Fig. 1.
Structure of voltage-gated ion channels. As example for a structure of a voltage-gated ion channel, pore-forming α-subunit of voltage-gated Ca2+ channels is shown. The α1-subunit contains of Ca2+ channels contain four homologous domains (I–IV) with each six membrane-spanning α-helices (S1–S6). The carboxy- and aminoterminus as well as the loops connecting the domains are situated on the cytoplamatic site of the plasma membrane.
Table 1
Summary of the pore-forming subunits of voltage-gated calcium channels
Type | Cav name | Old name | Chromosomal localiszation | Exemplary characteristic inhibitors |
---|---|---|---|---|
High voltage-activated calcium channels (HVA) | ||||
Ca v subfamily | ||||
L L L L | Cav 1.1 Cav 1.2 Cav 1.3 Cav 1.4 | α1S α1C α1D α1F | 1q31–q32 12p13.3 3p14.3 Xp11.23s | L-type Ca2+ channel blockers, such as 1,4-dihydropyridines (e.g., nifedipin), phenylalkylamine (e.g., verapamil) or benzothiazepines (e.g., diltiazem) |
Ca v subfamily | ||||
P/Q N E/R | Cav 2.1 Cav 2.2 Cav 2.3 | α 1A α 1B α 1E | 19p13.1–q13.2 9q34 1q25–q31 | ω-Aga-IVA ω-CgTx-GVIA SNX-482 |
Low voltage-activated calcium channels (LVA) | ||||
T T T | Cav 3.1 Cav 3.2 Cav 3.3 | a1G a1H a1I | 17q22 16p13.3 22q12.3–q13.2 | a |
1.3 Aim of This Chapter
Molecular-biological studies support a central role of ion channels at the genesis of cerebral vasospasm following SAH, but how they are involved remains complex and incomprehensive. Evaluation of voltage-gated ion channels, especially of the role of voltage-gated calcium channels, is critical for our understanding of the molecular mechanisms underlying SAH-related cerebral vasospasm and includes all forms of ion channel assessment. Here, important implications of ion channel assessment should be discussed against the background of the recent studies as well as the what, why, where, when, and how to examine changes in ion channel expression and function after SAH. At some point, principles of Ca2+ channel assessment exemplary should be pointed for ion channel in general.
2 Materials and Instruments
Material and instruments required for ion channel assessment depends on the used procedures. However, usually standard laboratory equipment, such as PCR-cycler, centrifuges, etc., is needed. This standard equipment can be supplemented by special equipment. Furthermore, for various methods commercial kits are available which usually provide a comparatively easy way to perform a particular method—especially for beginners.
3 Procedures
Assessment of the role of ion channels during SAH-mediated vasospasm includes the wide-spread spectrum of molecular-biological, electrophysiological, immunochemical, and functional methods evaluating ion channel expression and function as well as the mediated currents. Standard molecular biological assessment of expression of ion channels and their tissue distribution in healthy and vasospastic cerebral vessels includes the evaluation of ion channel transcripts [e.g., by reverse transcription-polymerase chain reaction (RT-PCR)], confirmation of proteins (usually by SDS–PAGE and subsequent Western-blot analysis) or analysis of channel distribution by immunohistological staining. The mediated currents can be measured as whole-cell recordings as well as single-cell recordings by patch clamp analysis.
3.1 When Should Ion Channel Assessment Be Performed?
For success of the analysis, definition of several parameters is important: probably, changes in calcium channel function and expression occur at different time points reflecting a dynamic process of induction of cerebral vasospasm after aneurysmal SAH. Therefore, definition of the time range, respectively, the time points of ion channel analysis seems to be critical. The time course varies among different animal models of SAH-induced cerebral vasospasm probably due to species-related differences in the clot clearance and structural differences in the vascular and perivascular elements (14–19). In various animal models, delayed cerebral vasospasm could be distinguished from direct acute effects of oxy-hemoglobin on voltage-gated ion channels. Acute effects were suggested to depend on suppression of voltage-gated K+ channel currents, while delayed vasospasm involves also expression of voltage-gated Ca2+ channels (20). At best, ion channel assessment should reflect changes in channel expression and function over time with direct effects, like changed electrophysiological properties, a putative different behavior toward interaction partners or a putative enhancement or suppression by certain agents, and chronic effect depending on long-lasting changes or on alteration of channel expression.
3.2 Ion Channel Expression in Healthy Vessels
For assessment of changes in ion channels expression, knowledge about ion channel expression in healthy vessels is crucial. Only few studies addressed ion channel expression in cerebral arteries: Transcripts of the voltage-gated K+ channel subunit Kv1.1–Kv1.6, Kv2.1, and Kv2.2 were detected in healthy rat cerebral vessels but only the Kv1.2, Kv1.5, and Kv2.1 subunits were found to be expressed (21, 22). Furthermore, ATP-sensitive K+ channels (among other: Kir6.1/SUR2B KATP channels, reviewed in refs. (23, 24)), large conductance Ca2+-activated K+ channels (BKCa) and inwardly rectifying K+ channels (Kir) were proven (25, 26). For voltage-gated Ca2+ channels, L-type Ca2+ channel were traditionally believed to control calcium influx in myocytes of cerebral arteries, but two recent studies showed expression of various isoforms. In basilar myocytes of healthy dogs, Nikitina et al. (27) identified besides the L-type subunits Cav1.2 and Cav1.3 also the Cav2.2 subunit (N-type calcium channel) and the T-type subunits Cav3.1 and Cav3.3. In rat basilar arteries, the Cav1.2, an alternative splice variant of Cav1.2 containing exon 9*, Cav1.3, Cav3.1, and Cav3.2 subunit were found to be strongly expressed besides a weak expression of the Cav2.3 subunit (28).
3.3 Changes in Ion Channel Expression Following SAH
In response of SAH, voltage-gated K+ channel (Kv) are functionally impaired and expression of the Kv1.5 and Kv2 channels and Kv-mediated currents are markedly reduced (4, 5, 19, 29, 30). While expression of large conductance Ca2+-activated K+ channels (BKca) were unchanged after SAH, expression of Kir channels (Kir 2.1 subunit) was found to be increased which might be a compensatory mechanism (29, 31). Changes of Ca2+ channel expression in response to SAH occur later and have only partially been subjected. The recent studies analyzing expression of Ca2+ channels during vasospasm only carried out a molecular analysis for the Cav1.2 (L-type calcium channel) and the Cav2.3 subunit (E-/R-type calcium channels) in cerebral arteries (32, 33). In a rabbit model, cerebral arteries of healthy animals solely express Cav1.2 Ca2+ channels. Here, Ca2+ influx after pressure-induced vasospasm depends on L-type Ca2+ channels and spasm could completely reversed by the L-type Ca2+ channel blocker diltiazem (32). In contrast after SAH, oxy-hemoglobin induces an upregulation of the Cav2.3 subunit and a downregulation of Cav1.2 in small arterial vessels and cytoplasmatic Ca2+ influx is mediated by E-/R-type Ca2+ channels in up to 20%. These “resistant” Ca2+ currents were not assessable for L-type Ca2+ channel blockers (33). Furthermore, oxy-hemoglobin leads to a reduced sensitivity of L-type Ca2+ channels for diltiazem (33). Here, further studies evaluating expression and associated currents of all voltage-gated Ca2+ channels in arterial myocytes are needed.
3.4 Other Factors Influencing Ion Channel Expression
Several factors besides subarachnoid blood have great influence on ion channel expression and function and should therefore be considered. Here, a fussy definition and declaration of the general condition is crucial. Ion channel regulation is highly dynamically regulated during maturation or in response of other external influences (4, 34–39). Also yet not been studied for myocytes, several examples have documented developmental changes of Ca2+ channels, for example, in the calyx of Held, where transmission at early developmental stages is mediated by a cooperative action of spatially intermingled N-, P/Q-, and probably also R-type Ca2+ channels and N- and R-type Ca2+ channel are replaced by P/Q-type calcium channels during maturation (34, 35). Similar changes occur also in aging brains (40) and developmental changes have also been documented for K+ channels (41). Here, a study evaluating possible differences in ion channel expression after SAH at different points of maturation might be interesting. Furthermore, expression and function of ion channels are regulated by several other parameters, like in response of several hormones, pain, etc. (42–45). During planning of studies addressing ion channel expression, function or analysis of corresponding currents, those parameters influencing channel expression and function, like age of animals, should be considered and defined.
3.5 Where Should Ion Channel Assessment Be Performed?
Standard analysis of ion channel expression and of their mediated currents focused yet on cerebral vessel myocytes. Assessment of ion channel expression and dysfunction might also include other vascular layers. Oxy-hemoglobin-induced apoptotic changes in cultured vascular endothelial cells have been described; also they could not be prevented by standard Ca2+ channel blockers (46). Furthermore, evaluation of alterations of ion channel expression and function in the cerebral cortex in response to SAH might be interesting and has yet not been investigated. One might speculate, if subarachnoid bleed and its break-down products impair neurological transmission. For hippocampal CA1 neurons, such an influence on neuronal transmission has not been found for hemoglobin itself but for its degradation products ferrous chloride and hemin which produce an irreversible depression of field excitatory postsynaptic potential (47).
3.6 Functional Assessment of Ion Channels by Specific Blockade of Particular Ion Channels
Besides analysis of ion channel expression, distribution and their mediated currents, evaluation of their physiological and pathophysiological role is out of outstanding interest. Use of inhibitors enables specific blockade of particular ion channels in the plasma membrane and a subsequent analysis of their function and comparison to controls. Ion channels inhibitors should carefully be selected due to their inhibition pattern to prevent unwanted side effects. Furthermore, dosage and corresponding ED50 values should be considered. Importantly, nearly all ion channel inhibitors show unspecific side reactions in high concentrations, like the specific blocker of E-/R-type Ca2+ channels inhibiting L-type Ca2+ channels in high concentration over 200 nM.
Effects of ion channels, respectively, their blockage could be monitored in different ways and depend on the analyzed question. Often, functional analysis focuses on quantification of the contribution of ion channels due to cerebral vasospasm. Degree of vasospasm can be analyzed histopathologically, in either in vivo or in vitro models like isometric tension recordings or in vivo models for example by imaging methods like the traditional X-ray angiography or Doppler-Ultrasound measurement. These chapters were considered to discuss vasospasm assessments (Chap. 47), neuroimaging assessments, X-ray and angiographic assessments and MRI assessments of cerebral vasospasms (Chaps 58–60). But functional analysis includes also other methods, like evaluation of mediated currents or visualization of ion channel influx or efflux. Especially for voltage-gated Ca2+ channels, calcium influx can be visualized be specific dyes. Blockade of influx from the extracellular space allows also assessment of cytoplasmatic calcium influx from intracellular stores. Also these methods are presented elsewhere (Chap. 56)
3.7 Physiological Role of Ion Channels in Cerebral Vessels and Their Pathophysiological Role During Vasospasm
The physiological role of ion channels in cerebral vessels and in particular their pathophysiological role during SAH-induced vasospasm has not completely been elucidated and remains partially unclear. Previous studies of the physiological role of potassium channels revealed that they are involved in the maintenance of the resting membrane potential of vascular myocytes, that they are involved in limiting depolarization of the membrane potential by repolarization through K+ efflux and thus regulate diameter of cerebral arteries (6, 21, 22). KATP channels have shown to be involved in vasodilatation, reactive hyperemia in cerebral circulation, and cerebral autoregulation while their inhibition leads to vasoconstrictions (reviewed in ref. (6)). The role of Ca2+ channels remains unclear: Navarro-Gonzalez et al. (28) suggested that L-type Ca2+ channels were responsible for vasomotion, whereas non-L-type Ca2+ channel control the vascular tone. In contrast, an alternative splice variant of Cav1.2 containing exon 9* was found to be mainly involved in K+-induced arterial constriction (48). After SAH, decrease and dysfunction of Kv channels were mentioned to contribute partially to depolarization and to have a smaller contribution toward maintenance of the membrane potential. Therefore, they may be involved in the genesis and maintenance of cerebral vasospasm, also their role remains controversial (5). While BKCa channels are unchanged after SAH, Kir channels might compensatorily be upregulated (29, 31). Voltage-gated K+ channels were suggested to trigger acute effects, while expression changes of voltage-gated Ca2+ channels occur during delayed vasospasm (20). However, the role of expression changes of voltage-gated Ca2+ channels is yet not understood.
3.8 SAH-Knockout Models
Besides these methods, usage of an SAH-knockout model is a possibility for an evaluation of the physiological and pathophysiological role of a particular ion channel. Such an analysis has recently been performed for the role of adenosine A receptors in early ischemic vascular injury after SAH (49). Comparison of wild type and gene-manipulated animals might reveal striking differences and subsequently might give insights in ion channel function. Meanwhile, Ca2+ channel-deficient mice have been established for nearly all pore-forming subunits, but to our knowledge never used in SAH models. In part, results from experiments of ion channel deficient animals are hard to interpret. One should consider that loss of a particular ion channel usually leads to partial compensation by others. As an example, such a compensatory upregulation R-type and N-type Ca2+ channel occurs at the neuromuscular endplate of Cav1.2-deficient mice (36, 37).
3.9 Analysis of the Role of Ion Channel Interaction Partners
Ion channels are embedded in the functional or pathophysiologically disturbed network of the cell. Therefore, assessment has not only to focus on particular ion channels but also on their role in this network. The evaluation is important for understanding the mechanisms following SAH-induced cerebral vasospasm. Here, interaction partner plays an important role. Several regulatory proteins underlie molecular changes following SAH. As one example, protein kinase C (PKC) is mainly involved in the pathogenesis of cerebral vasospasms following SAH with an activation of the PKC-dependent contractile system (50, 51). Hemoglobin changes levels of PKC expression and different isoforms of the protein kinase are translocated from the cytosol to the plasma membrane after SAH (PKC-δ on day 4 and PKC-α on day 7) (52). Therefore, it was considered that PKC-δ is involved in initiation of SAH-induced vasospasm, whereas PKC-α plays a role in its maintenance (53, 54). As a classical target of modulation, PKC phosphorylates the Cav1.2 subunit of L-type calcium channels and leads to dual modulation with inhibitory and stimulatory effects in vascular smooth muscle cells. Further, PKC is involved in the Ca2+-dependent stimulation of Cav2.3. Interestingly, calmodulin, another regulatory protein of voltage-gated Ca2+ channels, was considered to decrease within 48 h after SAH in a canine model (55). One might speculate, if imbalance of calmodulin-mediated inactivation and PKC-mediated Ca2+-dependent stimulation of R-type calcium channels might lead to self-perpetuating Ca2+ influx during vasospasm. Therefore, further studies should also address interaction partners of voltage-gated calcium channels and elucidate their role within the cellular network.
< div class='tao-gold-member'>
Only gold members can continue reading. Log In or Register a > to continue
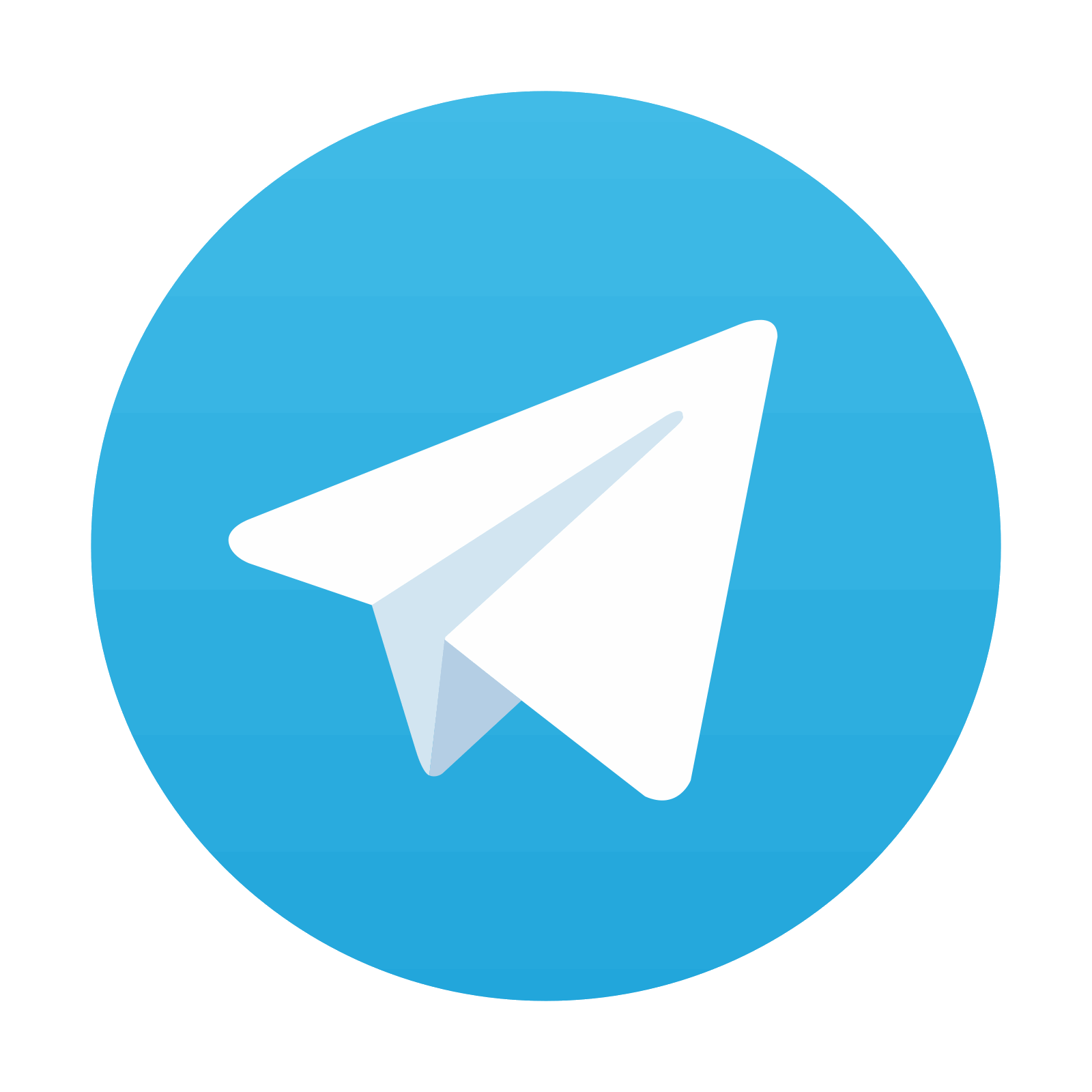
Stay updated, free articles. Join our Telegram channel
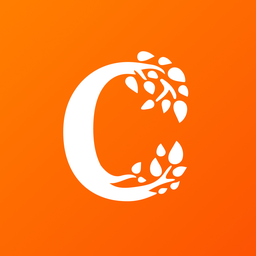
Full access? Get Clinical Tree
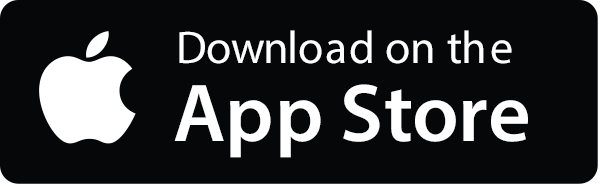
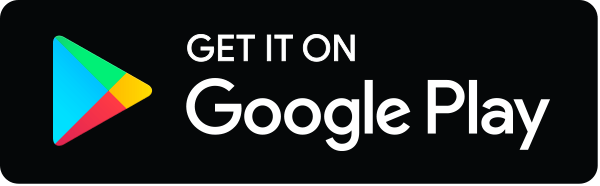