Fig. 1.1
Double plot actogram of locomotor activity in a rat kept under controlled environmental conditions. From day 1 to 5, white light (250 lux) was on at 07:00 h and off at 19:00 h. Dim red light (4 lux) was continuously on (LD). On day 6, white light was kept off until the end of the recording (DD). Each line is a histogram of 5 min bins of activity of two consecutive days; the 2nd day of each row is plotted again in the next row. During the first 5 days, the locomotion rhythm is entrained to the LD cycle (red); activity increases when white light goes off and decreases shortly before lights are on. On day 6, the rhythm starts to free run (blue) with a period of approximately 24:30 h. Room temperature was kept constant at 22 °C (±1°)
1.1.2 Entrainment
In natural conditions, circadian rhythms are aligned with the light–dark cycle, other external cycle’s secondary to the earth’s rotation or periodic events such as food availability and biological or social cues. The adaptive nature of the circadian rhythms depends on entrainment, because it allows the organism to align the biological and geophysical times. Entrainment, also known as synchronization, refers to the processes to match the period of the circadian rhythm to the period of the external cycle (Fig. 1.1) and to establish a constant time relation on a particular event of the circadian rhythm (such as awakening) and a specific event of the environmental cycle (such as dawn or dusk). Thus, when entrained, a particular phase of the circadian rhythm is locked to a particular phase of the external cycle, and both rhythms have equal periods (Pittendrigh 1993). When entrainment is studied under light–dark cycles, light has shown to speed up the clock(s), thus shortening the period of rhythmicity; in turn, during darkness, the clock(s) slows down, thus lengthening the period, so the complete cycle has an average of 24 h (Daan and Pittendrigh 1976b).
1.1.2.1 The Phase Response Curve
Entrainment has also been studied by applying discrete light pulses (minutes to hours) at different times of the circadian cycle to animals in constant conditions and measuring the response of the free-running rhythm, which is known as a phase response curve (PRC). This approach has shown that the same stimulus applied at different times of a circadian cycle has different effects on the phase (see Box 1.1 for definitions) of the rhythm due to the dynamical response of the underlying clock(s) (Pittendrigh 1984; Daan and Pittendrigh 1976a). The PRC has three characteristic zones (Fig. 1.2) in different species: (1) the dead zone occurs during the subjective day and is characterized by the lack of response to light, therefore the phase of activity observed after the light pulse occurs at the time it was expected from projecting the phase before the light pulse; (2) the delay zone occurs early during the subjective night and is characterized by phase delays, that is, when activity after the light pulse occurs later than expected; and (3) the advance zone occurs late during the subjective night and is characterized by phase advances, when activity after the light pulse occurs earlier than expected from projecting the phase before the light pulse. Phase shifts reach a steady state after some transitory cycles and maintain the new phase until perturbed by another phase-shifting stimulus (Pittendrigh 1984). Phase shifts are measured (in minutes or hours) by subtracting the phase of the rhythm before the stimulus to the phase in steady state after the stimulus. It is worth noticing that although day and night are absent in constant free-running conditions, we can still discriminate a subjective day and a subjective night, which depends on the circadian organization of the species under study, either active during the day or during the night. Moreover, the length of a free-running rhythm differs from the period of the entrained rhythm (24 h in natural conditions); therefore, each hour of the free-running day will also differ from 60 min. To estimate the circadian time (CT), the free-running period is divided in 24 segments or circadian hours. The PRC depicts in the abscissa the CT at which the light pulse is applied, while the ordinate depicts the magnitude of the induced phase shift, usually delays as negative values and advances as positive ones. The analysis of the PRC provides information about the dynamics of the circadian clock(s) which generates overt rhythmicity in each of the species studied, and nowadays is a valuable tool to unravel the cellular and molecular mechanisms involved in the functioning of the clock(s).
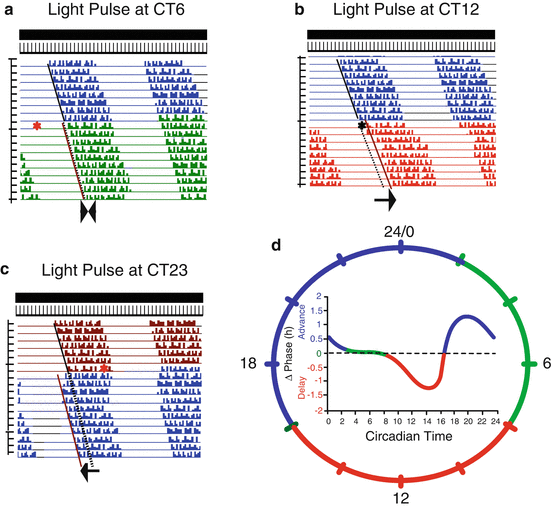
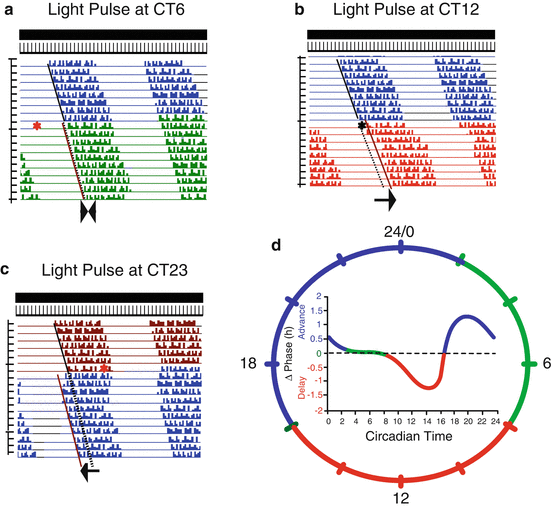
Fig. 1.2
Diagram of the phase response curve (PRC) to light pulses for a nocturnal rodent. The schematic actograms illustrate the phase responses to light pulses applied at different circadian times as indicated. The basal free-run recording is shown in blue (a, b) dark red (c); the effect on rhythmicity of a light pulse (red asterisk) at different circadian times (CT) is shown in green (at CT6), red (at CT12), or blue (at CT 23). The arrows below each actogram indicate the change on the activity onset after the light pulse (dotted lines) with respect to the recordings before the light pulse was applied (continuous line). The PRC is shown inside the 24 h dial; each color on the curve and the dial corresponds to the characteristic regions of the PRC: dead zone (green), the delay zone (red), and the advance zone (blue)
Box 1.1 Parameters Related to Rhythmic Phenomena
The period of a cyclic phenomenon refers to time lapse for a complete cycle to occur; in natural conditions, the period of the circadian rhythmicity is 24 h (but see also Sect. 1.1.1). The amplitude refers to the change in the intensity of the oscillatory variable from its highest value (peak) to its lowest (trough); in the context of the cosinor analysis (which is used to fit cyclic phenomena to a cosine function), it also may refer to the change in the intensity of the variable from the mean value of the variable throughout the cycle (mesor) to the highest value of the best fitting cycle (acrophase). Finally, the phase of the rhythm refers to the time at which any particular value of the cyclic variable occurs, for example, the time of waking or sleep initiates correspond each to a particular phase of the sleep–wake cycle. Also the time at which the peak or acrophase occurs is also a clear-cut phase reference for cyclic or rhythmic phenomena. Finally, since each rhythmic variable has specific phase descriptors, the time relation between two variables is best referred to as phase relation ; for example, the relation between waking and breakfast in a particular subject could be described as -1 h relation of breakfast with respect to awakening (a delay of 1 h after waking to have breakfast).
1.1.2.2 Masking
Complete cycles of light–dark or high–low temperature may also affect the behavioral expression of rhythmicity beyond the clock itself, therefore masking its phase and period; such effects of external factors on the expression of rhythmic behaviors are referred to as masking effects (Aschoff and von Goetz 1988; Rietveld et al. 1993).
1.1.3 Temperature Compensation
Temperature compensation was inferred by Pittendrigh as an essential property of circadian rhythms necessary to prevent the speed of the clock to be influenced by the temperature in the environment. For a detailed description on how this hypothesis was conceived and proved, we refer the reader to Pittendrigh (1993). The thermic coefficient or Q10 refers to the factor by which any metabolic process changes by an increase of 10 °C in the environment; thus, a Q10 of 1 indicates the process is not altered, while a Q10 of 2 means the speed of the process duplicates. The period of free-running rhythms has a Q10 close to 1 which indicates that it remains almost constant in a wide range of temperatures. This property was demonstrated in practically all species studied, and it is considered as a fundamental characteristic of circadian rhythmicity. The mechanisms involved in such property are still under investigation.
1.2 The Search for Circadian Clocks
During the 1970s circadian clocks became concrete biological entities in different species. In invertebrates the possible locus of circadian clocks was located in the brain of the silk moth (Truman 1972) and the eye of the Aplysia (Jacklet and Geronimo 1971) and Bulla (Block and Wallace 1982), while in vertebrates the clocks were identified in the pineal gland in birds (Gaston and Menaker 1968) and in the suprachiasmatic nuclei (SCN) in the rat hypothalamus (Moore and Eichler 1972; Stephan and Zucker 1972) (Fig. 1.3). All these studies stimulate the search for studying the mechanisms of circadian clocks at systemic, cellular, and molecular levels. When these and other studies were published, there was an intense debate whether they indicate or not the presence of a circadian oscillator or they were merely part of the clockwork or gear mechanism. It was thus necessary to outline the minimal criteria to be met in order to positively identify a circadian clock; such criteria included the following: circadian rhythmicity shall be completely disrupted when the putative cells are ablated; rhythmicity will be restored when the putative cells are transplanted from an intact donor to a lesion host; brief stimulation of the putative cells will induce phase shifts in overt rhythmicity; and when isolated from the organism, the putative cells shall continue to exhibit oscillations with a circadian period (Menaker et al. 1978). Although the criteria are enunciated here as referring to a cell or group of cells, the criteria are also valid to identify molecular components of the circadian oscillators such as genes, enzymes, and cell signaling processes.
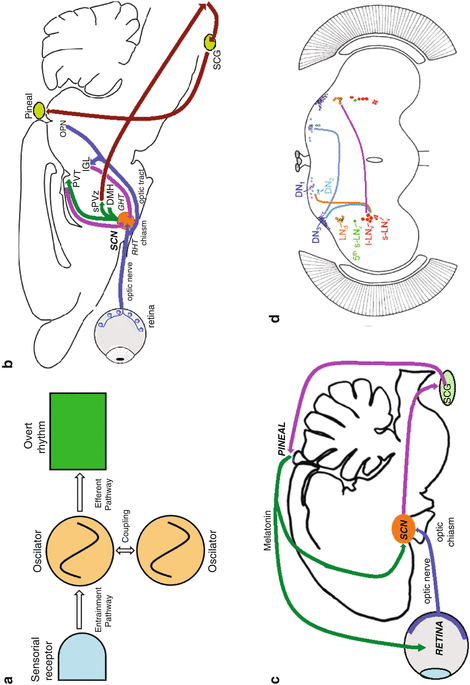
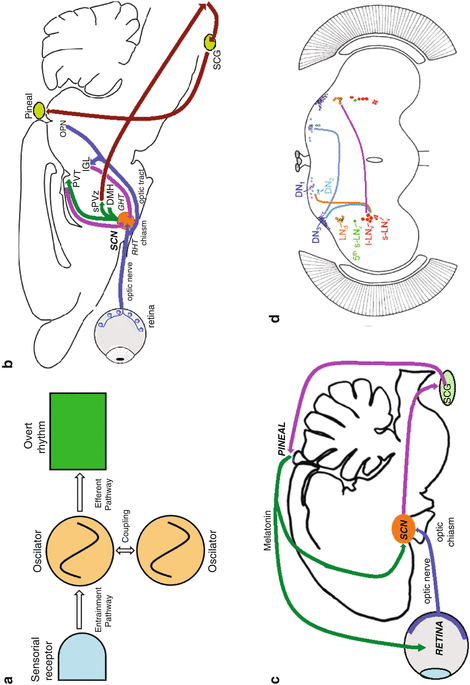
Fig. 1.3
Schematic of circadian systems in different species. (a) Conceptual model of the circadian system according to Eskin (Skinogram). (b) Rodent. (c) Avian. (d) Drosophila. Abbreviations in b and c: SCN suprachiasmatic nuclei (circadian clock), sPVZ subparaventricular zone, DMH dorsomedial hypothalamus, PVT thalamic paraventricular nucleus, IGL intergeniculate leaflet, OPN olivary pretectal nucleus, SCG superior cervical ganglion, RHT retino-hypothalamic tract, GHT geniculo-hypothalamic tract. Abbreviations in d: DN neuron clusters (1–3) in the dorsal brain, LNd dorsal–lateral brain, I-LNv large ventral lateral neurons, s-LNv small ventral–lateral neurons, 5th fifth small ventral–lateral neuron
Besides the characteristics summarized previously, a conceptual black-box model of circadian clocks was developed. The model involved an oscillator (the actual clock mechanism) which allowed the measurement of biological time; sensorial receptors which input to the oscillator and allows entrainment to environmental cycles; and output pathways which transmit the time signal from the oscillator to the effector systems of the organism which will generate the overt rhythms (Eskin 1979). As circadian clocks were identified in different animals, the concept of the circadian clock was modified to that of circadian system, which differentiates among the time-measuring entity (the oscillator or clock itself) and the inputs and output elements of the clock. Furthermore, although the original circadian system referred to a single oscillator, evidence suggested that there might be several oscillators in an organism; in such case, in order to maintain its internal synchronization, it would be necessary to postulate signaling processes to couple the different oscillators among themselves and sustain the temporal organization of the individual (Fig. 1.3).
1.2.1 Unraveling the Molecular Circadian Clock
The general structure of a biological oscillator, circadian or otherwise, was proposed in the early 1980s from the analysis of a number of well-characterized biological and chemical oscillations. Thus, it was demonstrated that a system with a delayed negative feedback loop behaves as an oscillator. The delayed element of the system is necessary to set the system to oscillate, and its kinetics determines the periodicity of the oscillation (Friesen and Block 1984). It is worth noting that in the absence of a significant delay, the feedback loop will keep a constant output of the system (Wiener 1948). On the other hand, experiments involving pharmacological blockade of gene transcription or its translation into proteins in Bulla gouldiana were able to stop the circadian clock in a reversible manner (Khalsa et al. 1992); these evidences lead to propose that the circadian clock comprised a transcription–translation loop (Block et al. 1995). These two hypotheses had a great influence in our notion of how circadian oscillators were organized at the cellular and molecular level.
The first step in the search for the molecular substrate of circadian rhythms occurred in 1971 when Konopka and Banzer described an arrhythmic mutation in Drosophila melanogaster; they identified the affected locus and call it per from period (of rhythmicity). A decade later, the gene was cloned (Reddy et al. 1984), and about 1990, Hardin et al. demonstrated that the Per protein feeds back to the per gene to regulate its own mRNA level. In 1973 mutants of Neurospora crassa with altered circadian periods were identified and named frequency mutants, since the name period was already taken (Feldman and Hoyle 1973). Some years later, the gene frq was identified and cloned; a negative feedback loop was described, where the Frq protein regulates its own mRNA transcription (Aronson et al. 1994). Some years later, the clock mutation was identified in the mouse and the gene was cloned (King et al. 1997) and was named after the acronym of circadian locomotor output cycles kaput. Interestingly, mouse clock mRNA levels did not show circadian oscillations as in the Drosophila ortholog (Shearman et al. 1999 ). On the other hand, homology screens of mouse c-DNA libraries to Drosophila per gene led to the finding of a mouse ortholog which was named RIGI, while independently a second ortholog for Drosophila per was characterized in mice (Shearman et al. 1997; Sun et al. 1997). These findings together with the contributions of other groups lay down the basis for the current knowledge on circadian clock genes (Fig. 1.4). Nowadays it has been shown that most circadian oscillators consist of delayed feedback transcription–translation loops of genes that regulate its own expression at least in eukaryotic cells (Lowrey and Takahashi 2004; Dunlap 1999; Hardin et al. 1990; Zheng and Sehgal 2012).
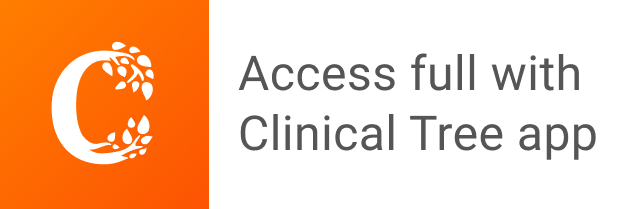