Eugene P. Steffey1, Robert J. Brosnan2, and Khursheed R. Mama3 1 Emeritus Professor, Department of Surgical and Radiological Sciences, School of Veterinary Medicine, University of California–Davis, Davis, California, USA 2 Department of Surgical and Radiological Sciences, School of Veterinary Medicine, University of California–Davis, Davis, California, USA 3 Department of Clinical Sciences, College of Veterinary Medicine and Biomedical Sciences, Colorado State University, Fort Collins, Colorado, USA Inhalation anesthetics are used widely for the anesthetic management of animals. They are unique among anesthetic drugs because they are administered, and in large part removed from the body, via the lungs. Their popularity arises in part because their pharmacokinetic characteristics favor predictable and rapid adjustment of anesthetic depth. In addition, a special apparatus is usually used to deliver the inhaled agents. This apparatus includes a source of oxygen (O2) and a patient breathing circuit, which in turn usually includes an endotracheal tube or face mask, a means of eliminating carbon dioxide (CO2), and a compliant gas reservoir. These components help minimize patient morbidity or mortality because they facilitate lung ventilation and improve arterial oxygenation. In addition, inhalation anesthetics in gas samples can be readily measured continuously. Measurement of inhalation anesthetic concentration enhances the precision and safety of anesthetic management beyond the extent commonly possible with injectable anesthetic agents. Over the nearly 150 years that inhalation anesthesia has been used in clinical practice, less than 20 agents have actually been introduced and approved for general use with patients (Fig. 28.1) [1]. Less than 10 of these have a history of widespread clinical use in veterinary medicine, and only four are of current veterinary importance in North America. It is this group of anesthetics that is the focus of this chapter. Isoflurane remains the most widely used veterinary inhalation anesthetic in North America, with sevoflurane a close second. Other inhalation anesthetics include desflurane and the gaseous agent nitrous oxide (N2O), which are used much less frequently. Halothane, once the most popular volatile anesthetic throughout the world, is no longer commercially distributed in North America. However, it remains available elsewhere (at least on a limited basis), and, considering the agent’s widespread international distribution, we will continue to include information here on some actions of clinical importance to veterinary anesthesia. Two additional volatile agents continue to receive brief attention for different reasons. Methoxyflurane, an agent popular during the period of about 1960 to 1990, has not been commercially available in North America for many years. However, because of some of its physicochemical characteristics, its inclusion here has value, especially to students of our clinical discipline and pharmacologists, and permits easy comparison to agents of more current interest. It remains commercially available in some global regions especially, for example, in the southern hemisphere. Enflurane, introduced for use in human patients in 1972 and still commercially available, has little or no use in veterinary practice in North America. Information on this volatile anesthetic was included in previous editions of this text. However, because enflurane is only in very limited and focused veterinary use elsewhere, we have deleted most information about this agent in the present chapter and refer interested readers to the fourth edition of this text for some coverage. Although of investigational and very limited human patient interest, a review of xenon is again not included in this animal patient‐focused chapter. Figure 28.1 Inhalation anesthetics introduced for widespread clinical use. Source: Adapted from Eger [1]. In this edition, information on agents of largely historical interest again has not been included. Readers interested in aspects of these formerly used agents are referred to the earlier editions of this and other textbooks [2–6]. Examples of such agents are diethyl ether and chloroform. A more complete listing is noted in Fig. 28.1. The chemical structure of inhalation anesthetics and their physical properties determine their actions and safety of administration. An in‐depth analysis of the impact of agent chemical structure and physical properties is beyond the scope of this chapter. However, brief discussion of aspects of Fig. 28.2 and Table 28.1 is appropriate because the physicochemical characteristics summarized determine and/or influence practical considerations of their clinical use. For example, they determine the form in which the agents are supplied by the manufacturer (e.g., as a gas or liquid) and account for the resistance of the anesthetic molecule to degradation by physical factors (e.g., heat and light) and substances it contacts during use (e.g., metal components of the anesthetic delivery apparatus and the CO2 absorbents such as soda lime). The equipment necessary to safely deliver the agent to the patient (e.g., vaporizer and breathing circuit) is influenced by some of these properties, as are the agent’s uptake, distribution within, and elimination (including potential for metabolic breakdown) from the patient. In summary, a knowledge and understanding of fundamental properties permits intelligent use of contemporary anesthetics. Figure 28.2 Chemical structure of inhalation anesthetics in current use for animals. Trade names are given in parentheses. Most contemporary inhalation anesthetics are organic compounds, except N2O (Fig. 28.2) (xenon is another notable inorganic anesthetic). Agents of current interest are further classified as either aliphatic (i.e., straight or branch chained) hydrocarbons or ethers (i.e., two organic radicals attached to an atom of oxygen; the general structure is ROR). In the continued search for a less reactive, more potent, nonflammable inhalation anesthetic, focus on halogenation (i.e., addition of fluorine, chlorine, or bromine) of these compounds has predominated. Chlorine and bromine especially convert many compounds of low anesthetic potency into more potent drugs. Historically, interest in fluorinated derivatives was delayed until the 1940s because of difficulties in synthesis, and thus quantities available for study were limited. Methods of synthesis, although difficult, improved considerably and facilitated new agent discovery (see Fig. 28.2). It is interesting that organic fluorinated compounds are a group of extreme contrasts – some are toxic, others are not; some are inert, others are highly reactive. In some anesthetics, fluorine is substituted for chlorine or bromine to improve stability, but at the expense of reduced anesthetic potency and solubility. Halothane (see Fig. 28.2) is a halogenated, aliphatic saturated hydrocarbon (ethane). Predictions that a halogenated structure would confer non‐flammability and molecular stability encouraged the development of halothane in the early 1950s. However, soon after clinical introduction, it was observed that the concurrent presence of halothane and catecholamines increased the incidence of cardiac arrhythmias, especially in human patients. An ether linkage in the molecule reduces the incidence of cardiac arrhythmias. Consequently, this chemical structure is a predominant characteristic of all agents, which have been developed or entered clinical use since the introduction of halothane (see Fig. 28.2). Table 28.1 Some physical and chemical properties of inhalation anesthetics used clinically in animals. a Methoxyflurane is no longer commercially available in the United States. Despite many favorable characteristics and improvements over earlier anesthetics (see Fig. 28.1) that included improved chemical stability, halothane is susceptible to decomposition. Accordingly, it is stored in dark bottles and a very small amount of a preservative, thymol, is added to slow decomposition. Thymol is much less volatile than halothane and over time concentrates within the devices used to control delivery of the volatile anesthetic (i.e., vaporizers) and causes them to malfunction. To achieve greater molecular stability, fluorine is substituted for chlorine or bromine in the anesthetic molecule. This chemical manipulation added shelf‐life to the substance and negated the need for additives such as thymol. Unfortunately, the fluorine ion is also toxic to some tissues (e.g., kidneys), which is of clinical concern if the parent compound (e.g., historically, most notably methoxyflurane) is not resistant to metabolism (see Fig. 28.2). Life is supported via a constant interchange of respiratory gases (O2 and CO2) between cells and the external environment and blood transport. Inhalation anesthetics similarly gain entrance and exit the body via the respiratory system and their movement from a container to sites of action in the central nervous system (CNS) involves similar considerations as respiratory gases. Early in the process of controlled transfer, the agent is diluted to an appropriate amount (partial pressure or concentration) and directed to the respiratory system in a gas mixture that contains enough O2 to support life. The chain of events that ensues is influenced by many physical and chemical characteristics that can be quantitatively described (Tables 28.1–28.4). The practical clinical applications of these quantitative descriptions are reviewed here. Limited space does not permit in‐depth review of all underlying principles, and readers interested in further background information are referred elsewhere [8,9]. The physical characteristics of importance to our understanding of the action of inhalation anesthetics can be conveniently divided into two general categories: those that determine the means by which the agents are administered and those that help determine their kinetics in the body. This information is applied in the clinical manipulation of anesthetic induction and recovery and in facilitating changes in anesthetic‐induced CNS depression in a timely fashion. A variety of physical/chemical properties determine the means by which inhalation anesthetics are administered. These include characteristics such as boiling point, liquid density (specific gravity), and vapor pressure. Molecules are in a constant state of motion and exhibit a force of mutual attraction. The degree of attraction is evident by the state in which the substance exists (i.e., solid, liquid, or gas). Molecular motion increases as energy (e.g., in the form of heat) is added to the molecular aggregate and decreases as energy is removed. With increased motion, there is a reduction in the intermolecular forces; if conditions are extreme enough, a change in physical state may ensue. All substances exist naturally in a particular state but can be made to exist (at least in theory) in any or all phases by altering conditions. Water, as an example, exists as ice (mutual molecular attraction is great), liquid water, or water vapor (attraction considerably reduced) depending upon conditions. Inhalation anesthetics are either gases or vapors. In relation to inhalation anesthetics, the term “gas” refers to an agent, such as N2O, that exists in its gaseous form at room temperature and sea level pressure. The term “vapor” indicates the gaseous state of a substance that at ambient temperature and pressure is a liquid. With the exception of N2O, all the contemporary anesthetics fall into this category. Desflurane (see Table 28.1) is one volatile liquid that comes close to the transition stage and offers some unique (among the inhalation anesthetics) considerations to be discussed later in this chapter [7]. Whether inhalation agents are supplied as a gas or volatile liquid under ambient conditions, the same physical principles apply to each agent when it is in the gaseous state. Molecules move about in haphazard fashion at high speeds and collide with each other or the walls of the containing vessel. The force per unit area of the bombardment is measurable and referred to as “pressure.” In the case of gases, if the space or volume in which the gas is enclosed is increased, the number of bombardments decreases (i.e., a smaller number of molecular collisions per unit time) and then the pressure decreases. The behavior of gases is predictably described by various gas laws. Relationships such as those described by Boyle’s law (volume versus pressure), Charles’s law (volume versus temperature), Gay‐Lussac’s law (temperature versus pressure), Dalton’s law of partial pressure (the total pressure of a mixture of gases is equal to the sum of the partial pressures of all the gaseous substances present), and others are important to our overall understanding of aspects of respiratory and anesthetic gases and vapors. However, in‐depth descriptions of these principles are beyond the scope of this chapter and readers are referred elsewhere for this information [9]. Table 28.2 Partition coefficients (solvent/gas) of some inhalation anesthetics at 37 °C. Source: Tissue samples are derived from human sources. Data are from sources referenced in the 4th edition of this text. Table 28.3 Blood/gas partition coefficients for desflurane, halothane, isoflurane, methoxyflurane, and sevoflurane in species of common clinical and/or research interest in veterinary medicine, including for comparison similarly derived values for humans. Note, some values reported in the present and the 5th editions of this text differ from earlier editions. Present and 5th edition values are taken primarily from the 2012 reported work of Soares et al. [14], except where indicated. Values are reported for 37 °C. A dash indicates the value was not found. Table 28.4 Rubber or plastic/gas partition coefficients at room temperature. These data are summarized from multiple sources as reported in Eger [1], with some differences in methods of determination. The data from Targ et al. [15] indicate more recently derived data which, unlike earlier data, were recorded following complete equilibration with these materials. Where there is overlap, the ranking of partition coefficients is consistent with halothane > isoflurane > sevoflurane > desflurane. Combining both groupings yields methoxyflurane > halothane > enflurane > isoflurane > sevoflurane > desflurane > N2O. Quantities of inhalation anesthetic agent are usually characterized by one of three methods: pressure (e.g., in mmHg), concentration (in vol %), or mass (in mg or g). The form most familiar to clinicians is that of concentration (e.g., X% of agent A in relation to the whole gas mixture). Agent analysis monitoring equipment samples inspired and expired gases and provides readings for inhalation anesthetics. Precision vaporizers used to control delivery of inhalation anesthetics are calibrated in percentage of agent, and effective doses are almost always reported in percentages. Pressure is also an important way of describing inhalation anesthetics and is further discussed under the heading of anesthetic potency. A mixture of gases in a closed container will exert pressure on the walls of the container. The individual pressure of each gas in a mixture of gases is referred to as its “partial pressure.” As noted earlier, this expression of the behavior of a mixture of gases is known as Dalton’s law, and its use in understanding inhalation anesthesia is inescapable. Use of the concept of partial pressure is important in understanding inhalation anesthetic action in a multiphase biologic system because, unlike concentration, the partial pressure of an agent is the same in different compartments that are in equilibrium with each other. That is, in contradistinction to concentration or volume percent, an expression of the relative ratio of gas molecules in a mixture, partial pressure is an expression of the absolute value. Molecular weight and agent density are used in many calculations to convert from liquid to vapor volumes and mass. Briefly (and in simplified fashion), Avogadro’s principle is that equal volume of all gases under the same conditions of temperature and pressure contain the same number of molecules (6.022 × 1023 [Avogadro’s number] per gram molecular weight). Furthermore, under standard conditions, the number of gas molecules in a gram molecular weight of a substance occupies 22.4 L. In order to compare properties of different substances of similar state, it is necessary to do so under comparable conditions; with respect to gases and liquids, this usually means with reference to pressure and temperature. Unless otherwise indicated, physical scientists have arbitrarily selected standard conditions as 0 °C (273 K in absolute scale) and 760 mmHg pressure (1 atmosphere at sea level). If conditions differ, appropriate temperature and/or pressure corrections must be applied to resultant data. The weight of a given volume of liquid, gas, or vapor may be expressed in terms of its density or specific gravity. The density is an absolute value of mass (usually grams) per unit volume (for liquids, volume = 1 mL; for gases, 1 L at standard conditions). The specific gravity is a relative value; that is, the ratio of the weight of a unit volume of one substance to a similar volume of water, in the case of liquids, or air, in the case of gases (or vapors), under similar conditions. The value of both air and water is one. At least for clinical purposes, the value for density and specific gravity for an inhalation anesthetic is the same. Thus, for example, we can determine the volume of isoflurane gas (vapor) at 20 °C from 1 mL of isoflurane liquid according to the scheme given in Box 28.1. This type of calculation has practical applications. For example, to determine the savings in isoflurane liquid afforded by reducing the fresh gas (e.g., O2) inflow rate, a series of calculations as presented in Box 28.2 can be made. Molecules of liquids are in constant random motion. Some of those in the surface layer gain sufficient velocity to overcome the attractive forces of neighboring molecules and in escaping from the surface, enter the vapor phase. The change in state from a liquid to a gas phase is known as “vaporization” or “evaporation.” This process is dynamic and in a closed container that is kept at a constant temperature, eventually reaches an equilibrium whereby there is no further net loss of molecules to the gas phase (i.e., the numbers of molecules leaving and returning to the liquid phase are equal). The gas phase at this point is saturated. Molecules of a vapor exert a force per unit area or pressure in exactly the same manner as molecules of a gas. The pressure (units of measure are mmHg) that the vapor molecules exert when the liquid and vapor phases are in equilibrium is known as the “vapor pressure.” Thus, the vapor pressure of an anesthetic is a measure of its ability to evaporate; that is, it is a measure of the tendency for molecules in the liquid state to enter the gaseous (vapor) phase. The vapor pressure of a volatile anesthetic must be at least sufficient to provide enough molecules of anesthetic in the vapor state to produce anesthesia at ambient conditions. The saturated vapor pressure represents a maximum concentration of molecules in the vapor state that exists for a given liquid at each temperature. Herein lies a practical difference between substances classified as a gas or vapor; a gas can be administered over a range of concentrations from 0% to 100%, whereas the vapor has a ceiling that is dictated by its vapor pressure. The saturated vapor concentration can be easily determined by relating the vapor pressure to the ambient pressure. For example, in the case of isoflurane (see Table 28.1), a maximal concentration of 32% isoflurane is possible under usual conditions and 20 °C (i.e., [240/760] × 100 = 32%, where 760 mmHg is the barometric pressure at sea level). With other variables considered constant, the greater the vapor pressure, the greater the concentration of the drug deliverable to the patient. Therefore, again from Table 28.1, isoflurane, for example, is more volatile than methoxyflurane under similar conditions. The barometric pressure also influences the final concentration of an agent. For example, in locations such as Denver, Colorado, where the altitude is about 5000 feet above sea level and the barometric pressure is only about 635 mmHg, the saturated vapor concentration of isoflurane at 20 °C is now (240/635) × 100 = 37.8%. It is important to recognize that the saturated vapor pressure at 1 atmosphere is unique for each volatile anesthetic agent and depends only on its temperature. In this case, the effect of barometric pressure can be neglected over ranges normally encountered in the practice of anesthesia. Thus, for a given agent, the graph of the saturated vapor pressure versus temperature is a curve as shown in Fig. 28.3. From this graph, it can be seen that if the temperature of the liquid is increased, more molecules escape the liquid phase and enter the gaseous phase. The greater number of molecules in the vapor phase results in a greater vapor pressure and vapor concentration. Conversely, if the liquid is cooled, the reverse occurs and vapor concentration decreases. Liquid cooling may occur not only as a result of ambient conditions but also as a natural consequence of the vaporization process. For example, during vaporization the “fastest” molecules at the surface escape first. With depletion of these “high‐energy” molecules, the average kinetic energy of those left behind is reduced and there is a tendency for the temperature of the remaining liquid to fall if this process is not compensated for externally. As the temperature decreases, the vapor pressure, and thus the vapor concentration, also decreases. Figure 28.3 Vapor pressure as a function of temperature for six volatile anesthetics. Curves are generated from Antoine equations. The boiling point of a liquid is defined as the temperature at which the vapor pressure of the liquid is equal to the atmospheric pressure. Customarily, the boiling temperature is stated at the standard atmospheric pressure of 760 mmHg. The boiling point decreases with increasing altitude because the vapor pressure does not change but the barometric pressure decreases. The boiling point of N2O is −89 °C (see Table 28.1) at 1 atmosphere pressure at sea level. It is thus a gas under operating room conditions. Because of this, it is distributed for clinical purposes in steel tanks compressed to the liquid state at about 750 psi (pounds per square inch; 750 psi/14.9 psi [1 atmosphere] = 50 atmospheres pressure). As the N2O gas is drawn from the tank, liquid N2O is vaporized, and the over‐riding gas pressure remains constant until no further liquid remains in the tank. At that point, only N2O gas remains, and the gas pressure decreases from this point as remaining gas is vented from the tank. Consequently, the weight of the N2O minus the weight of the tank rather than the gas pressure within the tank is a more accurate guide to the remaining amount of N2O in the tank [16]. Desflurane also possesses an interesting consideration because its boiling point (see Table 28.1) is near room temperature. This characteristic accounted for an interesting engineering challenge in developing an administration device (i.e., a vaporizer) for routine use in the relatively constant environment of the operating room and limits further consideration of its use in all but a narrow range of circumstances commonly encountered in veterinary medical applications. For example, because of its low boiling point, even evaporative cooling has a large influence on vapor pressure and thus the vapor concentration of gas mixtures delivered to the patient. The saturated vapor pressure of most volatile anesthetics is of such magnitude that the maximal concentration of anesthetic attainable at usual operating room conditions is above the range of concentrations that are commonly necessary for safe clinical anesthetic management. Therefore, some control of the delivered concentration is necessary and usually provided by a device known as a “vaporizer.” The purpose of the vaporizer is to dilute the vapor generated from the liquid anesthetic with O2 (or O2 plus N2O, or O2 plus air mixtures) to produce a more satisfactory inspired anesthetic concentration. This anesthetic dilution is usually accomplished as indicated in the model shown in Fig. 28.4 by diverting the gas entering the vaporizer into two streams: one that enters the vaporizing chamber (anesthetic chamber volume: Vanes) and the other that bypasses the vaporizing chamber (dilution volume or Vdilution). If the vaporizer is efficient, the carrier gas passing through the vaporizing chamber becomes completely saturated to an anesthetic concentration (%) reflected by (anesthetic agent vapor pressure/atmospheric pressure) × 100, at the vaporizer chamber temperature. The resultant anesthetic concentration then is decreased (diluted) downstream by the second gas stream to a “working” concentration. In modern, precision, agent‐specific vaporizers, no mental effort is required – just set the dial, as the manufacturers have precalibrated the vaporizer for accurate delivery of the dialed concentration (within some variability, say 10% of delivered concentration, allowance). Nevertheless, it is helpful to our overall understanding to know the principles underlying this convenience and how to apply these principles in the use of older, non‐compensated measured flow vaporizers. To calculate the anesthetic concentration from the vaporizer, one must know the vapor pressure of the agent (at the temperature of use), the atmospheric pressure, the fresh gas flow entering the vaporizing chamber, and the diluent gas flow. Then: More detail for interested readers is given in Fig. 28.4. Anesthetic gases and vapors dissolve in liquids and solids. The solubility of an anesthetic is a major characteristic of the agent and has important clinical ramifications. For example, anesthetic solubility in blood and body tissues is a primary factor in the rate of uptake and its distribution within the body. It is therefore a primary determinant of the speed of anesthetic induction and recovery. Solubility in lipid bears a strong relationship to anesthetic potency, and its tendency to dissolve in anesthetic delivery components such as rubber goods influences equipment selection and other aspects of anesthetic management. As previously mentioned, molecules of a gas that overlie a liquid surface are in random motion and some penetrate the liquid surface. After entering the liquid, they intermingle with the molecules of the liquid (i.e., the gas dissolves in the liquid). There is a net movement of the gas into the liquid until equilibrium is established between the dissolved gas in the liquid and the undissolved portion above the liquid. At this time, there is no further net gain of gas molecules by the liquid, and the number of gas molecules entering the liquid equals the number leaving. The gas molecules within the liquid exert the same pressure or tension that they exert in the gas phase. If the pressure (i.e., the number of gas molecules overlying the liquid) is increased, more molecules pass into the liquid and the pressure within the liquid is increased. This net inward movement of gas molecules continues until a new equilibrium is established between the pressure of the gas in the liquid and that overlying the liquid. Alternatively, if the pressure of gas overlying the liquid is somehow decreased below that in the liquid, gas molecules escape from the liquid. This net outward movement of gas molecules from the liquid phase continues until equilibrium between the two phases is re‐established. Figure 28.4 An anesthetic vaporizer model to assist in illustrating the principles associated with the calculation of the vapor concentration of an inhalation anesthetic emerging from a vaporizer. Conditions associated with halothane delivery in San Francisco (i.e., at sea level; barometric pressure = 760 mm Hg) at 20 °C are used as an example of general principles. The amount, that is, the total number of molecules of a given gas dissolving in a solvent, depends on the chemical nature of the gas itself, the partial pressure of the gas, the nature of the solvent, and the temperature. This relationship is described by Henry’s law: where V is the volume of gas, P is the partial pressure of the gas, and S is the solubility coefficient for the gas in the solvent at a given temperature. Henry’s law applies to gases that do not combine chemically with the solvent to form compounds. Before leaving this basic information, a brief focus on a number of variations may be helpful. First, it is important to recognize that if the atmosphere that overlies the solvent is made up of a mixture of gases, then each gas dissolves in the solvent in proportion to the partial pressure of the individual gases. The total pressure exerted by the molecules of all gases within the solvent equals the total gas pressure lying above the solvent. Within the body, there is a partition of anesthetic gases between blood and body tissues in accordance with Henry’s law. This process can perhaps be better understood by visualizing a system composed of three compartments (e.g., gas, water, and oil) contained in a closed container (Fig. 28.5). In such a system the gas overlies the oil, which in turn overlies the water. Because there is a passive gradient from the gas phase to the oil, gas molecules move into the oil compartment. This movement in turn develops a gradient for the gas molecules in oil relative to water. If gas is continually added above the oil, there will be a continual net movement of the gas molecules from the gas phase into both the oil and, in turn, the water. At a given temperature, when no more gas dissolves in the solvent, the solvent is said to be fully saturated. At this point, the pressure of the gas molecules within the three compartments will be equal but the amount (i.e., the number of molecules or volume of gas) partitioned between the two liquids will vary with the nature of the liquid and gas. Finally, it is important to understand that the amount of gas that goes into solution depends upon the temperature of the solvent. Less gas dissolves in a solvent as temperature increases, and more gas is taken up as solvent temperature decreases. For example, as water is heated, air bubbles appear inside the container as a result of the decreasing solubility of the air in water. Conversely, as blood is cooled from a normal body temperature (e.g., hypothermia), gases become more soluble in blood. Figure 28.5 Diagrammatic representation of an anesthetic gas distributing itself among three compartments (gas, oil, and water). At equilibrium, the number of anesthetic molecules in the three compartments differs but the pressure exerted by the anesthetic molecules is the same in each compartment. The extent to which a gas will dissolve in a given solvent is usually expressed in terms of its solubility coefficient (see Table 28.2). With inhalation anesthetics, solubility is most commonly measured and expressed as a partition coefficient (PC). Other measurements of solubility include the Bunsen and Ostwald solubility coefficients [9,17]. The PC is the concentration ratio of an anesthetic in the solvent and gas phases (e.g., blood and gas; Fig. 28.6) or between two tissue solvents (e.g., brain and blood; see Table 28.2). It thus describes the capacity of a given solvent to dissolve the anesthetic gas. That is, how the anesthetic will partition itself between the gas and the liquid solvent phases after equilibrium has been reached. Remember, anesthetic gas movement occurs because of a partial pressure difference in the gas and liquid solvent phases, so when there is no longer any anesthetic partial pressure difference, there is no longer any net movement of anesthetic and equilibrium has been achieved. Solvent/gas PCs are summarized in Table 28.2. Values noted in this table are for human tissues because they are most widely available in the anesthesia literature. Comparative data for blood solubility of desflurane, isoflurane, and sevoflurane in a variety of species of clinical interest in veterinary medicine are listed in Table 28.3. Regardless of the species, it is important to emphasize that many factors can alter anesthetic agent solubility [17–20]. Perhaps the most notable after the nature of the solvent is that of temperature. Of all the PCs that have been described or are of interest, two are of particular importance in the practical understanding of anesthetic action. They are the blood/gas and the oil/gas solubility coefficients. Figure 28.6 Illustration of two hypothetical gases (A. and B.) with varying blood/gas partition coefficients. See text for explanation. Source: Adapted from Eger [17]. Blood/gas solubility coefficients (see Tables 28.2 and 28.3) provide a means for predicting the speed of anesthetic induction, recovery, and change of anesthetic depth. Assume, for example, that anesthetic A has a blood/gas PC value of 15. This means that the concentration of the anesthetic in blood will be 15 times greater at equilibrium than that in alveolar gas. Expressed differently, the same volume of blood, say 1 mL, will hold 15 times more anesthetic A than 1 mL of alveolar gas despite an equal partial pressure. Alternatively, consider anesthetic B with a PC of 1.4. This PC indicates that at equilibrium, the amount of anesthetic B is only 1.4 times greater in blood than it is in alveolar air. Comparing the PC of anesthetic A with that of anesthetic B indicates that anesthetic A is much more soluble in blood than B (nearly 11 times more soluble: 15/1.4). From this, and assuming other conditions are equal, anesthetic A will require a longer time of administration to attain a partial pressure in the body for a particular endpoint (say, anesthetic induction) than will anesthetic B. Also, since there is more anesthetic A contained in blood and other body tissues under similar conditions, elimination (and therefore anesthetic recovery) will be prolonged when compared to anesthetic B. The oil/gas PC is another solubility characteristic of clinical importance (see Table 28.2). This PC describes the ratio of the concentration of an anesthetic in oil (olive oil is the standard) and gas phases at equilibrium. The oil/gas PC correlates directly with anesthetic potency (see Anesthetic dose: the minimum alveolar concentration (MAC) and Mechanism of action sections later in this chapter) and describes the capacity of lipids for anesthetic. Solubility characteristics for various tissues (see Tables 28.2 and 28.3) and other media, such as rubber and plastic (see Table 28.4), are also important. For example, the solubility of a tissue determines in part the quantity of anesthetic removed from the blood to which it is exposed. The higher the tissue solubility, the longer it will take to saturate the tissue with anesthetic agent. Thus, other things considered equal, anesthetics that are very soluble in tissues will require a longer period for induction and recovery. If the amount of rubber goods in the apparatus used to deliver the anesthetic to the patient is substantial and the anesthetic agent solubility in rubber is large (as, for example, was common with equipment in use prior to the 21st century), the amount of uptake of anesthetic agent by the rubber may also be of clinical significance. The aim in administering an inhalation anesthetic to a patient is to achieve an adequate partial pressure or tension of anesthetic (Panes) in the central nervous system (CNS, e.g., brain; for purposes of this discussion considerations of anesthetic delivery to spinal cord sites of action are considered similar to those of the brain) to cause a desired level of CNS depression commensurate with the definition of general anesthesia. Anesthetic depth varies directly with Panes in brain and spinal cord tissue. The rate of change of anesthetic depth is of obvious clinical importance and is directly dependent upon the rate of change in anesthetic tensions in the various media in which it is contained before reaching the brain. Thus, knowledge of the factors that govern these relationships is of fundamental importance to skillful control of general inhalation anesthesia. Inhalation anesthetics are unique among the classes of drugs that are used to produce general anesthesia because they are administered via the lungs. The pharmacokinetics of the inhaled anesthetics describe the rate of their uptake by blood from the lungs, distribution in the body, and eventual elimination by the lungs and other routes. Readers seeking more in‐depth coverage are directed to reviews by Eger [17,21,22] and Mapleson [23]. Inhalation anesthetics, similar to the gases of respiration (i.e., O2 and CO2), move down a series of partial pressure gradients from regions of higher tension to those of lower tension until equilibrium (i.e., equal pressure throughout the apparatus and body tissues) is established. Thus, on induction, the Panes at its source in the vaporizer is high, as is dictated by the vapor pressure, and progressively decreases as anesthetic travels from vaporizer to patient breathing circuit, from circuit to lungs, from lungs to arterial blood, and finally, from arterial blood to body tissues (e.g., the brain; Fig. 28.7). Of these, the alveolar partial pressure of anesthetic, PAAnes, is pivotal. The brain has a rich blood supply and the anesthetic in arterial blood, PaAnes, rapidly equilibrates with brain tissue, PbrainAnes. Usually, gas exchange at the alveolar level is sufficiently efficient that the PaAnes is close to PAAnes. Thus, the PbrainAnes closely follows PAAnes, and by controlling the PAAnes there is a reliable indirect way for controlling PbrainAnes and anesthetic depth. At this point, it may also be helpful to recall that although the partial pressure of anesthetic is of primary importance, we frequently define the clinical dose of an inhaled anesthetic in terms of concentration (C, i.e., vol %). As previously noted, this is because it is common practice for the clinician to regulate and/or measure respiratory and anesthetic gases in volume percent. In addition, in the gaseous phase, the relationship between the Panes and the Canes is a simple one: The fractional anesthetic concentration is of course Canes/100. However, as reviewed in the preceding section, in blood or tissues, the actual quantity of anesthetic depends on both the Panes and the anesthetic solubility (as measured by the PC) within the solvent (e.g., blood or oil). Consequently, at equilibrium, the partial pressure of the gas in the alveoli and among tissue compartments will be equal, although concentrations will vary within these tissues. Figure 28.7 The flow pattern of inhalation anesthetic agents during anesthetic induction and recovery. Inhalation anesthesia may be viewed as the development of a series of partial pressure (tension) gradients. During induction, there is a high anesthetic tension in the vaporizer that decreases progressively as the flow of anesthetic gas moves from its source to the brain. Some of these gradients are easily manipulated by the anesthetist; others are not or are difficult to achieve. The PA of anesthetic is a balance between anesthetic input (i.e., delivery to the alveoli) and loss (uptake by blood and body tissues) from the lungs. A rapid rise in the PA of anesthetic is associated with a rapid anesthetic induction or change in anesthetic depth. Factors that contribute to a rapid change in the PA of anesthetic are summarized in Box 28.3. Delivery of anesthetic to the alveoli and therefore the rate of rise of the alveolar concentration or fraction (FA) toward the inspired concentration or fraction (FI) depends on the inspired anesthetic concentration itself and the magnitude of alveolar ventilation. Increasing either one of these or both increases the rate of rise of the PA of anesthetic; that is, with other things considered equal, there is an increase in speed of anesthetic induction or change in anesthetic level. The inspired concentration has a number of variables controlling it. First of all, the upper limit of inspired concentration is dictated by the agent vapor pressure, which in turn is dependent on temperature. This may be especially important in veterinary medicine considering the breadth of applications involving inhaled anesthesia and methods of vaporizing volatile anesthetics under widely diverse conditions (some environmental conditions are quite hostile). Characteristics of the patient breathing system can also be a major factor in generating a suitable inspired concentration under usual operating room conditions. Characteristics of special importance include the volume of the system, the amount of rubber or plastic components of the system, the position of the vaporizer relative to the breathing circuit (i.e., within or outside the circuit), and the fresh gas inflow to the patient breathing circuit. The patient breathing circuit contains a gas volume that must be replaced with gas containing the desired anesthetic concentration. Thus, the volume of the breathing circuit serves as a buffer to delay the rise of anesthetic concentration. In the management of small animals (i.e., less than 10 kg), a nonrebreathing patient circuit and/or a relatively high fresh gas inflow into the patient breathing circuit is usually used, so there should not be a clinically important difference between the delivered (e.g., vaporizer dial setting) and the inspired concentration. That is, when the vaporizer dial setting is adjusted to the desired concentration setting, the fresh gas plus anesthetic flowing from the vaporizer almost immediately contains the dialed anesthetic vapor concentration. In addition, the total gas flow is high relative to the volume of the delivery circuit, so the anesthetic concentration in the inspired breath is rapidly increased. However, with larger animals (i.e., greater than 10 kg), a rebreathing (circle) patient circuit with a CO2 absorber is most commonly used for inhalation anesthesia. The volume of this breathing circuit may be very large compared to fresh gas inflow. This volume markedly delays the rate of rise of inspired anesthetic concentration because the residual gas volume must be “washed out” and replaced by anesthetic containing fresh gas in order for the inspired concentration to increase to that delivered from the vaporizer (Fig. 28.8). In addition, rebreathing of exhaled gas (minus CO2) occurs to varying degrees with these circuits. The inspired gas is composed of exhaled and fresh gases. Because the expired gas contains less anesthetic than the fresh gas, the inspired anesthetic gas concentration will be less than that of the fresh gas leaving the vaporizer. In veterinary applications, the delaying influence of the circle circuit is most notable with anesthetic management of very large animals such as horses [24] and cattle and/or when using a closed‐circuit fresh gas flow rate (i.e., where O2 is the fresh gas and its inflow [plus anesthetic] to the circuit just meets the metabolic needs of the patient). With closed‐circuit delivery, the fresh gas inflow is very low relative to the circuit volume [8,17,24]. Figure 28.8 A comparison of the rate of increase of inspired halothane concentration toward a constant delivered concentration Finsp/Fdel in a 7 L small animal anesthetic breathing circuit (SAAM) at fresh gas flow rates of 1, 3, 6, and 12 Lpm. Source: Eger [24]. Table 28.5 Vaporizer positioning within or outside a circle patient rebreathing circuit influences inspired anesthetic concentration. The high solubility of some anesthetics (e.g., methoxyflurane; see Table 28.4) in rubber and plastic delays development of an appropriate inspired anesthetic concentration. The loss of anesthetic to these equipment “sinks” increases the apparent volume of the anesthetic circuitry and may, in some cases, be clinically important (e.g., the use of rubber hoses and a large rubber rebreathing bag on circuits designed for anesthetic management of horses). With the newest inhalation anesthetics and more modern anesthetic delivery equipment, this issue is of minor or no clinical importance. Positioning the vaporizer in relation to the patient breathing circuit will influence inspired anesthetic concentration [23,25]. For example, with the vaporizer positioned within a circle rebreathing circuit, a decrease in inspired concentration will follow an increase in fresh gas inflow to the circuit, whereas an increase in inspired concentration will result if the vaporizer is positioned outside the circuit (Table 28.5). With the loss of methoxyflurane to clinical practice, nearly all, if not all, vaporizers in use in North America are agent‐specific, precision vaporizers. This style of vaporizer is always placed upstream and outside the patient’s breathing circuit. An increase in alveolar ventilation increases the rate of delivery of inhalation anesthetic to the alveolus (Fig. 28.9). If unopposed by blood and tissue uptake of anesthetic, alveolar ventilation would rapidly increase the alveolar concentration of anesthetic so that within minutes, the alveolar concentration would equal the inspired concentration. However, in reality, the input created by alveolar ventilation is countered by absorption of anesthetic into blood. Predictably, hypoventilation decreases the rate at which the alveolar concentration increases over time compared to the inspired concentration (i.e., anesthetic induction is slowed). Alveolar ventilation is altered by changes in anesthetic depth (increased depth usually means decreased ventilation), mechanical ventilation (usually increased ventilation), and deadspace ventilation (i.e., for constant minute ventilation, a decrease in deadspace ventilation results in an increase in alveolar ventilation). Figure 28.9 The effect of ventilation on the rise of the alveolar (FA) concentration of halothane toward the inspired (FI) concentration. As noted, the FA:FI ratio increases more rapidly as ventilation is increased from 2 to 8 L/min. Source: Eger [17], with permission of Wolters Kluwer Health, Inc. Alveolar ventilation and thus the alveolar anesthetic concentration can also be influenced by administering a potent inhalation anesthetic such as halothane in conjunction with N2O. Very early in the administration of N2O (during the period of large volume uptake; the first 5–10 min of delivery), the rate of rise of the alveolar concentration of the concurrently administered inhalation anesthetic is increased. This is commonly referred to as the “second gas effect,” and this phenomenon can be applied clinically to speed anesthetic induction [17,21,26]. As noted by Eger [17], anesthetic uptake is the product of three factors: solubility (S, the blood/gas solubility; see Table 28.3), cardiac output (CO), and the difference in the anesthetic partial pressure between the alveolus and venous blood returning to the lungs (PA − PV̄/Pbar), expressed in mmHg; that is: where Pbar = barometric pressure in mmHg. Note that if any of these three factors equals zero, there is no further uptake of anesthetic by blood. As previously discussed, the solubility of an inhalation anesthetic in blood and tissues is characterized by its partition coefficient (PC; see Tables 28.2 and 28.3). Remember that a PC describes how an inhalation anesthetic distributes itself between two phases or two solvents (e.g., the quantity of agent in blood and alveoli [gas] or blood and muscle, respectively) once equilibrium is established (i.e., when the anesthetic partial pressure is equal). Based on blood/gas PCs, inhalation anesthetics range from highly soluble (methoxyflurane) to poorly soluble (N2O, desflurane, and sevoflurane). Agents such as halothane and isoflurane are intermediate. Figure 28.10 The rise in the alveolar (FA) anesthetic concentration toward the inspired (FI) concentration. Note the rise is most rapid with the least soluble anesthetic, N2O, and slowest with the most soluble anesthetic, methoxyflurane. All data are from studies of humans. Compared to an anesthetic with high blood solubility (PC), an agent with low blood solubility is associated with a more rapid equilibration because a smaller amount of anesthetic must be dissolved in the blood before equilibrium is reached with the gas phase. In the case of the agent with a high blood/gas PC, the blood acts like a large “sink” into which the anesthetic is poured and accordingly, blood is “reluctant” to give up the agent to other tissues (such as the brain). The blood serves as a conduit for drug delivery to the brain and as such can be visualized as a pharmacologically inactive reservoir that is interposed between the lungs and the agent’s site of desired pharmacologic activity (i.e., brain). Therefore, an anesthetic agent with a low blood/gas PC is usually more desirable than a highly soluble agent, because it is associated with (1) a more rapid anesthetic induction (i.e., more rapid rate of rise in alveolar concentration during induction; Fig. 28.10); (2) more precise control of anesthetic depth (i.e., alveolar concentration during the anesthetic maintenance); and (3) a more rapid elimination of anesthetic and recovery (i.e., a rapid decrease in alveolar concentration during recovery). The amount of blood flowing through the lungs and on to body tissues also influences anesthetic uptake from the lungs. The greater the CO, the more blood passes through the lungs carrying away anesthetic from the alveoli. Thus, a large CO, like increased anesthetic agent blood solubility, delays the alveolar rise of Panes (Fig. 28.11). Patient excitement is an example in which a relatively large CO is anticipated. Conversely, a reduced CO should be anticipated with a patient with poor myocardial function or severe bradycardia. Such a situation would be associated with an increase in the rate of rise of the PA of the anesthetic and this, along with other factors, makes the anesthetic induction more rapid and risky. Figure 28.11 The effect of cardiac output on the rise of the alveolar (FA) concentration of halothane toward the inspired (FI) concentration. As noted, the FA:FI ratio increases more rapidly as cardiac output is decreased from 18 to 2 L/min. Source: Eger [17], with permission of Wolters Kluwer Health, Inc. The magnitude of difference in anesthetic partial pressure between the alveoli and mixed venous blood returning to the lungs is related to the amount of uptake of anesthetic by tissues. It is not surprising that the largest gradient occurs during induction. Once the tissues no longer absorb anesthetic (i.e., equilibrium is reached), there is no longer any uptake of anesthetic from the lungs because PV̄ = PA (i.e., the mixed venous blood returning to the lungs contains as much anesthetic as when it left the lungs). The changes in gradient between the initiation of induction and equilibration result in part from the relative distribution of CO. In this regard, it is important to recognize that roughly 70–80% of the CO is normally directed to only a small volume of body tissues in a lean individual [28,29]. Tissues such as the brain, heart, hepatoportal system, and kidneys represent only about 10% of the body mass but normally receive about 75% of the total blood flow each minute. As a result, these highly perfused tissues equilibrate rapidly with arterial anesthetic partial pressure when compared to other body tissues (actual timing is influenced by agent solubility). Since the venous anesthetic pressure or tension equals that in the tissue within 10 or 15 min, about 75% of the blood returning to the lungs is the same as the alveolar tension. This presumes there has been no change in arterial anesthetic partial pressure during this time and thus uptake is reduced. Skin and muscle comprise the major bulk of the body (about 50% in humans) but at rest receive only about 15–20% of the CO, so saturation of these tissues takes hours to accomplish. Fat is a variable component of body bulk and receives only a small proportion of blood flow. Consequently, anesthetic saturation of this tissue is very slow because all anesthetics are considerably more soluble in fat than other tissue groups (see Table 28.2). Other factors can influence the magnitude of the alveolar to arterial anesthetic partial pressure gradient. For example, abnormalities of ventilation/perfusion result in an alveolar–arterial gradient proportional to the degree of abnormality [17,30,31]. Others include loss of anesthetic via the skin [32–34] and into closed gas spaces [17,21,26], and metabolism [17,21]. Figure 28.12 The fall in alveolar (FA) concentration relative to the alveolar concentration at the end of anesthesia (FAO). Note that the newest, most insoluble, volatile anesthetic, desflurane, is eliminated in humans more rapidly than the other contemporary potent anesthetics. Not shown is information for methoxyflurane. If present, the curve for methoxyflurane would appear above that for halothane. Source: Reproduced with permission from Eger [27]. The rate at which the alveolar anesthetic concentration increases relative to the inspired concentration (i.e., the rate of change in anesthetic level) is often summarized as a plot of the ratio of FA:FI versus time. The position of individual curves representing different anesthetics on a plot is related to the solubility characteristics of the anesthetics (see Fig. 28.10). The shape of the graph of FA:FI versus time is similar for all anesthetics (see Fig. 28.10). There is a rapid rise initially that results from the effect of alveolar ventilation bringing anesthetic into the lung. There is then a decrease in the rate of rise of the curve as uptake by the blood occurs. With time, the highly perfused tissues of the body equilibrate with incoming blood so that eventually about three‐quarters of the total blood flow returning to the heart has the same anesthetic partial pressure as it had when it left the lungs. Thus, further uptake from the lung is decreased and the rate of approach of the FA to FI over time is further decreased. Recovery from inhalation anesthesia results from the elimination of anesthetic from the CNS. This requires a decrease in alveolar anesthetic partial pressure (concentration), which in turn fosters a decrease in arterial and then CNS anesthetic partial pressure (see Box. 28.3). Prominent factors accounting for recovery are the same as those for anesthetic induction. The percent clearance of an inhaled anesthetic can be expressed as: Therefore, factors such as alveolar ventilation (VA), CO, and especially agent solubility greatly influence recovery from inhalation anesthesia. Indeed, the graphic curves representing the washout of anesthetic from alveoli versus time (Fig. 28.12) are essentially inverses of the wash‐in curves. That is, the wash‐out of the less soluble anesthetics is high at first (i.e., rapid wash‐out by ventilation of the lung functional residual capacity), then rapidly declines to a lower output level that continues to decrease but at a slower rate. The wash‐out of more soluble agents is also high at first, but the magnitude of decrease in alveolar anesthetic concentration is less and decreases more gradually with time (see Fig. 28.12). Figure 28.13 The decrease in the alveolar (FE) anesthetic concentration from the concentration at the time of breathing circuit disconnect (i.e., the beginning of recovery from anesthesia; FEO) is influenced by both the solubility (λ) of anesthetic and the duration of anesthesia. Source: Stoelting and Eger [36], with permission of Wolters Kluwer Health, Inc. An important factor during the wash‐out period is the duration of anesthesia. This effect and a comparison of this effect between three agents spanning a range of blood solubilities are summarized in Fig. 28.13 [36]. Times to recovery from anesthesia in, for example, rats correlate with values for blood solubility of anesthetics (Fig. 28.14) [37]. If a patient rebreathing anesthetic circuit (e.g., circle system) is in use and the patient is not disconnected from the circuit at the end of anesthesia, the circuit itself may also reduce the rate of recovery, just as the circuit was shown to decrease the rate of rise of anesthetic during induction. This influence of rebreathing circuits can be reduced by directing high flow rates of anesthetic‐free O2 into the anesthetic circuit (i.e., applying principles of a nonrebreathing circuit). Other factors that are important to varying degrees for inhalation anesthetic elimination from the body include percutaneous loss, intertissue diffusion of agents, and metabolism. While some transcutaneous movement of inhalation agent occurs, the amount under consideration is small [32–35,38]. Intertissue diffusion is of theoretical interest, but its clinical importance is limited [35,39,40]. In this regard, the impact of obesity on recovery from anesthesia may be important to consider clinically [35]. Metabolism may also play a small role with some inhalation anesthetics (e.g., methoxyflurane and perhaps even halothane), especially when associated with prolonged anesthesia [39,41–43]. Figure 28.14 Increasing the delivered concentration of inhalation anesthetic increased the time to recovery of the motor coordination necessary to remain atop a 6 cm rod rotating at 8 rpm. The duration of anesthesia was constant for all trials. Source: Eger and Johnson [37], with permission of Wolters Kluwer Health, Inc. A special consideration associated with recovery following use of N2O deserves comment. Diffusion hypoxia is a possibility at the end of N2O administration when the patient breathes air immediately rather than O2 for at least a brief transition period (i.e., 5–10 min) [44–46]. In this case, a large volume of N2O returns to the lungs from the blood. This early rapid inflow of N2O to the lung displaces other gases within the lung. If at this time the patient is breathing air (only about 21% O2) rather than 100% O2, N2O dilutes alveolar O2, further reducing O2 partial pressure from levels found in ambient air. This may cause life‐threatening reductions in arterial oxygenation. Since the major effect is in the first few minutes after discontinuing N2O, the condition can be prevented by administering high‐inspired fractions of O2 at the conclusion of N2O administration rather than allowing the patient to immediately breathe ambient air. Most inhalation anesthetics are not chemically inert [47]. They undergo varying degrees of metabolism, primarily in the liver but also to lesser degrees in the lung, kidney, and intestinal tract [41,48–51]. The importance of this is two‐fold. First, in a very limited way, metabolism may facilitate anesthetic recovery. Second, and more important, is the potential for acute and chronic toxicities by intermediate or end metabolites of inhalation agents, especially involving the kidneys, liver, and reproductive organs [41,51]. The magnitude of metabolism of inhalation anesthetic agents varies greatly and is determined by a variety of factors, including the chemical structure, hepatic enzyme activity (cytochrome P450 enzymes located in the endoplasmic reticulum of the hepatocyte), the blood concentration of the anesthetic [52], disease states, and genetic factors (i.e., some species and individuals are more active metabolizers of these drugs than others, e.g., humans versus rats). An indication of the extent of biotransformation of contemporary inhalation anesthetics is given in Table 28.6. The degradation of sevoflurane occurs in vivo to about the same extent as isoflurane, perhaps a bit more depending on circumstances, and as indicated by transient postanesthetic increases in blood and urinary fluoride levels in rats [53–57], dogs [56], horses [58–60], swine [61], and humans [62]. The peak serum fluoride concentrations observed in humans during and following sevoflurane anesthesia are low, and nephrotoxicity is not expected [62,63]. Desflurane resists degradation in vivo [61,64,65]. The increase in serum inorganic fluoride is much smaller than that found with isoflurane [61,64,65]. For further information on the biotransformation of inhalation anesthetics in general and specific details regarding individual anesthetic agents, readers are referred to reviews by Baden and Rice [41], Mazze and Fujinaga [51], and Njoku et al. [66]. In 1963, Merkel and Eger described what became the standard index of anesthetic potency for inhalation anesthetics, MAC [73]. MAC is defined as the minimum alveolar concentration of an anesthetic at 1 atmosphere that produces immobility in 50% of subjects exposed to a supramaximal noxious stimulus. Thus, MAC corresponds to the effective dose50 or ED50; half of the subjects are anesthetized, and half have not yet reached that “level.” The dose that corresponds to the ED95 (95% of the individuals are anesthetized), at least in humans, is 20–40% greater than MAC [74]. Anesthetic potency of an inhaled anesthetic is inversely related to MAC (i.e., potency = 1/MAC). From information presented earlier, it also follows that MAC is inversely related to the oil/gas PC. Thus, a very potent anesthetic such as methoxyflurane, which has a high oil/ gas PC has a low MAC, whereas an agent with a low oil/gas PC has a high MAC. A number of characteristics of MAC deserve emphasis [17]. First, the “A” in MAC represents alveolar concentration, not inspired or delivered (as, for example, from a vaporizer). This is important because the alveolar concentration is easily monitored with contemporary technology. Also, as we reviewed earlier, after sufficient time for equilibration (minutes), alveolar partial pressure will closely approximate arterial and brain (CNS) anesthetic partial pressures. Second, MAC is defined in terms of volumes percent of 1 atmosphere and therefore represents an anesthetic partial pressure (P) at the anesthetic site of action (i.e., remember Px = (C/100) Pbar, where Px stands for the partial pressure of the anesthetic in the gas mixture, C is the anesthetic concentration in vol % and Pbar is the barometric or total pressure of the gas mixture). Thus, although the concentration at MAC for a given agent may vary depending on ambient pressure conditions (e.g., sea level versus high altitude), the anesthetic partial pressure always remains the same. For example, MAC for isoflurane in healthy cats is reported as 1.63 volumes %. The study reporting this value was conducted at near sea level conditions at Davis, California (i.e., Pbar of 760 mmHg). Based on discussion above, MAC of 1.63% represents an alveolar isoflurane partial pressure (Piso) of 11.6 mmHg. In comparison, for the same dog at Mexico City (elevation 2240 m above sea level; Pbar = 584 mmHg), the alveolar Piso at MAC is expected to be the same as determined at Davis, California (i.e., 11.6 mmHg); however, MAC (i.e., the alveolar concentration) would be about 2.17%. Finally, it is important to note that MAC is determined in healthy animals under laboratory conditions in the absence of other drugs and circumstances common to clinical use that may modify the requirements for anesthesia. General techniques for determining MAC in animals are given elsewhere [17,75–79]. In determining MAC in humans, the initial surgical skin incision has been the standard noxious stimulus used [17]. For the determination of MAC in smaller animals (mice to dogs and pigs) [17,80,81], the standard stimulus has been application of a forceps or other surgical clamp to the base of the tail or the base of the dewclaw of the limb (e.g., pigs [78]), while electrical stimulus applied beneath the oral mucus membranes is most commonly used in larger species such as horses [76]. The MAC values for contemporary inhalation anesthetics for a variety of mammals commonly encountered in veterinary medicine are summarized in Table 28.7A, while the ED50 values for non‐mammalian species are given in Table 28.7B. Values for humans are also given for comparison. For values of agents of historical interest such as methoxyflurane, enflurane, or diethyl ether, readers are referred to the review in an earlier edition of this book or elsewhere [17,81]. Since its original introduction, the MAC concept has been extended to other stimulus endpoints in an effort to better define and understand the anesthetic state. For example, Stoelting et al. [82] determined the value for MAC of an anesthetic at which humans opened their eyes on verbal command during emergence from anesthesia; this has been termed “MAC‐awake.” The verbal stimulus is of course less intense than the surgical incision in humans and thus the response occurs at a lower concentration of anesthetic than movement following incision. The end‐tidal concentration preventing movement in response to tracheal intubation (MAC for intubation) is more stimulating to humans than surgical incision and was described by Yakaitis et al. [83,84]. Roizen et al. [85] reported an even greater alveolar concentration necessary to prevent adrenergic response (rise in endogenous catecholamines) to skin incision (also in human patients) compared to the concentration necessary to just prevent movement; this is known as “MAC–BAR.” Similarly, Boscan et al. showed that ovarian/ovarian ligament traction increased sevoflurane MAC compared to tail clamp in dogs [86]. Thus, a group of response curves is possible and dependent upon the strength of the stimulus applied. In a single species the variability in MAC (response to a noxious stimulus) is generally small and is not substantially influenced by gender, duration of anesthesia, variation of PaCO2 (from 10 to 90 mmHg), metabolic alkalosis or acidosis, variation in PaO2 (from 40 to 500 mmHg), moderate anemia, or moderate hypotension (see Table 28.7A) [17,81,87]. Even between species, the variability in MAC for a given agent is usually not large. However, there is at least one notable exception (see Table 28.7A). In humans, the MAC for N2O is 104%, making it the least potent of the inhalation anesthetics currently used in this species. Its potency in other species is less than half that in humans (i.e., around 200%). Because the N2O MAC is above 100%, it cannot be used by itself at 1 atmosphere pressure in any species and still provide adequate amounts of O2. Consequently, and assuming that MAC values for combinations of inhaled anesthetics are additive, N2O is usually administered with another more potent agent to thereby reduce the concentration of the second agent necessary for anesthesia (see Fig. 28.15). However, because of the potency difference between animals and humans, the amount of reduction differs in an important way. For example, administration of 60% N2O with halothane reduces the amount of halothane needed to produce MAC by about 55% in healthy humans (see Fig. 28.15) but reduces it only by about 20–30% in dogs. As noted in Fig. 28.15, the response of other animals most closely resembles the dog. Some factors that are known to influence MAC are given in Table 28.8. Equipotent doses (i.e., equivalent concentrations of different anesthetics at MAC) are useful for comparing effects of inhalation anesthetics on vital organs. In this regard, anesthetic dose is commonly defined in terms of multiples of MAC (i.e., 1.5‐ or 2.0‐times MAC, or simply 1.5 MAC or 2.0 MAC). From the preceding discussion, therefore, the ED50 equals MAC or 1.0 MAC and represents a light level of anesthesia (clearly inadequate in 50% of otherwise unmedicated, healthy animals). The ED95 is 1.2 to 1.4 MAC, and 2.0 MAC represents a deep level of anesthesia, in some cases even an anesthetic overdose. The authors will use the concept of MAC multiples later to compare drug effects and contrast pharmacodynamics of multiple doses of a specific drug. In concluding this introduction to MAC, a few additional comments are warranted with further details available in recent publications and below in the section on CNS actions. Inhalation anesthetics act in the CNS to cause immobility, analgesia, and unconsciousness. Immobility is now known to reflect the activity of inhalation anesthetics on the spinal cord [88–90], but equi‐MAC may not translate to equivalent potency in the brain, at least when evaluated with regards to analgesic and hypnotic potency [91]. Regardless, and despite the fact that “…MAC has withstood the test of time…,” a recent editorial [92] (from which this quote was taken) in response to a publication in the same issue of the journal Anesthesiology [93] notes, “… in an era of precision medicine, we should use more precise terminology. It is time to call a MAC (minimum alveolar concentration) a MAC (median alveolar concentration).” Table 28.7A MAC values (%) for a variety of mammals at sea level or near sea level conditions. a Absolute value related to strain. Note, some variability within and across species is likely associated with the technique of measurement of alveolar anesthetic concentration [79]. Table 28.7B ED50 values for a variety of non‐mammals at sea level or near sea level conditions. All contemporary inhalation anesthetic agents in one way or another influence vital organ function. Some actions are inevitable and accompany the use of all agents, whereas other actions are a special or prominent feature of one or a number of the agents. In addition, dose–response relationships of inhalation anesthetics are not necessarily parallel. Differences in action, and especially undesirable action, of specific anesthetic agents, form the basis for selecting one agent over another for a particular patient and/or procedure. Undesirable actions also provide primary impetus for development of new agents and/or anesthetic techniques. Data from healthy animals exposed to equipotent alveolar concentrations of these drugs under controlled circumstances provide foundational information for this review. In other cases, results of studies of human volunteers form the basis of our understanding of some drug actions because actions in animals of common clinical focus in veterinary medicine have not been described. Because animals are commonly allowed to breathe spontaneously during clinical management of general anesthesia (versus controlled mechanical ventilation), investigational results obtained from spontaneously breathing test animals are often considered baseline by veterinarians. However, in the broader anesthesiology and pharmacology literature, results of studies from human volunteers or animals administered precise amounts of inhalation anesthetics during controlled ventilation (and normocapnia) most commonly form the basis of comparison of pharmacodynamic differences. It is important to stress that many variables other than mode of ventilation commonly accompany anesthetic management of animals in both clinical and laboratory settings. These variables influence drug pharmacokinetics and pharmacodynamics and may cause individuals to respond differently from test subjects that were studied under standardized conditions. Such confounding variables include species, duration of anesthesia, noxious (painful) stimulation, co‐existing disease, concurrent medications, variation in body temperature, and extremes of age, as examples. Figure 28.15 When N2O is combined with halothane the alveolar concentration of halothane at MAC is decreased. However, the halothane sparing imposed by N2O is less in animals compared to humans. Source: Reproduced with permission from Steffey and Eger [203]. Table 28.8 Some factors that influence the value of MAC (anesthetic requirement). a The list of example drugs is intended to be representative, not exhaustive. The list is summarized from previous reviews [17,75,81] except where indicated by reference numbers. Inhalation anesthetics affect the CNS in many ways. Mostly these agents are selected because they induce a reversible, dose‐related state of CNS (somatic, motor), but also hemodynamic and endocrine unresponsiveness to noxious stimulation, that is, a state of general anesthesia. Interestingly, although clinical anesthesia was introduced more than 150 years ago, the mechanisms and sites by which general anesthetics (including inhalation anesthetics) cause unresponsiveness to surgical or other forms of noxious stimulation remain unknown [221]. Inhalation anesthetics influence electrical activity of the brain, cerebral metabolism, cerebral perfusion, intracranial pressure, and analgesia – issues of critical importance to the anesthetic management of animals. Our systematic review will start with a focus on mechanisms of action of inhalation anesthetics within the CNS. Ever since the first public demonstration of diethyl ether in 1846, the molecular determinants of inhaled anesthetic action have remained elusive [221]. As a class, inhaled anesthetics exhibit unusual actions that set them apart from most other pharmacologic agents. Most drugs rely on a unique molecular size, shape, volume, charge, polarity, functional group, or other structural motif in order to bind with a specific target molecule and induce a functional change, as set forth by Fisher’s Schloss und Schlüssel (lock and key) description of specific enzyme–substrate or protein–drug interactions [222]. Yet inhaled anesthetics share no chemical or structural commonalities; they range in composition from single atoms (xenon) and diatomic elements (nitrogen) to inorganic molecules (N2O) and hydrocarbons of varying lengths containing any number of halogens and organic functional groups, including alkanes (chloroform, halothane), alkenes (trichloroethylene), alcohols (ethanol), ethers (isoflurane, sevoflurane, and desflurane), and combinations thereof (ethyl vinyl ether, fluroxene). Even endogenous by‐products of metabolism such as CO2 [223], ammonia [224], and ketones [225] exert anesthetic effects. Such chemical diversity among drug “keys” is incongruent with classical notions of drug–ligand interactions. Inhaled anesthetic efficacy is not limited to humans and domestic mammals. These drugs can reversibly immobilize all vertebrate and invertebrate animals in which they have been studied, including worms, fish, amphibians, reptiles, and birds. In fact, efficacy is not limited to the animal kingdom, as inhaled anesthetics can even prevent movement in protozoa [226] and plants (those with touch‐sensitive contractile leaves) [227]. It is unknown why these pharmacologic effects should be conserved across such diverse phyla, especially since most anesthetics are not found in nature and there is no obvious selective pressure underlying anesthetic sensitivity. It suggests, perhaps, that the molecular mechanism underlying inhaled anesthetic action involves interactions with cellular components that are essential to all life. Indeed, given that we are surrounded by potential anesthetics – from the nitrogen [228] in the air we breathe to the volatile essential oils [229] in the food we eat – there may have been evolutionary pressure to minimize sensitivity of target sites at the concentrations these “natural anesthetics” are present within the environment. Meyer [230] and Overton [231] noted that narcotic potency correlated with anesthetic solubility in oil from which they concluded that the cell lipid membrane served as the site of anesthetic action. However, non‐specific lipid theories do not adequately explain effect differences between anesthetic stereoisomers [232] or the existence of the anesthetic cut‐off effect and non‐immobilizers, compounds that lack an immobilizing effect at concentrations predicted by the Meyer–Overton hypothesis to produce general anesthesia [233]. More recently, Cantor [234,235] hypothesized that anesthetics may alter molecular composition and forces within a bilayer plane and cause a change in the lateral pressure profile exerted upon proteins within the lipid membrane, a theory that was supported by several computer simulation models [236–239]. However, using an experimental assay to detect changes in lipid bilayer properties, neither isoflurane, halothane, nor cyclopropane was found to produce detectable membrane effects at clinically relevant concentrations [240]. Miller [241] and Pauling [242] each proposed an aqueous site of action whereby the ordering of water into gas hydrates or clathrates alters electrical conductance across the cell membrane to produce unconsciousness. However, anesthetic MAC correlates better with lipid solubility than with hydrate dissociation pressure [243,244]. In addition, enthalpy for anesthetic hydrate formation is much greater than calculated for in vivo hypothermia MAC studies [109], and the extreme transience of anesthetic clathrate formation with reduced water concentration at anesthetic binding sites [245] makes a bulk aqueous phase site of action unlikely. Inhaled anesthetics bind proteins and can modulate their function even in the absence of a lipid environment [246]. Presynaptically, volatile anesthetics inhibit calcium channel function in the terminal bouton of the neuron and bind soluble N‐ethylmaleimide‐sensitive attachment proteins (SNAP) and SNAP receptor (SNARE) and associated proteins to decrease neurotransmitter release [247–255]. The magnitude of these effects is much greater at excitatory glutamatergic synapses than at inhibitory GABA‐ergic synapses, resulting in reduced excitatory neurotransmission relative to inhibitory neurotransmission [256,257]. Inhaled anesthetics also act postsynaptically to potentiate inhibitory cell targets such as γ‐aminobutyric acid type A (GABAA) receptors, glycine receptors, and two‐pore domain potassium channels; these same agents inhibit excitatory cell targets such as N‐methyl‐d‐aspartate (NMDA) receptors, α‐amino‐3‐hydroxy‐5‐methyl‐4‐isoxazole‐propionic acid (AMPA) receptors, neuronal nicotinic acetylcholine receptors, and voltage‐gated sodium channels [221,232,258–264]. Inability to identify a single target critical to anesthetic action may in fact suggest that immobility is the product of multiple cell receptor and ion channel target modulations [265] acting in a combination of in‐parallel and in‐series cell pathways and neuronal circuits to decrease cell excitability [266]. Such a model, in which the anesthetic MAC greatly exceeds the dissociation constant between the anesthetic and its relevant protein targets, also predicts steep dose responses (large Hill coefficients) and additive combinatorial effects that are hallmarks of most inhaled anesthetic actions both in vivo [267] and in vitro [268]. Multiple relevant sites of action would also explain why loss of anesthetic efficacy at one cellular target consequently produces greater anesthetic modulation of other putative cellular targets at MAC [269,270]. How can a single inhaled anesthetic modulate so many different protein targets? At MAC, aqueous phase drug concentrations are around 300 μM or more, depending on the agent, which implies low‐affinity drug binding of protein targets [271,272]. Indeed, any hydrocarbon – not just conventional inhaled anesthetics – appears able to modulate anesthetic‐sensitive ion channels and receptors when present at sufficiently high concentrations, limited only by the molar water solubility of the drug itself. However, according to the Molar Water Solubility Hypothesis, if a hydrocarbon’s saturated aqueous phase concentration is less than a critical cut‐off value specific for a given receptor, then the drug fails to modulate that receptor’s function [273–275]. This suggests that anesthetic binding may occur at allosterically active amphipathic pockets in the protein that are normally occupied by water with a high dissociation constant (kd). When an anesthetic agent is present at sufficiently high concentrations relative to the water kd, the drug displaces the water molecule from this site and induces a change in protein structure and function. Although this implies that access to this amphipathic site is not dependent on drug structure, the type and strength of the subsequent protein–drug molecular interaction – and hence the direction (positive or negative) and magnitude (efficacy) of receptor modulation – certainly would be. Inhaled anesthetics cause immobility, amnesia, and unconsciousness, but these distinct endpoints are achieved via actions at different CNS sites [88–90]. In an experimental goat model in which the cerebral circulation was isolated from the rest of the body, selective brain isoflurane administration more than doubled the anesthetic requirement for immobility compared to whole‐body isoflurane administration. This demonstrated that the spinal cord, and not the brain, is principally responsible for preventing movement during surgery with inhaled anesthetics [89]. Within the spinal cord, immobility is most likely produced by depression of locomotor neuronal networks located in the ventral horn [276]. In contrast, amnestic effects of inhaled anesthetics are produced by actions within the brain, most probably within the amygdala and hippocampus [277]. Lesions created within the amygdala of rats can block amnestic actions of sevoflurane [278]. Patch clamp recordings in vitro of murine amygdala slice preparations also show decreased synaptic transmission at 1 MAC of isoflurane due to inhibition of glutamatergic currents and potentiation of GABAA receptor currents [279]. Moreover, infusion of a selective 5‐HT7 receptor agonist bilaterally into the basolateral amygdala in rats can actually reverse memory impairment caused by sevoflurane [280]. On electroencephalography, hippocampal‐dependent θ‐rhythm frequency slows in proportion to the amnestic effects observed with subanesthetic concentrations of isoflurane in rats [281]. Furthermore, mutant mice lacking the gene encoding either the α4 or β3 subunits of GABAA receptors are resistant to isoflurane depression of hippocampus‐dependent learning and memory [282,283], and antagonism of α5 subunit‐containing GABAA receptors restores hippocampal‐dependent memory during sevoflurane administration [284]. Consciousness in humans is commonly assessed by testing for voluntary responses to verbal commands. In animals, behavioral tests of consciousness include presence of a righting reflex, movement in response to non‐noxious stimuli, and spontaneous interaction with the environment. Cholinergic stimulation of the prefrontal cortex, but not the parietal cortex, causes wakefulness and spontaneous movement in rats breathing 1.9–2.4% sevoflurane. Stimulation of the central medial thalamus via infusions of antibodies against the voltage‐gated K+ channel Kv1.2 restores ambulation and exploring behavior in rats breathing 0.5 MAC sevoflurane or desflurane [285]. Activation of dopaminergic neurons within the ventral tegmental area of the midbrain in rats or mice using electrical or optogenetic stimulation restores the righting reflex during isoflurane or sevoflurane anesthesia [286,287]. Finally, electrical or glutamatergic stimulation of neurons in the parabrachial nucleus of the pons can also cause return of righting in mice anesthetized with isoflurane or sevoflurane [288,289]. Collectively, these findings suggest that volatile anesthetics produce unconsciousness through actions at multiple discrete sites within the pons, midbrain, thalamus, and possibly cerebral cortex. At concentrations above MAC, it is presumed that inhaled anesthetics sufficiently depress cortical function to prevent animals from experiencing motivational‐affective dimensions of pain [290]. Additionally, concentrations of modern volatile agents (halothane, enflurane, isoflurane, sevoflurane, and desflurane) between 0.8 and 1.0 MAC decrease, but do not ablate, wind‐up, and central sensitization and so may help prevent heightened postoperative pain sensitivity; however, higher concentrations of contemporary drugs offer no further benefit in this regard [291,292]. Volatile anesthetic concentrations between 0.4 and 0.8 MAC decrease withdrawal responses to noxious stimuli, but lower concentrations can actually cause hyperalgesia with a peak effect at 0.1 MAC [293,294] due to potent nicotinic cholinergic receptor inhibition [295]. In contrast, the gaseous anesthetics xenon and N2O produce analgesia via glutamatergic receptor inhibition [296] and, in the case of N2O, through additional modulation of noradrenergic‐opioid receptor pathways [297]. Anatomically, analgesic actions occur both supraspinally and within the dorsal horn of the spinal cord and are likely responsible for greater blunting of autonomic responses associated with these gases compared to the modern volatile agents [298]. The electroencephalogram (EEG) is used to help identify pathologic brain disorders and predict outcome of brain insults. Studies have shown that general anesthesia alters EEG parameters; however, all anesthetics do not produce exactly the same changes in EEG pattern as dose (anesthetic depth) increases; therefore, the generic correlation of the raw EEG with anesthetic dose is not precise. Indeed, despite some weak correlations and its usefulness as an indication of changing anesthetic depth, no parameter has had sensitivity and specificity sufficient to justify use of the EEG alone as a reliable index of anesthetic depth [299]. With technological advances in recent years, research has focused on use of processed EEG parameters (e.g., the bispectral index) as improved descriptions of anesthetic states. Additional information on this topic is available in Chapter 10. In general, as the depth of anesthesia increases from awake states, the electric activity of the cerebral cortex becomes desynchronized. Using isoflurane as an example of the general EEG response to volatile anesthetics [1], there is initially an increased frequency of the EEG activity (alveolar concentrations less than 0.4 MAC). With further increases in anesthetic concentration, a decrease in frequency and increased amplitude of the EEG waves occur. The wave amplitude increases to a peak (about 1.0 MAC) and then, with further dose increase, it progressively declines (burst suppression occurs at about 1.5 MAC, that is, bursts of slow high‐voltage activity separated by electrical silence) and eventually becomes flat line. With isoflurane, an isoelectric pattern occurs at about 2.0 MAC, while at the other extreme, it is not seen with halothane until > 3.5 MAC. Electrical silence does not occur with enflurane. The two newest volatile anesthetics, sevoflurane and desflurane, cause dose‐related changes similar to those of isoflurane [300–302]. Systematic studies of EEG activity in humans [303] and dogs [304] showed that enflurane was associated with spontaneous or noise‐initiated intensified seizures. In addition, enflurane induces seizure activity that is associated with substantial increases in cerebral blood flow and cerebral metabolic use of O2. The EEG responses of desflurane, sevoflurane, and isoflurane are similar [300–302] and all three can suppress drug‐induced convulsive behavior [305–309]. However, there are reports of seizure activity in animals [119,310] and human patients [311,312] during sevoflurane anesthesia. All volatile anesthetics decrease cerebral metabolic rate (cerebral oxygen consumption [CMRO2]). The magnitude of decrease is least with halothane but similar with isoflurane, sevoflurane, and desflurane [119,300,305,313,314]. Anesthetic goals for patients having, or at risk for, intracranial hypertension should include reduction of intracranial pressure (ICP), maintenance of cerebral perfusion pressure (CPP), and matching cerebral blood flow (CBF) to a reduced CMRO2. According to the Monro–Kellie doctrine [315,316], three volumes are contained within the calvarium: brain parenchyma, cerebrospinal fluid, and blood; an increase in volume of one component will increase ICP unless compensated by a decrease in one or both of the other intracranial component volumes. Most inhaled agents cause cerebral vasodilation, thereby increasing brain blood volume and ICP. This effect is greatest with halothane, less with enflurane, isoflurane, and desflurane, and least with sevoflurane [317,318]. The gas anesthetics currently in use have divergent effects; N2O causes more cerebral vasodilation than equipotent doses of volatile anesthetics [319], whereas xenon has little effect on ICP and cerebral vascular tone [320]. CPP is defined as the difference between mean arterial blood pressure (MAP) and ICP; it represents the driving pressure for blood flow to the brain. The potent volatile agents depress cardiovascular function and decrease MAP, and thus decrease CPP [321]. Nonetheless, CBF typically increases due to dose‐dependent cerebral vasodilation that reduces vascular resistance [322], despite dose‐dependent reductions in EEG activity and CMRO2. Through possible actions on nitric oxide synthase [323–326], cyclo‐oxygenase, [323], adenylate cyclase [327], and ATP‐sensitive K+ channels [328], vasodilation caused by potent volatile anesthetics leads to a loss of cerebrovascular autoregulation, reflected by an uncoupling of cerebral perfusion from cerebral oxygen demand. Increased CBF from N2O is accompanied by an actual increase in metabolic rate of the cerebral cortex if administered alone [329], but not when co‐administered with a potent volatile agent such as isoflurane [330,331]. Xenon minimally affects CMRO2 [332] and preserves normal cerebrovascular autoregulation [333]. In addition to specific agent effects, anesthetic modulation of cerebral hemodynamics depends upon agent dose, anesthetic time, animal species studied, and hyper‐ or hypoventilation. At 0.5 MAC, cerebrovascular autoregulation during anesthesia with potent inhaled agents is slowed or obtunded; however, at concentrations greater than MAC, cerebrovascular autoregulation is significantly and progressively diminished as CBF becomes simply a function of CPP [334,335]. Blood flow also increases throughout most of the brain as a function of anesthetic duration, presumably due to time‐dependent increases in nitric oxide synthase inhibition [336]. Carbon dioxide tension further affects autoregulation. Although halothane tends to increase CBF regardless of ventilation status [337], cerebrovascular autoregulation may be better preserved at a given MAC‐multiple of a haloether anesthetic when hyperventilation is maintained concurrently [338]. The converse also appears true: autoregulation is lost and CBF is increased at lower inhaled anesthetic doses when animals are allowed to hypoventilate [339], although this interaction between CO2 and agent is greater with isoflurane than for sevoflurane [340]. Although most anesthetic effects on cerebral hemodynamics are similar among humans and small animal research models, the same does not hold true for horses, and perhaps other very large animal species. Intracranial pressure (ICP) in awake horses remains constant irrespective of head position [341], but cerebral vasodilation during inhalant anesthesia causes large increases in ICP – sometimes similar in magnitude to values seen with severe head trauma in human patients – that is exacerbated by dorsal recumbency, head‐down positioning, hypercapnia during spontaneous ventilation, and anesthetic time during controlled ventilation [342–345]. Surprisingly, over a wide range of perfusion pressures, isoflurane‐anesthetized horses maintain regional CBF relatively constant, albeit at a low flow that may still place animals at risk for tissue hypoxia [346]. Blood flow to the thoracolumbar spinal cord is particularly low, and further reduction may predispose to postanesthetic myelomalacia in horses [347]. Inhalation anesthetics depress respiratory system function. The volatile agents in particular decrease ventilation in a drug‐ and species‐specific manner. Depending on conditions, including species of interest, some of the most commonly considered measures of breathing effectiveness, that is, breathing rate and depth (tidal volume), may not be revealing or may even be misleading. In general, spontaneous ventilation progressively decreases as inhalation anesthetic dose is increased because at low doses tidal volume decreases more than frequency increases. As anesthetic dose is further increased, respiratory frequency also decreases. In otherwise unmedicated animals (including humans) anesthetized with volatile agents, respiratory arrest occurs at 1.5–3.0 MAC (Table 28.9). The overall decrease in minute ventilation and the likely variable increase in deadspace ventilation (resulting in an increase in the deadspace to tidal volume ratio, VD/VT, from a normal of about 0.3 to 0.5 or more) result in a reduction in alveolar ventilation. Decreases in alveolar ventilation are out of proportion to decreases in CO2 production (O2 utilization is decreased by general anesthesia), such that PaCO2 increases (Fig. 28.16). In addition, the normal stimulation of ventilation caused by increased PaCO2 (or decreased PaO2) is depressed by the inhalation anesthetics, presumably via the action of these agents directly on the medullary and peripheral (aortic and carotid body) chemoreceptors [348–351]. Bronchospasm is associated with some conditions that contribute to increased airway resistance. A variety of early studies indicated that among anesthetics available at the time, halothane was the most effective bronchodilator [352,353]. That effect is believed to result, at least partially, from decreased cholinergic neurotransmission [354,355]. Therefore, it was often recommended for patients at risk of bronchospasm. The work of Hirschman et al. suggests that isoflurane and perhaps enflurane were as effective in decreasing experimentally produced airway resistance and therefore were good alternatives to halothane [356,357]. Recent work with isoflurane, sevoflurane, and desflurane indicates that relaxation of constricted bronchial muscles by these agents at least equals or exceeds that caused by halothane [355,358,359]. Avoiding airway irritation during administration of inhalation anesthetics is important, especially during induction of anesthesia because it may cause breath holding, coughing, and laryngospasm (particularly in some species such as primates, both human and nonhuman) that in turn result in arterial oxyhemoglobin desaturation. At least in humans, none of the potent inhalation anesthetics seem to have irritant properties at subanesthetic concentrations. However, patient objection and airway irritation are evident with desflurane (and to a lesser degree with isoflurane [360]) at concentrations of 7% or greater [361,362] and as a result, desflurane is not commonly used for anesthetic induction of human patients. The PaCO2 is the most frequently used measure of respiratory system response to general anesthetics. All contemporary inhalation anesthetics depress alveolar ventilation and increase PaCO2 in dose‐related fashion. Fig. 28.16 summarizes the effects of inhalation anesthetics in humans, the species for which data are most complete [349,363–367]. Because appropriate data in the species of interest to veterinary anesthetists are incomplete, rank order of the magnitude of hypoventilation caused by the four contemporary volatile anesthetics at a common alveolar dose cannot be expressed with authority. Indeed, species variability is an important confounder (see below). Figure 28.16 Respiratory response to an increase in the alveolar concentration (expressed as a multiple of MAC) of inhalation anesthetics in humans. Source: Data are taken from multiple sources Fourcade et al. [349]; Lockhart et al. [363]; Munson et al. [364]; Larson et al. [365]; Doi and Ikeda [366]; Calverley et al. [367]. Table 28.9 Apneic Index (AI) in various species. Minimum alveolar concentration (MAC) is given in vol % and the AI is a ratio of the end‐tidal anesthetic concentration at apnea and MAC. Similar data are not currently available for sevoflurane. NA, not available. Ventilation is often assisted or controlled during inhalation anesthesia to compensate for the anesthetic‐induced respiratory depression. Controlled mechanical ventilation is used to predictably maintain a normal or some other specific PaCO2, during anesthesia. Assisted ventilation (i.e., the anesthetist augments tidal volume, but the animal determines its own breathing frequency) is used to attempt to improve the efficiency of oxygenating arterial blood and reduce the work of breathing but is usually not effective in substantially lowering PaCO2 compared to circumstances associated with spontaneous ventilation (i.e., the animal controls both the rate and depth of breathing) [370,371]. Respiratory function, including PaCO2, is little changed for as long as up to 10 h of constant, low‐dose halothane (or methoxyflurane) dogs [372,373]. This is also supported by studies of humans [374] anesthetized with constant low‐dose halothane. However, in horses anesthetized for 5 h with a constant dose of 1.2 MAC isoflurane, a substantial temporal increase in PaCO2 was noted [375]. A similar but more modest trend was noted in horses when halothane was used for anesthesia [376, 377]. At least in some species, if the alveolar dose of halothane is increased above about 1–1.3 MAC and maintained constant at a heightened level, the magnitude of change in hypoventilation also worsens with time [373]. Conversely, there is evidence for recovery from the ventilatory depressant effects of volatile anesthetics in humans [367]. Noxious stimulation may result in sufficient central nervous stimulation to lessen the ventilatory depression of inhalation anesthetics [378–381]. This effect is diminished with increasing anesthetic depth. In humans, the substitution of N2O for an equivalent amount of a concurrently administered volatile agent such as isoflurane results in a lower PaCO2 than that seen with the volatile agent alone [26]. However, in dogs and monkeys anesthetized with halothane, ventilation was at least as, and sometimes more, depressed when N2O was substituted for a portion of the halothane [382,383]. The addition of opioid drugs such as morphine may increase the respiratory depression produced by an inhalation anesthetic [116,131,132,380]. All volatile inhalation anesthetics cause dose‐dependent and drug‐specific changes in cardiovascular performance. The magnitude and sometimes direction of change may be influenced by other variables that often accompany general anesthesia (Box 28.4). The mechanisms of cardiovascular effects are diverse but often include direct myocardial depression and a decrease in sympathoadrenal activity. All of the volatile anesthetics can decrease cardiac output (CO). The magnitude of change is dose‐related and dependent upon agent. In general, among the contemporary agents in use with animals, halothane is most depressing to CO [1,384–386]. Desflurane in many ways is similar in cardiovascular action to isoflurane while sevoflurane has characteristics resembling both halothane and isoflurane. All of the newer volatile anesthetics tend to preserve CO at clinically useful concentrations, facilitated by reductions in systemic vascular resistance [128,361,387–394]. However, the direct effect of volatile agents on CO (if vascular resistance is maintained) is often a decrease in stroke volume as a result of dose‐related depression in myocardial contractility [1,128,361,391,395–397].
28
Inhalation Anesthetics
Introduction
Physicochemical characteristics
Chemical characteristics
Property
Desflurane
Enflurane
Halothane
Isoflurane
Methoxyfluranea
Nitrous oxide
Sevoflurane
Molecular weight (g)
168
185
197
185
165
44
200
Liquid specific gravity (20 °C) (g/mL)
1.47
1.52
1.86
1.49
1.42
1.42
1.52
Boiling point (°C)
23.5
57
50
49
105
‐89
59
Vapor pressure (mmHg) at:
20 °C
700b
172
243
240
23
–
160
24 °C
804
207
288
286
28
–
183
mL vapor/mL liquid at 20 °C
209.7
197.5
227
194.7
206.9
–
182.7
Preservative
None
None
Yes
None
Not available
None
Yes
Stability in:
Soda lime
No
No
No
No
No
Yes
No
Ultraviolet light
Yes
Yes
No
Yes
No
Yes
??
Physical characteristics
Properties determining methods of administration
General principles: a brief review
Gas versus vapor
Solvent
Desflurane
Enflurane
Halothane
Isoflurane
Methoxyflurane
Nitrous oxide
Sevoflurane
Water
–
0.78
0.82
0.62
4.50
0.47
0.60
Blood
0.42
2.00
2.54
1.46
15.00
0.47
0.68
Olive oil
18.70
96.00
224.00
91.00
970.00
1.40
47.00
Brain
1.30
2.70
1.90
1.60
20.00
0.50
1.70
Liver
1.30
3.70
2.10
1.80
29.00
0.38
1.80
Kidney
1.00
1.90
1.00
1.20
11.00
0.40
1.20
Muscle
2.00
2.20
3.40
2.90
16.00
0.54
3.10
Fat
27.00
83.00
51.00
45.00
902.00
1.08
48.00
Species
Desflurane
Halothane
Isoflurane
Methoxyflurane
Sevoflurane
Nitrous oxide
Cat
0.58
–
1.40
26.4
0.59
–
Cow
0.44
2.40 [10]
1.22
11.3
0.52
–
Dog
0.63
3.51 [10]
1.40
26.1
0.66
0.43 [11]
Goat
0.52
–
1.37
13.0
0.56
–
Horse
0.54
1.77 [10]
1.13
13.0
0.65
–
Pig
0.50
–
1.07
11.1
0.52
–
Rat
0.61
6.56 [12]
1.41
17.7
0.74
–
Rabbit
0.72
4.36 [13]
1.37
25.0
0.70
–
Sheep
0.50
–
1.24
13.2
0.56
–
Human
0.50
2.54 [12]
1.32
14.3
0.64
0.41 [11]
Solvent
Desflurane
Enflurane
Halothane
Isoflurane
Methoxyflurane
Nitrous oxide
Sevoflurane
Reference
Rubber
–
74
120
62
630
1.2
–
[1]
19
–
190
49
–
–
29
[15]
Poly(vinyl chloride)
–
120
190
110
–
–
–
[1]
35
–
233
114
–
–
69
[15]
Poly(ethylene)
–
~2
26
~2
118
–
–
[1]
16
–
128
58
–
–
31
[15]
Methods of description
Vapor pressure
Boiling point
Calculation of anesthetic concentration delivered by a vaporizer
Properties influencing drug kinetics: solubility
Solubility of gases
Blood/gas partition coefficient
Oil/gas partition coefficient
Other partition coefficients
Pharmacokinetics: uptake and elimination of inhalation anesthetics
Anesthetic uptake: factors that determine the PA of anesthetic
Delivery to the alveoli
Inspired concentration
Factor
Vaporizer positioning
Out of circuit
In circuit
Increase ventilation
Decrease
Increase
Increase fresh gas (O2) inflow to circuit
Increase
Decrease
Alveolar ventilation
Removal from alveoli: uptake by blood
Solubility
Cardiac output
Alveolar to venous anesthetic partial pressure difference
Overview
Anesthetic elimination
Biotransformation
Anesthetic dose: the minimum alveolar concentration (MAC)
Species
Desflurane
Halothane
Isoflurane
Sevoflurane
Nitrous oxide
Cat
9.79 [94]
10.27 [95]
0.99 [96, 97]
1.14 [98]
1.19 [99]
1.28 [100]
1.50 [97]
1.61 [99]
1.63 [101]
1.90 [95]
2.21 [102]
2.58 [103]
3.07 [97]
3.41 [95]
255 [98]
Cow
0.76 [104] (calf)
1.14 [105]
223 [104] (calf)
Dog
7.2 [106]
7.68–8.19 [107]
10.3 [108]
0.86 [109]
0.87 [98,110,111]
0.89 [112, 113]
0.92 [114]
0.93 [115]
1.28 [101]
1.30 [116]
1.31 [117]
1.39 [101]
1.39–1.50 [118]
2.10 [119]
2.36 [113]
188 [110]
222 [98]
Ferret
1.01 [120]
1.52 [120]
1.74 [121]
267 [120]
Goat
1.29 [122]
1.3 [123]
1.2 [89]
1.23 [124]
1.29 [122]
1.31 [125]
1.43 [126]
1.5 [123]
2.33 [122]
Horse
7.02 [127]
8.06 [128]
0.88 [76]
0.95 [129]
1.02 [130]
1.05 [131]
1.31 [76]
1.43 [132]
1.44 [133]
1.64 [134]
2.31 [135]
2.84 [60]
205 [136]
Monkey
0.89 [98]
1.15 [137]
1.28 [137]
1.46 [116]
200 [98]
Mouse
6.6–9.1a [138]
0.95 [139]
1.00 [140]
1.19–1.37a [138]
1.31–1.77a [138]
1.35 [139]
1.41 [140]
2.7 [141]
150 [142]
275 [140]
Pig
10.00 [78]
0.90 [143]
0.91 [144]
1.25 [145]
1.45 [146]
1.48 [143]
1.51 [116]
1.55 [147]
1.75 [148]
2.04 [78]
1.97 [149]
2.12 [143]
2.53 [150]
2.66 [151]
162 [147]
195 [148]
277 [145]
Rabbit
8.90 [106]
0.80 [152]
0.82 [153]
1.05 [154]
1.39 [155]
1.42 [156]
1.44 [157]
1.56 [158]
2.05 [155]
2.07 [156]
2.12 [157]
3.70 [159]
Rat
5.72 [160]
6.48 [161]
6.85 [162]
7.10 [163]
0.81 [164]
0.95 [165]
1.02 [166]
1.03 [139]
1.10 [167]
1.11 [168]
1.13 [169, 170]
1.17 [171]
1.23 [162]
1.17 [164]
1.28 [172]
1.30 [173]
1.38 [168]
1.46 [139]
1.46 [162]
1.58 [121, 165]
2.99 [166]
2.40 [167]
2.50 [53]
136 [174]
155 [175]
204 [176]
221 [177]
235 [177]
Sheep
0.97 [178]
1.58 [178]
Human (30–60 years)
6.00 [179]
0.73 [180]
0.74 [181, 182]
0.77 [183]
1.15 [184]
1.58 [185]
1.71 [186]
1.83 [187]
1.84 [188]
1.85 [189]
1.9 [190]
2.05 [159]
104 [191]
Desflurane
Halothane
Isoflurane
Sevoflurane
Nitrous oxide
Birds:
Chicken
0.85 [77]
1.15 [192]
Cockatoo
1.44 [193]
Crane
1.34 [194]
Duck
1.04 [195]
1.30 [195]
Guinea fowl
1.45 [196]
2.9 [197]
220 [196]
Hawk
2.05 [198]
Parrot, Amazon
1.47 [193]
Parrot, African Gray
1.91 [193]
Parrot, thick‐billed
1.07 [199]
Pigeon
1.51 [196]
1.45 [200]
154 [196]
Other:
Goldfish
0.76 [201]
Toad
0.67 [202]
82.2 [202]
Pharmacodynamics: actions and toxicity of the volatile inhalation anesthetics on body systems
No change
Increase
Decrease
Arterial blood pressure > 50 mmHg [204]
Atropine, glycopyrrolate, scopolamine [96]
Duration of anesthesia
Gender
Hyperkalemia, hypokalemia
Metabolic acid‐base change
PaO2 > 40 mmHg
PaCO2 15–95 mmHg
Drugs causing CNS stimulation:
Amphetamine
Ephedrine
Morphine (horse) [131]
Laudanosine [158]
Physostigmine [205]
Hyperthermia (to 42 °C)
Drugs causing CNS depressiona:
Other inhaled anesthetic; N2O [26]
Injectable anesthetics:
Ketamine [206]
Lidocaine [102, 115]
Thiopental [207]
Preanesthetic medications:
Acepromazine [208–210]
Diazepam [211–213]
Detomidine [133]
Fentanyl [214]
Medetomidine [215, 216]
Meperidine [217]
Midazolam [218]
Morphine [219]
Xylazine [134]
Other:
Adenosine
Central anticholinergic [205]
5‐HT antagonist [220]
Arterial blood pressure < 50 mmHg
Hyponatremia
Hypothermia
Increasing adult age
PaO2 < 40 mmHg
PaCO2 > 95 mmHg
Pregnancy
Central nervous system
Mechanism of action
Molecular mechanisms of action
Background
Molecular sites: lipid and aqueous theories
Molecular sites: protein
Anatomic sites of action
Electroencephalographic effects
Cerebral metabolism
Cerebral blood flow and cerebral perfusion pressure
Intracranial pressure
Respiratory system
Arterial carbon dioxide tension
Species
Desflurane
Halothane
Isoflurane
MAC
AI
MAC
AI
MAC
AI
Cat
NA
NA
NA
NA
1.63
2.4 [101]
Dog
7.2
2.4 [361]
0.87
2.9 [111]
1.28
2.5 [101]
Horse
NA
NA
0.88
2.6 [76]
1.31
2.3 [76]
Pig
9.8
1.6 [361, 368]
NA
NA
NA
NA
Rat
NA
NA
1.11
2.3 [369]
1.38
3.1 [176]
Human
7.25
1.8 [363]
0.77
2.3 [363]
1.15
1.7 [363]
Factors influencing respiratory effects
Mode of ventilation
Duration of anesthesia
Surgery and other noxious stimulation
Concurrent drugs
Cardiovascular system
Cardiac output
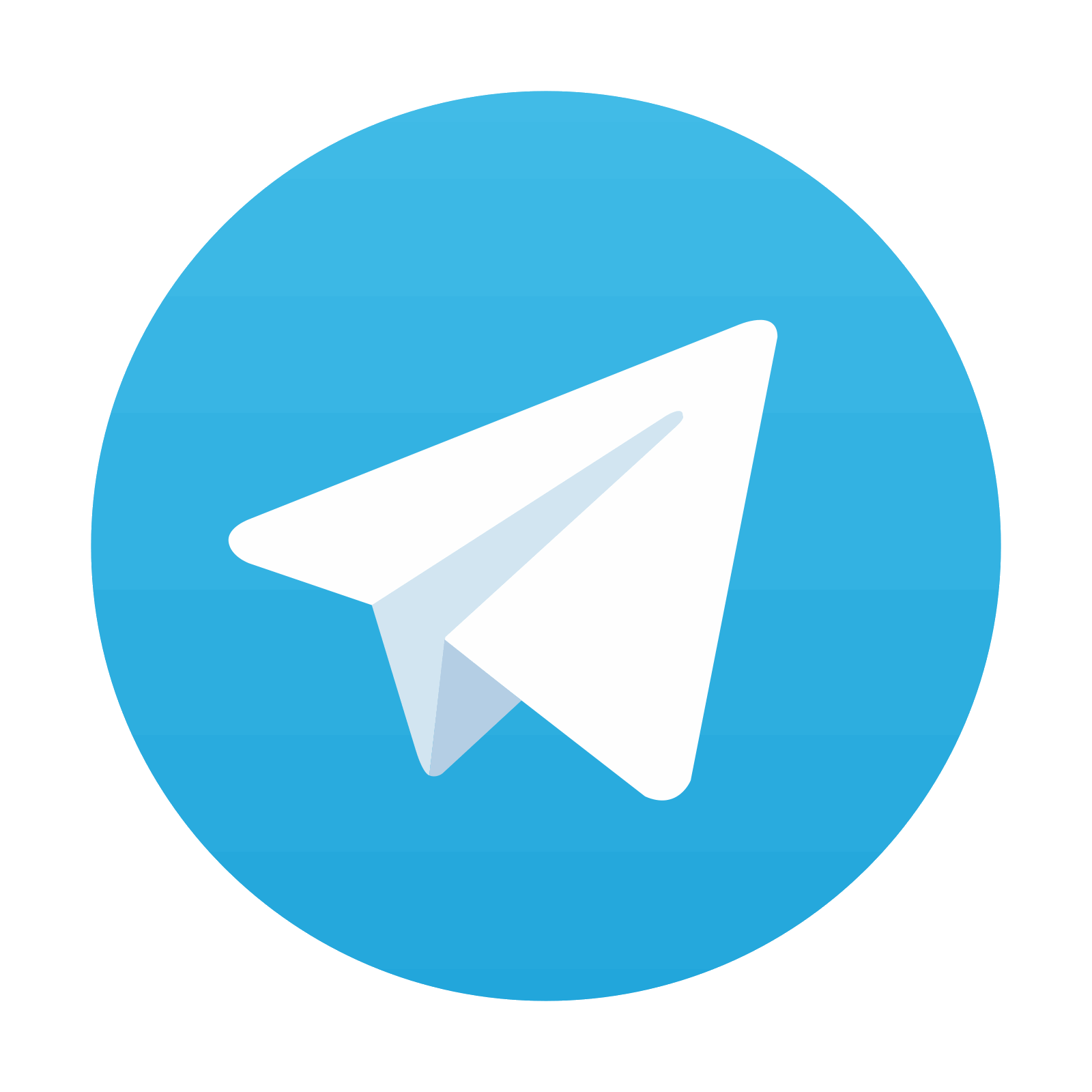
Stay updated, free articles. Join our Telegram channel
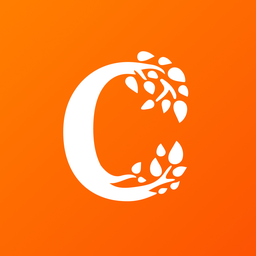
Full access? Get Clinical Tree
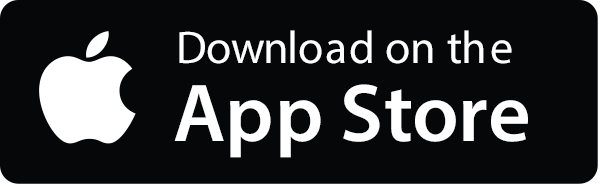
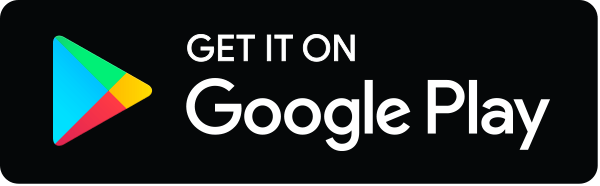