measurement of AST in conjunction with CK can often be useful to indicate the time course of muscle injury. CK has a very short half‐life, increases very quickly (peaks at 6–12 hours), and remains increased for only a couple of days following an episode of muscle injury. Continued increases suggest ongoing or active muscle injury. In contrast, AST has a more gradual rise to peak activity and decreases more slowly due to its longer half‐life. It can be present for one to several weeks following an episode of muscle injury and thus may be increased with either persistent or resolved muscle injury. Therefore, serial measurements that initially show an increase in both AST and CK, and later show only an increased AST, indicate that the episode of myonecrosis has resolved (see Figure 10.1). Creatine kinase catalyzes the phosphorylation of creatine, utilizing ATP, to form phosphocreatine and adenosine diphosphate (ADP). In skeletal and cardiac myocytes, phosphocreatine serves as a reservoir for regeneration of ATP required for muscle contraction. CK is present in negligible amounts in many tissues throughout the body and in high concentrations within skeletal and cardiac muscle [14]. It is predominantly a cytoplasmic enzyme though a small amount is associated with the outer side of the inner mitochondrial membrane [15]. The enzyme is stable for seven days at 4 °C and one month at −20 °C in canine serum; published studies for refrigerated stored equine samples are lacking. Plasma collected from healthy foals and stored at −20 °C had a clinically insignificant decrease in CK activity after 12 weeks [16]. Equine samples (clotted blood and serum) stored at room temperature resulted in increases in CK after 72 hours [6]. Serum CK activity is higher than plasma CK activity in the dog due to release of CK with clot formation, but it is unknown if this is true in the horse [17]. Results are reported in international units per liter (U/L). Figure 10.1 Increases in serum CK and AST activities after muscle injury. CK has an earlier rise to peak and shorter half‐life than AST. Differences in CK and AST serum activities over time may be helpful in determining the time course of muscle injury. (a) After a single insult both CK and AST are initially increased. If measurements are repeated at day 3 following the insult, CK activity will likely be within or close to normal range while AST will still be increased. (b) Continued high levels of both CK and AST suggest repeated or ongoing muscle injury. The half‐life of CK is relatively short though specific times vary amongst studies. Intravenous and intramuscular injections of CK produce half‐lives of approximately two hours and 12 hours, respectively [18]. The intramuscular administration is expected to be more reflective of actual physiological conditions as CK must be absorbed from the lymphatics into the blood after muscle injury and release from the myocyte. In more recent studies in horses, CK was shown to peak from six to 12 hours [10]. Return of CK to baseline values were reported at 24 hours, 2–3 days, and 3–4 days in each of the studies. Creatine kinase has several isoenzymes. In most tissues, both cytoplasmic CK and mitochondrial CK isoenzymes are coexpressed. Cytoplasmic CK has a dimeric structure made of M (muscle) and B (brain) subunits. There are three cytosolic CK isoenzymes: CK‐1 (or CK‐BB) is found primarily in the brain and CK‐2 (CK‐MB) and CK‐3 (CK‐MM) are found in cardiac and skeletal muscle; CK‐2 is present in overall low concentrations in the horse [19, 20]. Although it is possible to separate these isoenzymes, CK isoenzyme analysis has not been shown to be diagnostically useful in equine studies [21]. The majority of serum CK is of muscle origin. Therefore, CK is considered a muscle‐specific marker and increases in serum CK activity are considered indicative of muscle injury. However, it is worthy of note that hemolysis can cause increases in measured CK, due to the release of adenylate kinase, glucose‐6‐phosphate, and ADP from erythrocytes which can interfere with the coupled reactions of CK assays [13, 22]. Adenosine monophosphate and diadenosine pentaphosphate may be used as inhibitors of adenylate kinase in CK assays [22]. As discussed above, serial measurements of AST and CK can be utilized to assess the time course of the muscle injury. Extremely high serum CK activity (>10 000 IU/L) often requires dilution by a technician running the assay. Dilution of the serum results in dilution of endogenous CK inhibitors, so serial dilution of a sample with high CK activity may result in progressively higher activity levels. Thus, while the true activity level is high, it may be spuriously increased as a result of multiple dilutions to the sample [23]. Lactate dehydrogenase (LDH) is a cytoplasmic enzyme that catalyzes the conversion of pyruvate to lactate at the end of anaerobic glycolysis. It is present in many tissues in the body and is therefore not specific to muscle. However, it is present in higher concentrations in skeletal and cardiac muscle, kidney, and liver [14]. In heparinized equine plasma samples, the enzyme is stable when stored at room temperature and −18 °C for seven days. However, storage of heparinized equine whole blood in the same conditions results in a marked increase in LDH activity [24]. In another study, storage of equine clotted blood and serum samples at room temperature produced increases in LDH activity after 24 hours [6]. Results are reported in international units per liter (U/L). The half‐life of LDH is reported to be seven days and peak concentrations are expected 24 hours after tissue injury [9]. More recent studies have produced different results for half‐life, with return to normal values in three days in one study and 10–21 days in another [9, 11]. Perhaps these variations are due in part to the different half‐lives of the LDH isoenzymes, which vary in concentration in any one tissue, as well as the variable half‐lives of the same isoenzyme in different tissues [25, 26]. Lactate dehydrogenase is not organ specific and is present in a variety of tissues. Increases in serum LDH activity in the horse can suggest skeletal muscle injury, cardiac muscle injury, or hepatocellular insult; however, in the absence of isoenzyme analysis, utilization of other enzymes more specific for muscle and liver is recommended for interpretation. Erythrocytes contain a greater concentration of LDH than plasma, so hemolysis can cause increases in LDH as well [13]. Alanine aminotransferase catalyzes the reversible transamination of L‐alanine and 2‐oxoglutarate (alpha‐ketoglutarate) to pyruvate and glutamate. This reaction requires PP as a cofactor. In horses, a considerable amount of serum ALT is reported to be in the apoenzyme form (inactive form that is not bound to the cofactor), which can generate an underestimation of the total serum ALT activity if PP is not added to the sample. In one study, the increase in serum ALT activity after the addition of PP ranged from an average of 27% in retired, non‐Thoroughbred, unexercised horses to 61% in resting Thoroughbred racehorses and 72% in postexercise Thoroughbred racehorses [4]. Alanine aminotransferase is a cytoplasmic and mitochondrial enzyme, though the mitochondrial form is present in much smaller amounts. ALT activity is found in several organs in the horse, including muscle and liver. Based on studies of bovine blood, ALT is stable at room temperature in plasma for four days and serum for two days, though reports for human blood suggest a shorter stability of 24 hours at room temperature (and up to seven days at 4 °C) [27, 28]. Plasma ALT half‐life in dogs is 2–3 days. Reports for values in the horse are lacking. Results are reported in international units per liter (U/L). Many consider ALT to be a muscle‐specific enzyme in the horse, as the liver has minimal ALT activity and is expected to contribute little to serum ALT activity [29]. Despite this specificity, it is often absent from large animal biochemical profiles, perhaps due to greater reliance on CK and AST for muscle injury assessment. Hemolysis in equine serum is reported to cause increases in ALT due to both spectral interference and the addition of ALT from erythrocytes [30]. The effect of exercise and training on serum muscle enzyme activity is difficult to determine. Results vary in the literature due to differences in protocols used by different studies (such as variations in exercise and training duration and intensity), fitness level of the study subjects, and the study interval. Overall, the majority of studies find minimal to no increases in muscle enzymes during exercise. These studies include protocols of primarily submaximal intensity or short duration exercise [4, 7, 31]. In some studies that did report increases in muscle enzyme activity, these increases were attributed to muscle injury. For example, in one study, three horses had gluteal muscle injury and four had subclinical muscle damage. These horses were also exercised on a treadmill at an incline, which may have predisposed to injury [32]. In contrast to these results, studies in horses performing maximal or longer duration (endurance) exercise found significant increases in serum muscle enzymes (CK) activities, though these studies reported either no increase in AST or inconclusive AST results [33, 34]. Although CK and AST increases may occur with exercise, values are often not clinically significant and still within normal limits. Generally, with exercise there is a 50% increase in enzyme activity. CK increases can be attributed to plasma volume changes and muscle leakage and AST increases can be attributed to plasma volume change [35]. Differences in CK and AST due to age and sex are inconsistent in the literature. One study of Thoroughbred mares between 2 and 4 years of age found no effects of age on resting CK or AST [36]. However, a separate study of 2‐ and 3‐year‐old Thoroughbreds in training revealed that fillies were more likely to have increased CK and AST than colts, and 2‐year‐olds tended to have higher AST than 3‐year‐olds. Thus, age was a factor for CK and both age and sex were factors for AST [37]. In addition, in another study, 2‐year‐old Thoroughbred fillies showed more marked fluctuations in AST and CK than 3‐year‐old Thoroughbred fillies and colts. No relationship was found between elevations in muscle enzymes and stage of the estrous cycle [38]. Some studies did find evidence that sex differences may be due to the effect of hormones such as progesterone and estradiol on CK and AST release [38, 39]. These findings suggest that age‐ and/or sex‐specific reference intervals may be necessary. In a study evaluating biochemical differences between pregnant and nonpregnant Lipizzaner mares, no significant differences were found in activities of AST, CK, or LDH between the two groups. However, AST activity was lower in late‐term pregnant mares compared to those in early or mid‐term pregnancy [40]. Incorrect venipuncture can result in injury to connective tissue surrounding the vein and/or adjacent musculature and subsequent release of CK which mixes with the sample, thus increasing the CK activity measured. CK can increase over 125% above those values obtained via normal/correct venipuncture, yielding false‐positive results [41]. Myoglobin is a heme‐containing, oxygen‐carrying monomer protein expressed in muscle fibers that may be a useful biomarker of muscle fiber injury. Myoglobin is released into circulation immediately after muscle damage and its concentration peaks shortly (5–30 minutes) after muscle injury [42]. Myoglobin is cleared from the circulation faster than CK. Studies of horses with recurrent rhabdomyolysis show return to normal levels by 24–72 hours after exercise, though a study in humans suggests longer increases (of up to 19 days) may be possible with endurance exercise [42, 43]. Because myoglobin is cleared so quickly from the circulation, measurement of myoglobin in the urine may be of diagnostic value and helpful in situations where blood sampling soon after muscle injury is not possible. Myoglobin causes discoloration of the urine to a reddish‐brown (port‐wine‐like) color (Figure 10.2). Pigmenturia can elicit suspicion for myoglobinuria; however, hematuria and hemoglobinuria must also be considered as possible differentials. Hematuria may be ruled out by sedimentation of erythrocytes after centrifugation of the urine sample for 30 seconds or by microscopic evaluation of the urine sample and detection of erythrocytes. In cases of hemoglobinuria, the plasma will be discolored as well (though it is more of a pink color), whereas myoglobin does not cause a change in plasma color because of its rapid clearance [44]. Figure 10.2 Urine sample with myoglobinuria. Unlike hemoglobinuria, a concurrent serum sample will be clear due to the rapid clearance of myoglobin from plasma. Laboratory methods to detect myoglobin include urine dipstick tests, ammonium sulfate, spectrophotometric assays, and immunoassays. Cautious use of urine dipstick tests is recommended as many urine dipstick tests do not distinguish between myoglobin, hemoglobin, and hematuria. Ammonium sulfate, when added to urine, should precipitate the hemoglobin but not the myoglobin. Hemoglobin precipitates at 80% saturation with ammonium sulfate, whereas myoglobin precipitates at 100% saturation. However, because precipitation depends on the pH, temperature, time, and other factors, ammonium sulfate precipitation can give false results and is generally considered unreliable [45]. Spectrophotometric analysis can differentiate hemoglobinuria from myoglobinuria but some investigators consider it less reliable due to the rapid degradation of myoglobin to the met‐myoglobin form (which changes the spectra). Immunoassays are the most sensitive and specific method for detection of myoglobin in both the blood and urine. Radial immunodiffusion, nephelometry/immunoturbidimetry, and radioimmunoassay have all been described. Radial immunodiffusion and nephelometric methods can detect very low concentrations of myoglobin in urine. Of particular concern in horses with myoglobinuria is the development of acute tubular necrosis with acute or delayed renal failure due to tubular damage from the excretion of myoglobin. This sequela to muscle injury occurs only in rare cases, and appears to occur in horses with concurrent systemic acidosis and dehydration [46]. The troponins are proteins that regulate skeletal and cardiac muscle contraction by making actin–myosin interactions sensitive to cytosolic calcium levels. The troponin complex is composed of three different proteins: troponin C (TnC), which binds calcium, troponin I (TnI), which has an inhibitory function, and troponin T (TnT), which attaches troponin to tropomyosin [47]. The cardiac myocyte contains specific isoforms (cTnI and cTnT), which are used as biomarkers for cardiac myocyte injury due to their specificity and are discussed further in the cardiac muscle section. Skeletal troponin I (sTnI) is a skeletal muscle‐specific protein that has been proposed as a marker for skeletal myocyte injury. Significant sequence dissimilarity (40%) exists between sTnI and cTnI isoforms in humans [48]. If this difference is similar in horses, development of a commercial assay to detect skeletal muscle troponin could be useful in assessing muscle‐specific injury. Studies in humans have shown the utility of sTnI to detect muscle injury and have even been successful in differentiating between slow‐ and fast‐twitch muscle isoforms [49]. The enzyme rises in parallel to CK and stays increased for 1–2 days. Limited data in rats also show promise for skeletal muscle troponin as a biomarker for skeletal muscle injury [50]. However, studies in horses are lacking thus far. Equine myopathies have many different etiopathogeneses (see Table 10.1). While many result in abnormal increases in laboratory biomarkers of muscle injury, some do not. Most show clinical signs attributable to myopathy that include abnormal function (e.g., tremors and fasciculations), muscle atrophy, and/or pain from muscle necrosis. Immune‐mediated myopathy in horses has been reported secondary to infection with or exposure to Streptococcus equi subsp. equi. Both development of a severe infarctive purpura hemorrhagica (IPH) and an acute, severe, rhabdomyolysis have been described secondary to infection with this bacterial agent [51, 52]. There are also reports of immune‐mediated myositis in horses with a different clinical course (i.e., muscle atrophy). Table 10.1 Equine myopathies. One study in horses with this type of immune‐mediated myositis revealed 39% had a history of exposure to S. equi or S. zooepidemicus within the three months prior to the onset of clinical signs; however, the remaining horses had no underlying trigger identified. The same study showed an overrepresentation of the quarter horse breed. A subsequent study identified an autosomal dominant missense mutation in the MYH1 gene encoding type 2X myosin heavy chain (substitution of glutamic acid for glycine at position 321), which is strongly associated with susceptibility to immune‐mediated myositis in quarter horses [53, 54]. There appears to be a bimodal age distribution, with horses younger than 8 and older than 17 years more likely to be affected. Clinical signs include rapid onset of muscle atrophy (with the epaxial and gluteal muscles most severely affected), lethargy, stiffness, weakness, and fever. CK and AST levels are often persistently increased (between 1000 and 10 000 U/L), though in some cases are normal. Histological findings are consistent with immune‐mediated myositis with cellular infiltrates of predominantly macrophages and lymphocytes [55]. Infarctive purpura hemorrhagica (IPH) has been reported as an uncommon sequela to infection or exposure to S. equi equi, Corynebacterium pseudotuberculosis, and vaccination against S. equi equi. Rare cases have occurred post infection with equine influenza virus, equine viral arteritis, equine herpesvirus type I, S. equi zooepidemicus, and Rhodococcus equi. However, around one‐third of cases have no identified underlying etiology. Young to middle‐aged horses are most commonly affected. Clinical signs often occur within 2–4 weeks after a respiratory infection. Most common signs include muscle swelling and edema of all the limbs, stiffness, lethargy, anorexia, hemorrhages on mucous membranes, and fever. Increases in CK ranging from 50 000 to 280 000 U/L and AST ranging from 1000 to 7000 U/L are common. Hematological changes include an inflammatory leukogram (leukocytosis characterized by a neutrophilia with left shift and toxic change), hyperfibrinogenemia, hyperglobulinemia, hypoalbuminemia, and abnormal clotting parameters. Histological findings show acute coagulative necrosis of affected tissue with leukocytoclastic (small vessel) vasculitis. Immune complexes in horses with IPH are composed of IgM or IgA and streptococcal M protein (SeM). These complexes result in deposition of complement in vessel walls, cell destruction, and vascular occlusion. Enzyme‐linked immunosorbent assay (ELISA) for detection of antibodies against SeM may be markedly increased (>1:1600) [51, 56, 57]. Rhabdomyolysis associated with Anaplasma phagocytophilum, an obligate intracellular gram‐negative bacterium, has been rarely reported in the literature. Clinical signs include fever, tachycardia, and stiffness. Increases in CK (>100 000 U/L) and AST (>20 000 U/L) are marked [58]. Microscopic blood smear evaluation may reveal morulae (or microcolonies) within neutrophils. Other methods of diagnosis include detection of antibodies via immunofluorescence assay (IFA), Western immunoblot (WIB), or ELISA, which require serial measurement and demonstration of an increasing titer, or detection of antigens in the blood via polymerase chain reaction (PCR) [59]. Clostridium perfringens and C. septicum are the most common species reported in association with myonecrosis in the horse; other less commonly implicated species include C. chauvoei, C. sordelli, C. novyi, and C. fallax. The organisms are large, gram‐positive, anaerobic bacteria that most commonly cause infection in horses by contamination of injection sites or puncture wounds. CK and AST may be mildly to moderately increased if sufficient muscle necrosis is present. Diagnosis may be by microscopic identification of organisms in affected tissue, anaerobic culture, or detection via a fluorescent antibody test [60, 61]. Clostridium botulinum can also cause myopathy in horses due to type C toxin produced by the gram‐positive anaerobic bacillus. The toxin may be ingested from contaminated feed, produced by ingested bacteria in the intestinal tract, or produced by the bacteria in wounds. Production of toxin within the intestines is the most common cause in foals, and usually occurs between 1 week and 6 months of age, while production in wounds is an uncommon cause in horses. The toxin cleaves SNARE proteins required for presynaptic vesicle exocytosis, prohibiting the release of acetylcholine and causing muscle weakness, tremors, and dysphagia. Signs often progress to generalized flaccid paralysis and recumbency. CK and AST are often normal but may eventually increase due to ischemic myopathy secondary to recumbency. Horses are extremely sensitive to the toxin and tests to detect and measure toxin levels may not be able to register such low amounts. Diagnosis can be based on analysis of stomach contents or feed but usually depends on clinical signs and elimination of other possible causes [62]. Equine grass sickness is a dysautonomia of unknown etiology, but may be a form of botulism in horses [63]. However, unlike botulism, equine grass sickness is associated with autonomic and enteric neurodegeneration and increased expression of SNARE proteins in neurons [64]. Corynebacterium pseudotuberculosis is a gram‐positive facultative intracellular pleomorphic bacterium. The bacteria may cause a diffuse infection of the limbs and internal or external abscesses. External abscesses are the most common presentation in the western United States. Intramuscular abscesses fall into this external abscess category and are commonly located in the pectoral region and along the ventral midline or abdomen. It is postulated that the bacteria enter the horse through skin abrasions and penetrating wounds. One study demonstrated that house flies may act as mechanical vectors of C. pseudotuberculosis in horses [65]. Infections usually occur in the fall and early winter. Both ELISA for detection of antibodies and PCR to identify bacteria isolated from abscesses have been reportedly used for the diagnosis of infection [61, 66]. Severe acute rhabdomyolysis is a rarely reported but often fatal complication of upper respiratory infections due to S. equi in the horse. Common signs of infection with this gram‐positive bacterium include myalgia, stiffness, stilted gait (especially in the pelvic limbs), severe swelling and pitting edema of epaxial and gluteal muscles, recumbency, and myoglobinuria. Clinicopathological findings include a leukocytosis characterized by a neutrophilia, hyperfibrinogenemia, and markedly increased CK (over 100 000 U/L) and AST. Diagnostic methods include detection of cocci in affected skeletal muscle, bacterial culture of affected tissues, measurement of serum antibody titers to S. equi myosin binding protein via ELISA, immunofluorescent staining of skeletal muscle for S. equi myosin‐binding protein, and S. equi PCR [52]. The exact mechanism of pathogenicity is unknown but may be due to cross‐reacting streptococcal antibodies that target skeletal muscle myosin, direct muscle invasion by the bacteria, bacteremia with exotoxin and protease production within skeletal muscle, or nonspecific T‐cell stimulation by streptococcal superantigens. Four S. equi superantigens have been identified that elicit immune responses similar to those in humans infected with Streptococcus pyogenes causing necrotizing myositis and toxic shock [52]. Infestation with the equine ear tick (Otobius megnini) is a rare cause of muscle tremors and muscle fasciculations in the horse. CK and AST may be mildly to moderately increased [67]. Sarcocystis spp. are a common incidental finding in equine skeletal and cardiac muscle and are assumed to be rarely associated with clinical disease. However, this assumption is challenged by sporadic case reports linking S. fayeri infection with muscle degeneration and necrosis and eosinophilic myositis in the horse. Presenting clinical signs in these cases included weight loss, weakness, ataxia, and dysphagia (if tongue or esophagus was involved) [68–70]. One study identified a higher prevalence and burden of sarcocysts in the muscle of horses with neuromuscular disease compared to clinically healthy horses [71]. Sarcocystis bertrami is the species affecting horses and donkeys in Europe [72]. Theileria equi and Babesia caballi affect horses in tropical, subtropical, and temperate regions and are transmitted by ixodid ticks. An inflammatory myopathy has been described in horses with chronic piroplasmosis [73]. Clinical features include anorexia, weight loss, muscle atrophy, and poor performance. Endomysial lymphocytic infiltrates and autoantibodies directed against muscle antigens detected in horses with chronic piroplasmosis support an immune‐mediated pathogenesis [73]. An outbreak of muscle stiffness in Thoroughbreds at a racing yard revealed a significant proportion of horses with antibody levels supportive of EHV‐1 infection as well as increases in CK and AST [74]. Equine influenza virus is reported as a rare cause of muscle degeneration in the horse. Clinical signs include upper respiratory infection and myalgia. Clinicopathological findings can include marked increases in muscle enzymes and myoglobinuria. The disease was rapidly progressive and fatal in all three of the reported cases in one study [68]. Accidents or falls can cause significant muscle damage, including diaphragmatic rupture in the horse. Neurological disease can also cause myopathy in the horse, particularly during seizure activity or prolonged recumbency. Illness or trauma that results in prolonged recumbency has the potential to cause ischemic myopathy and rhabdomyolysis. In the horse, the gluteal muscles are often affected in dorsal recumbency and in lateral recumbency muscles such as the triceps group tend to be affected [75]. Trauma to muscle can also occur from medical treatments including surgical incisions and manipulation, injection of medications or irritating substances, and placement of tight casts or bandages. In horses, trauma to specific muscles such as the gastrocnemius muscle can occur during exercise or while struggling to rise. Compartment syndrome and postanesthetic myopathy develop when accumulation of fluid (edema or hemorrhage) creates high pressure within the enclosed fascial space surrounding the muscle. This high pressure results in reduced capillary blood flow to the muscle and ischemic damage. Placement of tight external bandages or casts can also result in a reduction of the compartment size and similar ischemic injury. Compartment syndrome in horses undergoing anesthesia for surgical or nonsurgical procedures has also been reported and is referred to as postanesthetic myopathy. The most important contributing factors to development of inadequate muscle perfusion and myopathy are thought to include positioning (with the dependent muscles of recumbent horses often affected), increased intracompartmental muscle pressures, low arterial blood pressure, venous stasis, and a longer length of procedure [75–78]. Affected muscles may have increased intracompartmental pressures and increases in serum CK and AST are also reported [79]. Extreme exercise and overexertion may cause myopathy and rhabdomyolysis in the horse. These cases are considered sporadic, versus the recurrent or chronic exertional rhabdomyolysis discussed later in this chapter. Sporadic exertional rhabdomyolysis occurs most often in cases of extreme exercise, heavy training/exercise after a period of decreased intensity training, or exercise/training in adverse environmental conditions (such as extreme heat). Dietary imbalances such as high nonstructural carbohydrate and low forage content or inadequate selenium and vitamin E can also be contributing factors [54]. Common clinicopathological findings include hemoconcentration, lactic acidosis, electrolyte changes, and increases in muscle enzymes (AST, CK, and LDH). Hypochloremia, hypokalemia, and hypocalcemia may all be seen with heavy sweating. Hyponatremia and hypernatremia have both been reported. Sporadic episodes may be subclinical to severe. In more severe cases, moderate to marked increases in muscle enzymes (up to 100 000 times the upper reference limit) and myoglobinuria may be seen. Calcium, magnesium, and potassium depletion can all contribute to stasis of the gastrointestinal tract and related clinical signs. Damage to the kidneys from myoglobin and/or inadequate tissue perfusion can result in renal failure, although this sequela is rare [80]. Glycogen branching enzyme deficiency is a fatal, autosomal‐recessive disease of quarter horses and American Paint horses caused by a nonsense mutation of the glycogen branching enzyme I (GBE1) gene [81]. Approximately 8% of quarter horses and American Paint horses are carriers [82]. The enzyme is essential for the formation of alpha‐1,6 glycosidic linkages to form branched glycogen, thus the enzyme deficiency limits the number of nonreducing ends at which glycogen can be formed and degraded. Polysaccharide with largely alpha‐1,4 glycosidic linkages accumulates in skeletal and cardiac muscle, liver, and brain [81]. The inability to store and mobilize glycogen to maintain normal glucose homeostasis has catastrophic consequences. Affected foals may be aborted, stillborn, or weak at birth with contracted tendons. In surviving foals, rhabdomyolysis, hypoglycemic seizures, or cardiac failure occur and usually lead to euthanasia or death before 18 weeks of age [81]. Common laboratory findings include leukopenia, intermittent hypoglycemia, and moderate increases in CK, AST, and gamma‐glutamyl transferase (GGT). Analysis of peripheral blood or skeletal muscle for reduced activity of the enzyme or histopathological analysis of myocytes for detection of PAS‐positive and amylase‐resistant inclusions are supportive of the diagnosis. However, the most accurate method of diagnosis for carriers and affected foals is a DNA test performed on samples from pulled mane or tail hairs [83]. Hyperkalemic periodic paralysis (HYPP) is an autosomal‐dominant trait found in quarter horses, Appaloosas, American Paint horses, and quarter horse cross‐breeds. Approximately 4% of the quarter horse population carries the mutation [84]. It is caused by a missense point mutation in the skeletal muscle sodium channel gene (SCN4A). Affected horses are either homozygous or heterozygous for the disease. The HYPP mutation results in a lower threshold for membrane depolarization in skeletal muscle and the failure of a subpopulation of sodium channels to inactivate after depolarization. The result is an excessive influx of sodium ions and outflux of potassium ions that generates a persistent depolarization of the myocyte [85]. Affected horses exhibit intermittent muscle fasciculations in the face, neck, shoulders, and flanks beginning around 2–3 years of age [81]. Fasciculations may cease after 15–20 minutes but can progress to weakness and recumbency. Horses heterozygous for HYPP are often less severely affected, with less frequent episodes than their homozygous counterparts, indicating a codominant mode of inheritance [81, 86]. Respiratory stridor, respiratory distress, and dysphagia may be evident with possible obstruction of the upper airway due to pharyngeal collapse or laryngeal paralysis. Upper airway obstruction tends to be seen more frequently in horses homozygous for the trait [87].
10
Laboratory Markers of Muscle Injury
10.1 Laboratory Evaluation of Equine Muscle Disorders
10.1.1 General Causes of Increased Serum Enzymes
10.1.2 Serum Enzymes Detecting Muscle Injury
10.1.2.1 Aspartate Aminotransferase (AST)
10.1.2.2 Creatine Kinase
10.1.2.3 Lactate Dehydrogenase
10.1.2.4 Alanine Aminotransferase
10.1.3 Additional Factors Affecting CK and AST Enzyme Activity
10.1.3.1 Exercise and Training
10.1.3.2 Sex, Age, and Pregnancy
10.1.3.3 Venipuncture
10.1.4 Nonenzymatic Markers of Muscle Injury
10.1.4.1 Myoglobin
10.1.4.2 Troponin I
10.2 Equine Muscle Diseases
10.2.1 Immune‐Mediated Myopathies
10.2.1.1 Immune‐Mediated Myopathy with Muscle Atrophy
Immune‐mediated
Infectious
Traumatic
Inherited/congenital
Myopathy with muscle atrophy
Infarctive purpura hemorrhagica
Bacterial/rickettsial
Anaplasma phagocytophilum
Clostridial myositis
Clostridium botulinum
Corynebacterium
pseudotuberculosis
Streptococcus equi
arasitic
Otobius megnini
Sarcocystis spp.
Theileria equi
Babesia caballi
Viral
Equine herpesvirus I
Equine influenza virus 2
Compartment syndrome
Postanesthetic myopathy
Extreme exercise
Glycogen branching enzyme deficiency
Hyperkalemic periodic paralysis
Malignant hyperthermia
Myotonia
Polysaccharide storage myopathy
Myofibrillar myopathy
Recurrent exertional rhabdomyolysis
Lipid storage myopathy
Centronuclear myopathy
Toxic
Nutritional
Unknown
Other
Hypoglycin A
Blister beetle
Vit E/selenium deficiency
Acquired motor neuron disease
Pituitary pars intermedia dysfunction
Thiaminase‐containing plants
Aortic iliac thrombosis
Senna occidentalis, obtusifolia
Systemic calcinosis
Ionophores
Ageratina altissima
Malva parviflora
10.2.1.2 Infarctive Purpura Hemorrhagica
10.2.2 Infectious Myopathies
10.2.2.1 Bacterial
cssStyle=”font-weight:bold;font-style:italic;” 10.2.2.1.1 Anaplasma phagocytophilum
cssStyle=”font-weight:bold;font-style:italic;” 10.2.2.1.2 Clostridial Myositis
cssStyle=”font-weight:bold;font-style:italic;” 10.2.2.1.3 Clostridium botulinum (Botulism)
cssStyle=”font-weight:bold;font-style:italic;” 10.2.2.1.4 Corynebacterium pseudotuberculosis (Pigeon Fever)
cssStyle=”font-weight:bold;font-style:italic;” 10.2.2.1.5 Streptococcus equi
10.2.2.2 Parasitic
cssStyle=”font-weight:bold;font-style:italic;” 10.2.2.2.1 Otobius megnini (Equine Ear Tick)
cssStyle=”font-weight:bold;font-style:italic;” 10.2.2.2.2 Sarcocystis spp.
cssStyle=”font-weight:bold;font-style:italic;” 10.2.2.2.3 Piroplasmosis
10.2.2.3 Viral
cssStyle=”font-weight:bold;font-style:italic;” 10.2.2.3.1 Equine Herpesvirus 1 (EHV‐1)
cssStyle=”font-weight:bold;font-style:italic;” 10.2.2.3.2 Equine Influenza Virus A2
10.2.3 Traumatic Myopathies
10.2.3.1 Compartment Syndrome and Postanesthetic Myopathy
10.2.3.2 Extreme Exercise/Overexertion
10.2.4 Inherited or Congenital Myopathies
10.2.4.1 Glycogen Branching Enzyme Deficiency
10.2.4.2 Hyperkalemic Periodic Paralysis
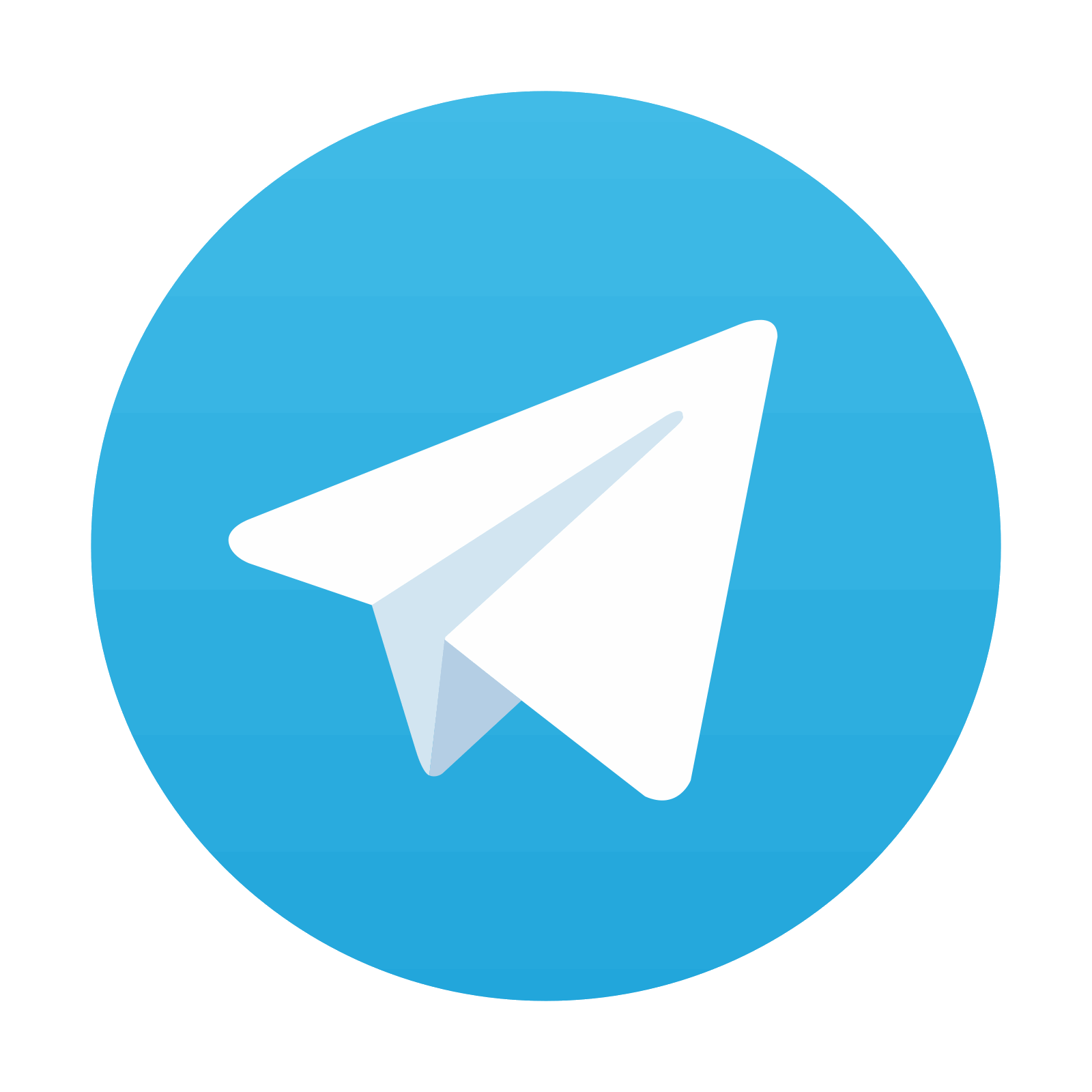
Stay updated, free articles. Join our Telegram channel
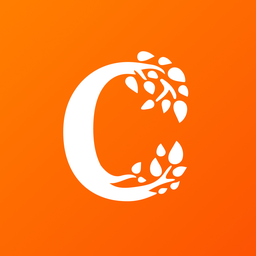
Full access? Get Clinical Tree
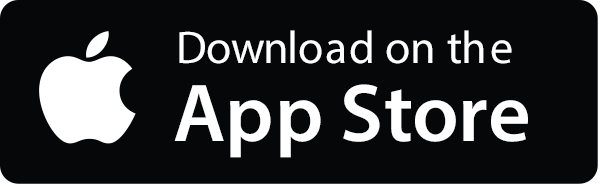
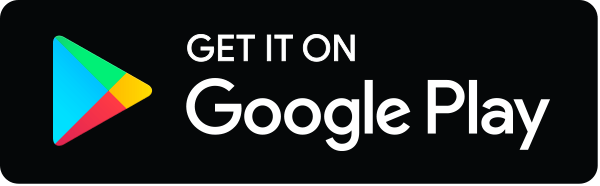