HuiChu Lin Department of Clinical Sciences, College of Veterinary Medicine, Auburn University, Auburn, AL, USA When general anesthesia is considered in farm animal species, factors that affect the decision between injectable and inhalation anesthesia include the type of procedure to be performed, anticipated duration of the procedure, inhalation anesthetic equipment availability, familiarity of the veterinarians with the anesthetic technique, and anesthetic costs. Injectable anesthesia has the advantages of easy drug administration and relatively low cost of specific anesthetic equipment or accessories required for delivery and monitoring. Delivery of an inhalation anesthetic requires expensive and specialized equipment and accessories to be able to deliver the anesthetic to the patient. However, accumulation of injectable anesthetics in the patient following repeated dosing or extended infusion required for completion of a procedure can result in delayed drug elimination and prolonged recovery. Because of the ease and speed in adjusting the depth of anesthesia with the currently available inhalation anesthetics (e.g. isoflurane, sevoflurane, and desflurane), inhalation anesthesia is often preferred for use in long procedures and also for patients that are considered at higher anesthetic risk as a result of preexisting systemic conditions. Isoflurane was first synthesized in 1965 and subsequently became a popular inhalation anesthetic in human patients in 1981. It is still a very commonly utilized inhalation anesthetic in veterinary practice. Sevoflurane was synthesized in the early 1970s. At that time, it was difficult to synthesize the drug and therefore it was expensive to manufacture. It was not until the late 1980s that sevoflurane was introduced in Japan and was later marketed in the USA in 1995 [1]. Special preanesthetic considerations and preparations with respect to laryngeal and gastrointestinal anatomy and physiology prior to general anesthesia in farm animal species are discussed in detail in Chapter 1. The goal of delivering surgical anesthesia with an inhalation anesthetic is to maintain a constant and optimal partial pressure of the anesthetic in the alveoli of the lungs and central nervous systems (CNS). Currently available inhalation anesthetics, isoflurane, sevoflurane, and desflurane, have low blood solubility (isoflurane 1.46, sevoflurane 0.68, desflurane 0.42) and minimal hepatic metabolism (isoflurane 0.2%, sevoflurane 3–5%, desflurane 0.02%) [2]. Blood solubility of an inhalation anesthetic is referred to as blood–gas partition coefficient, which is the ratio of the distribution of an inhalation anesthetic between the blood and the gas. The partition coefficient is the concentration ratio of an anesthetic between the solvent and gas phase, for example blood and gas, or between two tissue solvents, for example brain and blood. As an example, an inhalation anesthetic with a blood–gas partition coefficient of 10 at equilibrium (i.e. the partial pressure of that anesthetic is identical in the blood and gas phases) implies that the concentration of that anesthetic is 10 in the blood and 1 in the gas phase. An anesthetic with a lower blood solubility has a lower blood–gas partition coefficient. In other words, a smaller amount of the anesthetic is dissolved in the blood than in the gas phase when their partial pressures reach equilibrium. Therefore, the speed of achieving surgical plane of anesthesia, the rate of anesthetic depth variation, and the time until consciousness is returned and recovery is achieved are normally faster for an inhalation anesthetic with a lower blood solubility than anesthetics with higher blood solubility. In this case, the time of induction to surgical plane of anesthesia and the time to recovery of full motor function of a patient are faster with desflurane (0.42), intermediate with sevoflurane (0.69), and slower with isoflurane (1.41) [2]. Under ideal conditions, the partial pressure of an anesthetic from the alveoli (P A) to the pulmonary arterial blood (P a) and then to the brain (P br) should be close, if not equal, to the inspired anesthetic partial pressure (P I), that is, P I ≈ P A ≈ P a ≈ P br, at equilibrium. Changes in P I, alveolar ventilation, and the characteristics of an anesthetic breathing system will affect the uptake of the anesthetic from the inspired anesthetic gas flow delivered by the anesthesia machine to the alveoli. Variation of the blood–gas partition coefficient of the anesthetics, the cardiac output of the patients, and the alveoli to venous partial pressure difference affect the transfer of an inhalation anesthetic from the alveolar tissues to pulmonary arterial blood. Factors that determine how quickly the inhalation anesthetic accumulates in the brain include the brain–blood partition coefficient, the cerebral blood flow, and the cerebral arterial to venous partial pressure difference. Because the brain–blood partition coefficients for isoflurane (1.6) and sevoflurane (1.7) are very similar, there should be no significant difference in the speed of the transfer from blood to brain between these two anesthetics [1]. Therefore, increasing P I and alveolar ventilation and reducing anesthesia breathing system volume decreases the time of the partial pressure of an inhalation anesthetic to equilibrium between alveoli and pulmonary arterial blood, and therefore results in a faster induction of anesthesia for that anesthetic. Vice versa, decreasing P I and alveolar ventilation and increasing the volume of the anesthesia breathing system prolongs the time to equilibrium and results in a slower induction of anesthesia. Furthermore, anesthetics with a low blood–gas partition coefficient, patients with a low cardiac output, and small differences between alveolar and venous partial pressures tend to result in faster induction than an anesthetic with greater blood–gas partition coefficient, patients with an increased cardiac output, and larger differences between alveolar and venous partial pressures. Similarly, an anesthetic with a low brain–blood partition coefficient, patients with increased cerebral blood flow, and small differences between cerebral arterial and venous partial pressures tend to result in a faster induction than those with a greater brain–blood partition coefficient, patients with decreased cerebral blood flow, and larger differences between cerebral arterial and venous partial pressures. These tend to result in a slower induction of anesthesia [1, 3]. Return of consciousness and recovery from inhalation anesthesia is an inverse process of induction, which is a result of the elimination of the anesthetic from the CNS. Therefore, factors that affect the speed of induction, for example alveolar ventilation, cardiac output, and blood and tissue solubility, also affect the speed of recovery. In the presence of normal alveolar ventilation and cardiac output, recovery is generally faster for an anesthetic with lower blood and tissue solubility. Hence, recovery from desflurane (0.42) and sevoflurane (0.69) tends to be faster than from isoflurane (1.41). Isoflurane and sevoflurane are the two most commonly used inhalation anesthetics in current veterinary practice (Figures 6.1 and 6.2). Both anesthetics can be used safely and effectively for general anesthesia in farm animal species. Desflurane is a newer inhalation anesthetic with a chemical structure similar to isoflurane with the exception of the substitution of a fluorine for the chlorine on the alpha‐ethyl carbon. Desflurane is unique among the conventional inhaled anesthetics. It has a vapor pressure of 681 mmHg at 20 °C, which is very close to the atmospheric pressure (760 mmHg) and a boiling point of 22.8 °C. At normal operating room temperature, desflurane can boil with a saturated vapor concentration of 87% (681 mmHg/760 mmHg), which is approximately 10 times the minimum alveolar concentration (MAC) of desflurane for humans (6.6%). Because of these unique characteristics, desflurane requires a specially designed vaporizer that is pressurized and heated to provide precise control of anesthetic output from the vaporizer and prevent over anesthetizing the patient [4]. Because of this special vaporizer requirement, desflurane has not been used as commonly as isoflurane and sevoflurane. Isoflurane and sevoflurane require a standard vaporizer calibrated specifically for each anesthetic. Compared to older inhalation anesthetics, isoflurane and sevoflurane have lower potency with MAC values of 1.29% and 2.33%, respectively [5]. The MAC value of an inhalation anesthetic is the minimum alveolar anesthetic concentration required to prevent gross purposeful movement in 50% of patients in response to obnoxious stimuli such as surgical incision. The MAC value is used as an indicator of the potency of the anesthetic. The higher the MAC value, the lower the potency of the anesthetic. From the physico‐chemical properties of the inhalation anesthetics, MAC values are inversely related to the blood solubility of the anesthetic. Therefore, an inhalation anesthetic with high potency normally has a high blood solubility but a low MAC value. On the contrary, an inhalation anesthetic with low potency generally has a low blood solubility but a high MAC value [1]. Sevoflurane is less potent than isoflurane, as reflected in the higher MAC values (2.33% vs. 1.29%), and lower blood solubility (0.69 vs. 1.41). One should always keep in mind that at 1 MAC (1 × MAC), only 50% of patients will not respond to obnoxious stimulation. In other words, the remainder of the patients may respond to an obnoxious stimulation, resulting in gross purposeful movement. In general, surgical anesthesia requires maintaining anesthetic concentration at 1.3 MAC to prevent 95% of the patients from responding to surgical stimulation. Thus, 1.3 MAC of an inhalation anesthetic is referred to as ED95 or surgical anesthesia [1, 3]. For very painful procedures, 1.5 MAC of an inhalation anesthetic concentration may be required to maintain surgical anesthesia. The MAC value is measured and determined in normal healthy patients anesthetized with that inhalation anesthetic alone with no other CNS‐depressing drugs such as tranquilizers, sedatives, analgesics, and injectable anesthetics administered at the time of measurement. The MAC values can be influenced by concurrent administration of anesthetic‐related drugs, alterations of the physiological conditions of the patient, or concurrent administration of medications for the treatment of other conditions or illness. Factors that may increase the MAC value of an inhalation anesthetic for a particular patient include hyperthermia (fever), hypernatremia, and drug‐induced increased CNS catecholamine levels. Increased age, hypothermia, hyponatremia, pregnancy, and concurrent administration of tranquilizers, sedatives, analgesics, injectable anesthetics, local anesthetics, neuromuscular blocking drugs, and drugs that decrease CNS catecholamine levels tend to decrease the MAC value. Duration of anesthesia and magnitude of individual anesthetic metabolism have no effect on the MAC value [6, 7]. Table 6.1 summarizes the blood–gas partition coefficient, metabolism, and MAC values of isoflurane, sevoflurane, and desflurane in farm animal species. Figure 6.1 Anesthesia machine with isoflurane vaporizer. Figure 6.2 Anesthesia machine with sevoflurane vaporizer. Eger [23] and Malan et al. [24] reported that the circulatory effects produced by desflurane closely resemble those produced by isoflurane. Sevoflurane‐induced circulatory effects have the characteristics of both isoflurane and halothane. In humans, isoflurane and sevoflurane produce a dose‐dependent decrease in mean arterial pressure primarily due to the decrease in systemic vascular resistance produced by these anesthetics. Heart rate tends to increase up to 1 MAC (1.29%) during isoflurane anesthesia, while heart rate does not increase until the concentration of sevoflurane anesthesia is greater than 1.5 MAC (1.5 × 2.3% = 3.5%). An abrupt increase in alveolar concentration of isoflurane from 0.55 to 1.66 MAC produces an increase in sympathetic nervous system and renin–angiotensin activities. As a result, transient increases in heart rate and mean arterial blood pressure occur, which may cause the anesthetist to misinterpret insufficient anesthetic depth and further increase the anesthetic concentration delivered to the patient [25]. The transient increase in heart rate and mean arterial pressure associated with an abrupt increase in anesthetic concentration has not been observed during sevoflurane anesthesia [26]. It is believed that the neurocirculatory excitatory effect of isoflurane is caused by stimulation of the sympathetic nervous system located in the upper airway and in the lung in response to a sudden increase in alveolar concentration of the anesthetic. This effect is more likely to occur for inhalation anesthetics with low blood solubility that is capable of causing a rapid rise in alveolar concentration by increasing the inspired concentration delivered to the patient [27]. However, prior administration of fentanyl, alfentanil, or clonidine has been shown to blunt the neurocirculatory excitatory response to abrupt increase of desflurane concentration [28, 29]. A similar suppression response may occur if these drugs are administered prior to isoflurane anesthesia. In general, isoflurane does not affect cardiac output as much as sevoflurane. Cardiac output decreases significantly at 1 and 1.5 MAC of sevoflurane but returns to near‐awake values at 2 MAC [30]. It is believed that isoflurane may possess mild β agonist effects and the resultant sympathomimetic effect is reflected as an increased heart rate, decreased systemic vascular resistance, and overall unchanged cardiac output in human patients [30]. However, this theory has not been supported by animal data [31]. Neither isoflurane nor sevoflurane overly sensitizes the myocardium to circulating catecholamines, thus they are unlikely to cause cardiac dysrhythmias in susceptible patients [30]. Table 6.1 Blood–gas solubility, metabolism, and MAC of isoflurane, sevoflurane, and desflurane in farm animal species. N/A, not applicable. Hypoventilation and increased PaCO2 are often observed during inhalation anesthesia as a result of medullary respiratory center depression and reduced chest wall expansion due to anesthetic‐induced intercostal muscle relaxation [4]. Furthermore, positioning of ruminant patients in lateral or dorsal recumbency for surgery results in compression of the diaphragm, which collapses the caudal lung lobes by the cranial shifting of the rumen and further debilitates the ventilation function of an anesthetized ruminant patient [32, 33]. Sevoflurane produces a dose‐dependent increase in respiratory rate in human volunteers. Isoflurane increases the respiratory rate up to a concentration of 1 MAC. A further increase in isoflurane concentration is not associated with a further increase in the respiratory rate. Awake patients may be able to compensate for a decrease in respiratory rate by increasing the tidal volume to maintain normal minute ventilation and prevent the increase in PaCO2. However, dose‐dependent depression of the response of the medullary respiratory center to the increased PaCO2 is often observed, which prohibits central compensatory mechanism by increasing respiratory rate or tidal volume in an effort to maintain normal PaCO2 during isoflurane or sevoflurane anesthesia [23, 34]. Isoflurane and sevoflurane both produce bronchodilation in patients suffering from chronic obstructive pulmonary disease. Isoflurane vapor has a special pungent odor and has been shown to cause airway irritation, coughing, and breath holding during induction. On the contrary, sevoflurane has been described as “pleasant smelling,” and does not irritate the airway. Therefore, sevoflurane is often preferred for mask inductions [35]. Isoflurane, sevoflurane, and desflurane have little effect in overall hepatic and renal function in healthy patients. Isoflurane has been reported to decrease portal venous blood flow at 1.5 MAC, but total hepatic blood flow and hepatic arterial blood flow are shown to be within normal range. Therefore, hepatic perfusion is well maintained, which when combined with isoflurane‐induced vasodilation ensures adequate hepatic O2 delivery [36]. Of the three most popular inhalation anesthetics, isoflurane is probably the one that better maintains hepatic O2 supply and is least likely to cause hepatic injury. Nevertheless, sevoflurane and desflurane produce hepatic effects similar to isoflurane [37–39]. The elimination of these inhalation anesthetics depends primarily on ventilation rather than the hepatic metabolism. Sevoflurane has a higher hepatic metabolism (3%) than isoflurane (0.2%) and desflurane (0.02%). Apparently, sevoflurane is 10 times more vulnerable than isoflurane and 100 times more than desflurane to hepatic metabolism and the subsequent production of inorganic and organic fluoride [23]. Fluoride‐induced renal toxicity and renal dysfunction with a fluoride concentration of 50 μmol/l or greater have been previously documented with the administration of one of the older‐generation inhalation anesthetics, methoxyflurane [40]. Clinical studies in humans showed that plasma fluoride concentrations can be maintained below 80 μmol/l, even though peak plasma fluoride concentration is rarely reached due to methoxyflurane’s high blood solubility and slow rise in blood concentration [41]. Further evidence shows that renal dysfunction rarely occurs even with a peak plasma fluoride concentration of greater than 50 μmol/l, but less than 80 μmol/l, in the presence of high sevoflurane concentration and prolonged duration of anesthesia [41–46]. That being said, sevoflurane‐induced cardiovascular depression may enhance the renal toxicity effect by detrimental hepatic or renal effects, for example hepatocellular injury due to reduced hepatic blood flow and decreased O2 delivery and diminished renal function as a result of the decreased renal blood flow and glomerular filtration rate. Therefore, it is important to maintain normal cardiovascular function and ensure adequate hepatic and renal perfusion in anesthetized patients. Compound A is a vinyl ether produced as one of the degradation products of sevoflurane presented in the CO2‐absorbent canister as a trace contaminant. Breakdown of sevoflurane to compound A has been associated with administration of high sevoflurane concentrations, the presence of the dry alkaline CO2 absorbent (e.g. soda lime or baralyme), the use of the low O2 flow in the breathing circuit, and the production of high temperature from the chemical interaction of exhaled CO2 with the CO2 absorbent [47–56]. In rats, inhalation of high compound A concentrations has been shown to cause fatal renal injuries [56]. However, it is believed that compound A is less toxic in humans due to lower β‐lyase enzyme activity compared to rats [57]. When a fresh O2 flow rate of 2 l/min was used in rats during sevoflurane anesthesia, which provided much higher O2 than the minimum metabolic O2 requirement of the rats, a very low concentration of compound A in the breathing circuit of the anesthetic machine was analyzed, and renal toxicity to the rats was not reported [34]. Increased production of compound A has been associated with higher CO2 absorbent temperatures up to 46 °C. Also, studies have shown that CO2 absorbent that contained NaOH and/or KOH often resulted in higher compound A production. No compound A was produced when NaOH‐ and KOH‐free CO2 absorbent was used [55, 58]. In rats, the concentrations of compound A that are associated with renal toxicity and median lethal concentration following 1 hour of sevoflurane anesthesia are reported to be 100–300 ppm [59] and 1050–1090 ppm [60], respectively. In humans, peak compound A concentration of less than 40 ppm was reported even after prolonged duration of sevoflurane anesthesia [49, 61]. Similarly, a peak compound A concentration of 61 ppm was reported in dogs anesthetized with sevoflurane using a low flow (fresh O2 flow rate 3 ml/kg/min), closed circuit system for 1 hour [62]. Kandel et al. [60] reported that renal injury in rats only occurred in the presence of a compound A concentration of at least 200 ppm with a maximum of 1 hour of exposure time. Fortunately, there is no report of compound A‐related renal toxicity under normal clinical conditions in humans or domestic animals. All halogenated inhalation anesthetics are known triggers for malignant hyperthermia. Halothane appears to be the most potent and the most frequently reported in pigs. Isoflurane has been reported to trigger malignant hyperthermia in susceptible pigs like Pietrain or Pietrain‐mixed pigs [63]. Incidences of isoflurane‐induced malignant hyperthermia have been reported in a potbellied pig [64] and a bull [65]. Sevoflurane‐induced malignant hyperthermia has been reported in purebred Poland China pigs [66]. Episodes of malignant hyperthermia induced by desflurane have been reported in Large White, Pietrain, and Pietrain‐mixed pigs [63, 67]. Compared to well‐documented halothane‐induced malignant hyperthermia, the episodes induced by desflurane and isoflurane were reported to have a slower onset than halothane [63]. Please refer to Chapters 1 and 7 for detailed discussion and treatment of malignant hyperthermia. In steers anesthetized for rumenotomy with atropine/guaifenesin/thiamylal for induction and either isoflurane or halothane for maintenance, higher heart rate and lower respiratory rates were observed during isoflurane than those observed during halothane anesthesia. Mean arterial blood pressure was not significantly different between the two anesthetics. Surprisingly, the end‐tidal isoflurane concentration required for rumenotomy surgery (1.7% at 15 minutes and 1.3% at 90 minutes) was lower than that of halothane (2.6% at 15 minutes and 1.7% at 90 minutes) [68]. In most of the other domestic species, isoflurane is reported to be less potent than halothane with 1.5 MAC values being 1.95% (1.5 × 1.3%) and 1.35% (1.5 × 0.9%) vapor concentration for isoflurane and halothane, respectively. The reason for this discrepancy remains unknown [68]. Both anesthetics produced a similar degree of respiratory depression which was reflected in the same level of PaCO2 accumulation during anesthesia. However, it seems that animals anesthetized with isoflurane tend to breath with greater tidal volume to compensate for the lower respiratory rate caused by isoflurane. On the contrary, a decreased tidal volume accompanied by an increased respiratory rate in isoflurane‐anesthetized calves was not able to prevent hypoventilation, as evidenced by a significant increase in PaCO2 throughout the anesthesia period. In normal, awake animals, an increase in PaCO2 (hypoventilation) and a decrease in PaO2 (hypoxia) are the primary stimulants for the medullary respiratory center and peripheral chemoreceptors to increase respiratory rate or tidal volume to maintain normal PaCO2 and PaO2. When the medullary respiratory center and peripheral chemoreceptors are depressed by isoflurane during anesthesia, hypoxia becomes the primary stimulant responsible to improve ventilation. However, 100% O2, typically used as the carrier gas for all the inhalation anesthetics, more specifically isoflurane in this case, eliminated the ventilatory drive that normally responds to hypoxia, resulting in an accumulation and subsequent increase in PaCO2 despite an increased respiratory rate [69, 70]. Nonetheless, calves induced with xylazine (0.1 mg/kg IM) and ketamine (2 mg/kg IV) and maintained with isoflurane in O2 for repair of umbilical hernia had better arterial oxygenation, less degree of pulmonary vascular shunting, and better quality of anesthesia than those induced with xylazine (0.2 mg/kg IM) and ketamine (5 mg/kg IV) and maintained with intermittent IV ketamine (2.5 mg/kg) [71]. In calves, mask induction with desflurane to tracheal intubation only required 151 ± 32.8 seconds. No struggling, breath holding, or coughing was observed during the induction period, indicating lack of upper airway irritation from desflurane. Anesthesia was maintained at mean end‐tidal desflurane concentration of 10 ± 0.76% with control ventilation. No surgery was performed in these calves during desflurane anesthesia. The only cardiovascular changes observed were significant decreases in arterial blood pressures and systemic vascular resistance. Heart rate and cardiac output did not change from baseline values, suggesting that the decrease in systemic vascular resistance was responsible for the decrease in arterial blood pressure observed in these calves, similar to that seen with isoflurane and sevoflurane. Desflurane anesthesia was discontinued after 45 minutes of recording of cardiovascular variables, and isoflurane was then used to maintain anesthesia for surgical placement of a duodenal cannula and thymectomy. All calves in this study recovered uneventfully. Compared to isoflurane and sevoflurane, desflurane, due to its low blood solubility, has the advantage of having a very rapid induction and recovery, and ease of adjustment of anesthetic depth [72]
6
Inhalation Anesthesia and Use of Ventilators
Isoflurane
Sevoflurane
Desflurane
Blood–gas solubility [7]
1.46
0.68
0.42
Hepatic metabolism [7]
0.2%
3–5%
0.02%
MAC values (%)
Cattle
Calves: 1.47 [8]
N/A
N/A
Goats
1.13 ± 0.03 [9]
2.3–2.7 [10]
N/A
1.29 ± 0.11 [5]
2.33 ± 0.15 [5]
1.63 ± 0.17 [11]
Sheep
1.01–1.58 [12, 13]
3.3 [14]
9.5 [15]
1.51 [16]
Llamas/alpacas
1.05 ± 0.17 [17]
Llamas: 2.29 ± 0.14 [18]Alpacas: 2.33 ± 0.09 [18]
Llamas: 7.99 ± 0.58 [19]Alpacas: 7.83 ± 0.51 [19]
Pigs
1.65 ± 0.36–2.04 ± 0.19 [20]
4.1 (3.65–4.5) [21]
8.28 ± 1.34%–10 ± 0.94 [20]
3.5 ± 0.1 [22]
6.1 Cattle
Stay updated, free articles. Join our Telegram channel
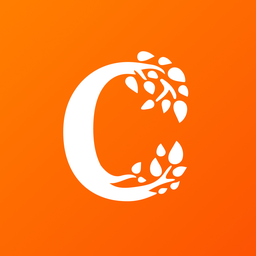
Full access? Get Clinical Tree
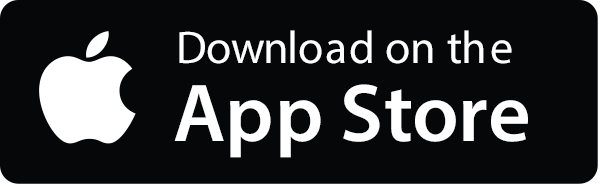
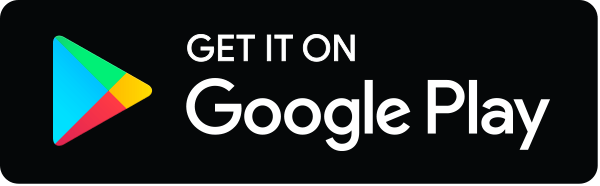