3 José A. Ramos‐Vara1 and Luke B. Borst2 1Purdue University, USA 2North Carolina State University, USA In many laboratories, immunohistochemistry (IHC) has become a routine supplement to the classic morphologic approach of investigational pathology.1 Though its main application in veterinary oncology is in the characterization and diagnosis of neoplastic diseases, current trends highlight a central role for IHC in prognosis and theranostics.2–4 IHC bridges three disciplines: immunology, histology, and chemistry. Antigens are recognized in histologic sections by specific antibodies. The antigen–antibody binding is then visualized by light or fluorescent microscopy as a colored histochemical reaction.5 The value of IHC in the study of neoplastic diseases has been enhanced by increased sensitivity and specificity, improved detection of multiple antigens in the same histologic section, simultaneous evaluation of numerous samples with tissue microarrays, new antigen retrieval (AR) methods, and sophisticated automation.6 The authors believe IHC is a powerful tool in oncology, but only in concert with morphology. A blue, red, or brown reaction with IHC is of little or no help if not accompanied by an understanding of the morphologic changes typical of a specific neoplasm. Therefore, IHC is best utilized as a complement to morphology and should be considered as a piece of data in the diagnostic puzzle. IHC used in isolation or as the test to overrule all others may lead to spurious results. As Basturk et al. stated: “There is no IHC marker that makes the diagnosis by itself. Exceptions always occur, and unfortunately, they tend to merge when IHC is necessary the most”.7 For a review of immunocytochemistry applied to neoplastic diseases, please see reference 8. The IHC technique is a combination of immunologic and chemical reactions visualized with a photonic microscope. The technique can be divided into three main phases (Table 3.1). Phase 1 (preanalytical) starts with sample procurement, followed by tissue fixation, trimming, embedding, and tissue sectioning on a microtome. Phase 2 (analytical) starts with deparaffination of tissue sections and includes all the steps from AR and blocking nonspecific activities through the binding and dection of primary antibody and ending with counterstaining and coverslipping. Phase 3 (postanalytical) includes evaluation of the IHC control, interpretation of results and generation of an IHC report.1 Table 3.1 Steps in an immunohistochemical test Fixation of tissues is necessary to (1) preserve cellular components (e.g., including soluble and structural proteins); (2) prevent autolysis and displacement of cell constituents (e.g., antigens and enzymes); (3) stabilize cellular materials against deleterious effects of subsequent procedures; and (4) facilitate conventional staining and immunostaining.2 However, there is no “one fixative fits all” in IHC; in fact, some antigens can only be detected in fresh frozen sections.3 In general, two types of chemical fixatives are used in histopathology: cross‐linking (noncoagulating) fixatives and coagulating fixatives.4 Formaldehyde is a cross‐linking fixative and is the standard and most common fixative for both routine histology and IHC. Formaldehyde preserves mainly peptides and the general structure of cellular organelles. It also interacts with nucleic acids, but has minimal preservation of carbohydrates.5,6 It can be a good preservative of lipids if the fixative is supplemented with calcium.7 Formaldehyde is used mainly because it is reliable for general histology, it is inexpensive, and its deleterious effects can often be countered with AR. In addition, over the decades pathologists have become accustomed to the artifacts produced by this fixative.8 In contrast, formalin‐substitute fixatives are often based on alcohol solutions; therefore, their mechanism of fixation is dehydration and protein coagulation.9–12 A recent study indicates that Weigners fixative (a mixture of alcohols and pickling salt) performs comparably to formaldehyde for IHC and even maintained immunoreactivity for some biomarkers after prolonged fixation, whereas formaldehyde did not.13 If a formalin substitute is used for an IHC test already validated on formalin‐fixed tissues, a new validation of the test is mandatory.14 Fixation with formaldehyde is a time‐dependent, three‐step process that entails penetration, covalent bonding (binding), and formation of cross‐links.10,15 These steps happen simultaneously, but at very different rates, with penetration occurring around 12 times faster than binding, and binding 4 times faster than cross‐linking.10 As an example, a 3‐mm‐thick sample will be 100% penetrated, 24% bonded and 6% cross‐linked in 8 hours; after 24 hours of fixation it will be 70% bonded and 36% cross‐linked.16,17 Fixation is also temperature dependent; fixation at 37 °C occurs faster than at 25 °C.18 Although there is no optimal standard fixation time for every antigen, it is recommended that diagnostic specimens be fixed for 16–32 hours, with complete fixation, on average, achieved after 24–48 hours.14 Samples to be fixed should not be thicker than 2–4 mm. The basic mechanism of fixation with formaldehyde is the formation of addition products (adducts) between the formalin and uncharged reactive amino groups (‐NH or ‐NH2), which eventually will form cross‐links.18 Once the addition product (reactive hydroxymethyl compound) is formed, additional cross‐linking will happen. In the presence of a second reactive hydrogen, the hydroxymethyl group will form a methylene bridge. Therefore, the final result of formaldehyde fixation is a profound change in the conformation of macromolecules, which may make the recognition of antigens by antibodies impossible or, at best, difficult (Figure 3.1).19 For example, these changes modify the three‐dimensional (tertiary and quaternary) structure of proteins, whereas the primary and secondary structures are little affected.2,20 Changes in the tertiary structure of proteins may also occur in subsequent interactions of cross‐linked proteins with ethanol or clearing agents during tissue processing.15,21,22 Figure 3.1 Effects of formalin fixation on proteins. Formalin fixation produces conformational changes in proteins secondary to cross‐links between protein groups and the fixative. The use of antigen retrieval (heat‐induced epitope retrieval, HIER) is intended to revert those changes. (Source: Ramos‐Vara and Miller, 2014. Reproduced with permission of SAGE Publications.) The fixation step is a critical quality control point, so standardized laboratory practices are needed to ensure accurate and repeatable IHC using formalin‐fixed paraffin‐embedded (FFPE) tissues.14 Prolonged fixation thoretically can produce false‐negative results due to excessive cross‐links;2 however, the use of heat‐based AR methods considerably mitigates the impact of overfixation in many cases (Figure 3.1).23–30 That said, the effect of prolonged fixation with formaldehyde on an antigen located in different cell compartments may vary (Figure 3.2).2 Figure 3.2 Effects of fixation on antigen expression. Antigens are affected by fixation differently. (A,B) Horse, fetus lung infected with Bartonella sp. (A) Fixation for 2 days: antigen (arrowheads) is easily detected. (B) Fixation for 11 weeks: Antigen detection is lost. (C,D) Dog, skin. Cytokeratins. (C) Fixation for 2 days: Strong detection in adnexal structures. (D) Fixation for 7 weeks: Significant loss of immunoreactivity. Immunoperoxidase‐DAB. (Source: Ramos‐Vara and Miller, 2014. Reproduced with permission of SAGE Publications.) Underfixation is currently considered a more common and serious problem than overfixation.14,31 If large samples are underfixed, cross‐links develop only in the periphery of the specimen with the core of the tissue left unfixed or fixed through coagulation by the series of alcohol gradients prior to paraffin embedding.2,32 This can result in a staining gradient in IHC that can confound interpretation (Figure 3.3).14 In addition, underfixed tissues may undergo inadequate antigen retrieval or produce unexpected antigen detection, which can result in false‐negative or false‐positive results, respectively.33,34 Figure 3.3 Effects of inadequate fixation. Dog, lymph node. Diffuse large B cell lymphoma. This sample was submitted without slicing, resulting in incomplete penetration of fixative and inadequate staining. (A) Reduced staining with hematoxylin in the center of the sample. (B) Lack of staining for CD79a in the center of the sample. Immunoperoxidase‐DAB. (Source: Ramos‐Vara and Miller, 2014. Reproduced with permission of SAGE Publications.) Like underfixation, delayed fixation may affect IHC results, particularly when apoptotic markers or markers recognizing phosphorylated proteins are used.14,35–37 Enzyme‐rich tissues, such as intestine or pancreas, autolyze rapidly; proteolysis may lead to increased background staining.14 Diffusion of soluble proteins may occur with fixation delay and has been reported with thyroglobulin, myoglobin, glial fibrillary acid protein (GFAP), and other cellular proteins.38–40 Decalcification with weak acids does not seem to interfere significantly with IHC for most antigens, provided the tissues are well fixed in formalin.32,41 Strong acid decalcifying solutions have negative effects on immunoreactivity, at least for some antigens.42–45 Therefore, weak acid (e.g., formic acid) decalcifying solutions diluted in formalin are recommended for IHC. Due to their potential negative effects, if decalcifying solutions are required then the IHC report should indicate the type of decalcification. Fixation may not be the sole cause of failure to detect an antigen; in some cases, tissue processing is also a key limiting factor.46–48 Evidence for a cumulative effect of fixation and tissue processing as the major cause of lack of antigen recognition in FFPE tissues has been reported.10,49 Changes in the tertiary structure of proteins during the dehydration and clearing steps of tissue processing can reduce or abolish antibody recognition without AR or can increase background staining.14 These negative effects depend on the dehydrating and clearing agent used.50 Paraffin sections dried overnight at temperatures of 60 °C or higher may have reduced immunoreactivity for some antigens.50–53 However, a combination of temperature and duration of drying may be more influential than temperature alone.54 Paraffin blocks contain archived tissue suitable to evaluate morphologic and immunohistochemical features of diseases and apply the results to targeted medicine.55 It is the opinion of the authors and others that paraffin blocks remain stable for years in terms of antigenicity.50 Any deleterious effects of prolonged paraffin block storage on tissue antigenicity could differ among antigens and should be considered if IHC results on old paraffin blocks are inconsistent or unexpected. Storage of unstained paraffin control tissue sections increases efficiency but may adversely affect immunoreactivity (tissue section aging/slide oxidation/biomolecule degradation).14,50,56 These differences are epitope specific rather than protein target specific.14 Both light (photo‐oxidation) and temperature contribute to this process.4 Tissue section aging is a common problem with nuclear antigens (e.g., Ki67, estrogen receptor, p53); however, in a recent study with markers employed in animal tissues, cytoplasmic membrane antigens were more sensitive than nuclear antigens to degradation from photo‐oxidation and/or ambient temperature.4,33,57–66 Others have reported a negative effect on immunoreactivity when using tissues suboptimally dehydrated (retained endogenous water) during paraffin embedding or with paraffin sections stored under high humidity conditions.67 To reduce antigen degradation due to oxidation, paraffin sections should be used within a week of sectioning or at least should be stored in an airtight container in the dark and in the cold (refrigerator or freezer).4,14 Because fixation and tissue processing modify the three‐dimensional structure of proteins (antigens), these essential steps can render certain antigens undetectable by specific antibodies. This dilemma is better understood if we remember that, in general, an immunologic reaction between antigen and antibody depends on the conformation of the former.1 Antigen retrieval (AR) methods that reverse the conformational changes in FFPE tissues are particularly important (Figure 3.1). Approximately 85% of antigens fixed in formalin require some type of AR to optimize the immunoreaction.2 The two most common AR procedures in IHC are enzymatic and heat‐based retrieval. Protease‐induced epitope retrieval (PIER) was the most commonly used AR method before the advent of heat‐based AR in the 1990s. Many enzymes (e.g., trypsin, proteinase, K., pronase, ficin, and pepsin) have been used for AR.3–7 The mechanism of PIER is probably protein digestion, but the cleavage is nonspecific and some antigens may be negatively affected.8 It is our preference to optimize a few enzymes rather than to use a broad range of enzymes. The disadvantages of PIER are that it is the optimal AR method for only a few antigens, that it can alter tissue morphology, and that it can damage epitopes.6,8 HIER was introduced by Shi et al. and is based on a concept developed by Fraenklen‐Conrat et al., who documented that the chemical reactions between proteins and formalin may be reversed, at least in part, by high‐temperature heating or strong alkaline hydrolysis.9–12 HIER involves heating FFPE tissue sections in an AR buffer. The mechanism involved in HIER is not completely understood, but its final effect is reversal of changes produced during fixation. The mechanism requires the dissociation of irrelevant proteins from target peptides.13 The secondary and tertiary structure of proteins is probably modified (denatured) during HIER; however, this does not affect the immunoreactivity of most antigens because IHC antibodies require only an intact primary (linear) protein structure.14,15 Tissue‐bound calcium ions may be important in masking some antigens during fixation, explaining why calcium chelating substances (e.g., EDTA) are sometimes more effective than citrate buffer in antigen retrieval.16–19 Restoring native electrostatic charges modified during formalin fixation has also been considered as an AR mechanism.20,21 Various types of equipment may be used for HIER, including a de‐cloaker (commercial pressure cooker with electronic controls for temperature and time), vegetable steamer, water bath, microwave oven, or pressure cooker.9,18,22–24 A universal AR solution is not available. AR solutions which have been used in HIER vary in the composition of the buffer (e.g., citrate, tris, Tris‐HCl) and pH range (3–10).1,25 Successful AR retrieval using HIER requires the optimum combination of incubation temperature and AR solution pH and chemical composition. For example, some antigens can be retrieved with low pH solutions, others only with high pH solutions, and a third group with solutions with a wide pH range.26,27 In our and others’ experience, HIER with 0.01 M sodium citrate buffer (pH 6.0) frequently results in successful AR, while preserving cell morphology better than higher pH buffers or solutions containing EDTA.1,28,29 However, the possibility of unexpected immunoreactivity (false‐positive labeling) should always be considered when using HIER, particularly with low pH buffers.28 Recently, so‐called three‐in‐one retrieval buffers have been introduced for use in IHC. These buffers can perform aqueous deparaffination, rehydration, and AR.30 However, their use in IHC is relatively new, and comparison with traditional means of deparaffination, rehydration, and AR is required before routine use in a diagnostic setting as issues related to incomplete removal of paraffin by these buffers have been reported.31 The use of mechanical vibrations by ultrasound as an AR method has been successful in restoring tissue immunoreactivity for some antigens.32–34 This method presumably breaks cross‐links produced during fixation. Fundamental to the IHC test is the ability of an antibody to specifically recognize and strongly bind to an epitope on the desired target antigen.1 From a biochemical point of view, these antigen–antibody (Ag–Ab) interactions are the result of noncovalent bonds and are caused primarily by hydrophobic, electrostatic, and van der Waals forces.2 The specificity of the IHC test is largely dependent on the the ability of the primary antibody to bind to epitopes on the target antigen without cross‐reacting to epitopes on off‐target antigens. Regions of an antigen that are recognized and bound by antibodies are called epitopes and generally consist of 5 or 6 amino acid residues.1 Multivalent antigens have multiple epitopes which can be either identical (homopolymeric) or unique (heteropolymeric).3 In addition, a specific antigen can have different structures (isoforms) which can contain unique epitopes.3 For example, most protein antigens are multivalent and heteropolymeric which means that for a specific protein antigen, several different antibodies can be raised that bind unique epitopes with variable strength (affinity). Furthermore, these antibodies may or may not recognize an isoform of the same protein. Two broad groups of immunogens are used to produce antibodies: synthetic peptides and purified proteins.4 Synthetic peptides have the advantage of a known amino acid sequence. However, synthetic peptides may lack the normal three‐dimensional structure of the native protein, and other proteins can be intimately associated with the protein of interest in vivo. Both these factors can mask target epitopes, preventing their detection by antibodies raised to synthetic peptides. Also in vivo post‐translational modifications of the native antigen are not present in synthetic peptides.5,6 These factors theoretically decrease the sensitivity of primary antibodies raised to synthetic peptides for target antigens. Furthermore, using synthetic peptides as immunogens can result in decreased antibody specificity. For example, the sequence of the synthetic peptide could also be present in unrelated antigens, producing immunologically specific antigen–antibody binding (molecular mimicry) despite the absence of the target antigen.7–9 Use of purified proteins as immunogens avoids many of the problems of synthetic peptides.4 However, purification of a protein to homogeneity from either cells or tissues can be technically difficult. Also, contaminating proteins may be more antigenic than the protein of interest, producing a disproportionate and unwanted immunogenic response. Another problem with purified proteins arises if the targeted antigen includes highly immunogenic epitopes that are not specific to the antigen of interest. For the IHC test, the most commonly used immunoglobulin (Ig) is IgG; IgM is used less commonly.9 Immunoglobulins are “Y” shaped and consist of two identical light chains and two identical heavy chains (Figure 3.4). The heavy chains determine the antibody class. The tail of the Y is called Fc (fragment, crystallizable) and is composed of two heavy chains on the C‐terminal side.10 Each end of the forked portion of the Y is called Fab (fragment, antigen‐binding) region. The light chains of most vertebrate antibodies have two distinct forms called kappa and lambda. In any immunoglobulin molecule, both light chains and both heavy chains are of the same type. The light chains consist of two distinct regions: the C‐terminal half of the chain is constant and called CL (constant: light chain), whereas the N‐terminal half of the chain has abundant sequence variability and is called the VL (variable: light chain) region. The Fab (antigen‐binding) region of the immunoglobulin has variable and constant segments of the heavy and light chains. The Fc portion determines the biological functions and permits antibody binding to other antibodies, complement, and immune cells with Fc receptors.9 The specific binding of an antibody to an antigen occurs via hypervariable regions of both heavy and light chains of the amino terminus.9 The antigen‐binding site of an antibody is called the paratope.11 Figure 3.4 Structure of an immunoglobulin molecule. In immunohistochemistry, both the variable (antigen‐binding site) and the Fc (antibody‐binding site) regions are necessary for proper detection of the antigen–antibody reaction. (Source: Ramos‐Vara and Miller, 2014. Reproduced with permission of SAGE Publications.) Paratopes recognize either linear or conformational epitopes.12 Linear epitopes are a group of 5–7 (and up to 21) contiguous amino acids. Conformational (discontinuous) epitopes, the typical form, consist of small groups of amino acids brought together by conformational folding or binding.13 Although most epitopes involved in immune responses are believed to be conformational, there is evidence that antibodies used in FFPE tissues mainly, or exclusively, recognize linear epitopes.12,13 Affinity is a thermodynamic expression of the binding strength of an antibody (paratope) to an antigenic determinant (epitope).3 Affinity can be defined in mathematical terms as an affinity constant (Ka), which represents the amount of antibody–antigen complex that will be formed at equilibrium. The affinity of the antigen–antibody reaction has practical importance in that high‐affinity antibodies can (1) bind more antigen in a shorter incubation time than low‐affinity antibodies; and (2) in general, be used at lower concentrations than low‐affinity antibodies.14 Avidity (also called functional affinity) is a measure of strength of binding between bi‐ or multivalent antigens and antibodies (combined bond affinities).1 Avidity is the result of the affinity(ies) of the antibody for the epitope(s), the number of antibody‐binding sites, and the geometry of the antigen–antibody complexes.3 As a result, an antibody of IgM (decavalent) has a higher avidity (although typically lower affinity) than an antibody of IgG (bivalent).1,3 Polyclonal antibodies (PAbs) are produced with purified antigen in various species (mouse, rabbit, goat, donkey, horse, hamster, sheep, guinea pig, rat, chicken); however, the rabbit is the most common species. PAbs have higher affinity and wide reactivity, but lower specificity in comparison to monoclonal antibodies (mAbs).11 Polyclonal antisera include not only several different antibodies to multiple valencies on the target protein, but also irrelevant antibodies that can be present in high concentration (up to 10 mg/mL) if not affinity‐purified.4,15 PAbs have the advantage over mAbs in that they often identify multiple epitopes and isoforms of the target protein. However, variations in antibody titer and quality, depending on the animal immunized, also contribute to variance among batches of antibodies.16 The “immunologic promiscuity” of PAbs, which can be an advantage (e.g., more possibilities of detecting an antigen due to multiplicity of epitope recognition) can also be a disadvantage: The greater the number of different antibodies to the target protein in the preparation, the greater the likelihood of cross‐reactivity with similar epitopes in other proteins and, therefore, the greater the likelihood of false‐positives.4 Monoclonal antibodies (MAbs) are produced mostly in mice and react with only one valency site per antigen.17 Background reactivity with MAbs due to nonspecific Igs is reduced (ascites fluid) or non‐existent (cell culture supernatant). The high specificity reduces, but does not eliminate, the possibility of cross‐reactivity with other antigens because MAbs target epitopes consisting of a small number of amino acids, which can be contained in multiple proteins and peptides.18 MAbs can also be produced in rabbits. The advantages of rabbit over mouse MAbs are their higher affinity (probably a result of high glycosylation), suitability for use on mouse tissues without special procedures, increased specificity in some cases, and less need for antigen retrieval.1,19–23 In a study comparing 10 rabbit MAbs with 10 mouse MAbs targeting the same protein in animal tissues, rabbit MAbs were superior for some markers; however, there was no significant overall improvement in immunoreactivity.24 A reliable IHC test must be both specific and sensitive; however, there is often confusion surrounding the application of these terms. The confusion stems from the fact that these terms can be applied both to the primary antibody itself as well as to the IHC test as a whole. Further blurring the distinction between antibody and test is the fact that the specificity of the IHC test in large part derives from the primary antibody’s ability to recognize a specific antigen. Likewise, the sensitivity of the IHC test derives from a combination of the binding properties of the primary antibody and the subsequent detection methods. Given the possibilities for confusion, it may be useful to introduce more specific terminology to distinguish the sensitivity and specificity characteristics of the primary antibody from the IHC test as a whole. For example, molecular specificity is the ability of a primary antibody to bind strongly with a specific epitope of the target antigen; this depends on the antibody molecular structure.1 In oncology, ideal molecular specificity would be an antibody that recognizes one antigen only in tumors of one cell type. There are few if any such antibodies. While many primary antibodies specifically recognize their target antigen, these antigens frequently can be expressed by multiple cell types or are expressed by normal cells of a certain type. All markers also have the potential to be expressed by a tumor line that is not expected to have that antigen. As an example, MUM1 is used to recognize plasma cell tumors (B cell differentiation) because it is required for immunoglobulin light‐chain rearrangement at the pre‐B stage of lymphocyte maturation. MUM1 is expressed in the majority of (but not all) cutaneous plasmacytomas and multiple myelomas. In humans, the molecular specificity of MUM1 is diminished because it is expressed in melanoma and T cell lymphomas, including adult T cell leukemia lymphoma and anaplastic large cell lymphoma. However, in dogs MUM1 has excellent molecular specificity as it is not expressed in common differentials for plasma cell tumors, including melanoma, mast cell tumors, histiocytomas, cutaneous and systemic histiocytoses, histiocytic sarcomas, epitheliotroic lymphomas, and T cell lymphomas.25 Furthermore, target antigens expressed by normal cells of a certain type may be variably expressed (downregulated or upregulated) in neoplastic populations. Therefore it is clear that these variations in molecular specificity impact the diagnostic specificity and sensitivity of the IHC test. Likewise, the analytical sensitivity of the IHC test depends, in part, on the primary antibody and is defined as the ability of the test to detect low amounts of antigen over background. Analytical sensitivity is a function of the binding characterists of the primary antibody and the detection methods. Polyclonal antibodies may have more analytical sensitivity than mAbs because of polyvalent binding.23 Polymeric labeling and catalyzed signal amplification detection methods can increase analytical sensitivity by amplifying labeling.1 Detection of low amounts of target antigen (analytical sensitity) is critical to the overall sensitivity and specificity of the IHC. Probably of greatest interest to the diagnostic pathologist is the diagnostic specificity and sensitivity of an IHC test. These are calculated during test standardization and validation (see below) by comparing IHC detection of target antigen in a reference set of “known” normal tissue and tumor types, which are usually determined by morphology.26 However, the approach of using morphology as the gold standard when calculating diagnostic sensitivity and specificity can only provide an approximation of the true diagnostic sensitivity and specificity. Inevitably, tumors of a certain type may exhibit unexpected labeling or indiscernable morphology. As mentioned, the latter case is when IHC would be the most useful; however, these types of tumors are not frequently included during test validation for obvious reasons. As the molecular pathogenesis of specific neoplasms is elucidated, IHC tests might be validated using a combination of morphology and genetic markers that would allow inclusion of these problematic cases. One approach to increase the diagnostic specificity and sensitivity of the IHC test is the application of mixtures of primary antibodies as a “cocktail.” These cocktails exploit the molecular specificity and analytical sensitivity of multiple antibodies to target a specific molecule or cell type. A well‐known example is pancytokeratin. This antibody mixture contains multiple antibodies with specificity to one or more of the 20 keratins that occur in different epithelia (see discussion of Cancer of unknown primary site below). This extremely useful antibody cocktail sensitively detects the keratins in all epithelia via the molecular specificity of the component antibodies. Alternatively, antibody cocktails have been developed to accurately label specific tumor types. A recent example is the development of a cocktail of antibodies for the diagnosis of amelanotic melanoma in dogs.27 In this approach, multiple antibodies that target different cellular components of melanoma cells are combined to increase the diagnostic sensitivity and specificity of the IHC test. This type of cocktail has unique technical challenges. For example, the specific antibodies in the cocktail target unique epitopes in different proteins that may have quite disparate optimal AR conditions and buffers. Combining such antibodies into one cocktail using a single AR method may result in unexpected labeling (false‐positives and false‐negatives). Thus, careful validation of this type of cocktail is important. If the diagnostic sensitivity and specificity of individual antibodies are to be compared to that of the cocktail, the same AR methods and buffers must be used for both the individual antibodies and the cocktail. Additionally, careful documentation of methods is paramount to ensure repeatability among laboratories. Kalyuzhny has summarized the nature of a good antibody: “Having a good antibody for IHC is not only about getting a strong staining signal with low background, but also about knowing the staining makes sense in terms of its histological and physiological relevance.”28 The antigen–antibody reaction cannot be seen with the light microscope unless it is tagged to a visible label. Therefore, labels (reporter molecules) are attached to the primary, secondary, or tertiary antibodies of a detection system to allow visualization of the immune reaction. The most commonly used labels are enzymes (e.g., peroxidase, alkaline phosphatase, glucose oxidase).1 Enzymes in the presence of a specific substrate and a chromogen produce a colored precipitate at the site of the antigen–antibody reaction.2 Most common chromogens impart a brown, red, or blue color to the reaction. The choice of enzyme and chromogen depends on several factors, such as the reaction intensity, antigen location, presence or absence of endogenous pigments, or mounting media used, but often is a matter of personal preference.3 For horseradish peroxidase, 3,3′ diaminobenzidine tetrachloride (DAB) is the most commonly used chromogen, imparting a brown color that is insoluble in organic solvents.2 For alkaline phosphatase, 5‐bromo‐4‐chloro‐3‐indolylphosphate/nitro blue tetrazoliumchloride (BCIP/NBT) (blue, permanent media), Fast red (red, aqueous mounting media), and new fuchsin (fuchsia, permanent media) are the chromogens most commonly used.2 Van der Loos has reviewed the chromogens used in multiplex IHC with photonic microscope and spectral analysis.4 The primary goal of detection in IHC is to use the fewest steps in the shortest time for visualization of the optimal immunoreactivity in tissue sections.5 In other words, the sensitivity of the detection system affects the outcome of an IHC test. In addition, the detection system must be reproducible, accurate, and render an excellent signal‐to‐noise ratio when combined with the AR method used.6 The detection system must be compatible with the animal species to be tested. Some companies marketing IHC detection systems for veterinary use optimize their detection kits based on the species evaluated (e.g., PromARKTM for dogs and cats, food animals, etc.), reducing the chances of background or false‐positive staining. The three more commonly used IHC methods are avidin–biotin based methods, non‐avidin–biotin polymer methods and catalyzed signal amplification (CSA). In these methods, the first layer of antibodies is unlabeled, but the second layer (for two‐step methods), raised against the primary antibody, is labeled. For three‐step methods, the tertiary reagent is labeled.7 Avidin is a large glycoprotein extracted from egg whites that has four binding sites per molecule and high affinity for a low molecular weight vitamin called biotin. Streptavidin (from Streptomyces avidinii) produces less background than avidin. Biotin has one binding site for avidin and can be attached through other sites to an antibody (biotinylated antibody) or any other macromolecule such as an enzyme, fluorochromes or other label.7 The increased sensitivity of avidin–biotin methods results from the larger number of biotin molecules (and therefore label molecules) that can be attached to a primary antibody.8–10 One of the most common avidin–biotin methods is the avidin–biotin complex (ABC) method (Figure 3.5A). In this case, the second antibody is biotinylated and the third reagent is a complex of avidin mixed with biotin linked with appropriate label. The avidin and labeled biotin are allowed to react for about 30 minutes before application, resulting in the formation of a large complex with numerous molecules of label (e.g., enzyme). Another commonly used avidin–biotin method is the labeled avidin–biotin (LAB) or labeled streptavidin–biotin (LSAB). This method uses a biotinylated secondary antibody and a third reagent of peroxidase (or alkaline phosphatase)‐labeled avidin. The sensitivity of this method is higher than that of standard ABC.11 Figure 3.5 (A) Avidin–biotin complex (ABC) method. The primary antibody (in black) binds the antigen on the tissue section followed by incubation with biotinylated antibody (in red). Avidin–peroxidase molecules then will bind biotinylated immunoglobulins. The antigen–antibody reaction is detected by a colored reaction produced when the enzyme molecules (e.g., peroxidase) interact with a substrate and a chromogen. (B) Polymer method. This detection system does not rely on the binding of avidin to biotin. The second antibody (in red) is attached to a polymer containing numerous molecules of enzyme. (Source: Ramos‐Vara and Miller, 2014. Reproduced with permission of SAGE Publications.) Endogenous biotin activity or tissue affinity for avidin, known as endogenous avidin–biotin activity (EABA), is common in certain tissues, particularly those rich in biotin, such as the liver, brown fat, adrenal cortex, and kidney. EABA is more prominent after HIER, although it is also present in tissues subject to other types of AR.7,12–15 EABA‐blocking reagents (pure avidin and biotin solutions) are commercially available but less expensive, “homemade” reagents are also effective (egg white solution as a source of avidin; 5% dried milk solution as a source of biotin).14,16–20 This is currently the most widely used non‐avidin–biotin method and the detection method of choice in many laboratories (Figure 3.5B). It consists of a compact polymer to which multiple (4–70) molecules of enzyme (peroxidase or alkaline phosphatase) and the secondary antibody specific for the primary antibody (1–10 Ig molecules) are attached.5,21 The advantages are: (1) simplicity compared to the three‐step methods; (2) equal or higher sensitivity than ABC or LSAB methods; (3) lack of background due to endogenous biotin or avidin.22–26 This method is usually more expensive than ABC or LSAB methods. Higher sensitivity can be achieved using a three‐step method, which uses a bridge antibody to link the primary antibody and the polymer complex. This method is based on the ability of tyramide to bind to a solid substrate (e.g., tissue section) following oxidation/radicalization27 and the deposition of biotinylated or FITC‐conjugated tyramide at the location of the antigen–antibody reaction, catalyzed by horseradish peroxidase (HRP). Highly reactive intermediates formed during the HRP–tyramide reaction bind covalently to electron‐rich amino acids (e.g., tyrosine) of proteins in the vicinity of the HRP‐binding sites, via the production of free radicals by the oxygen liberated by HRP.28 This reaction is very short‐lived and, therefore, the deposition of biotinylated tyramide occurs only at or near the site where it is generated.29 The biotin conjugated to the bound tyramide is subsequently used for the attachment of avidin conjugated to HRP.29 This method is complex and laborious because it involves an initial avidin–biotin procedure followed by the tyramide reaction. However, the sensitivity can be increased 5‐ to 10‐fold compared to the regular avidin–biotin method.30 This method is suitable for antigens present in very low amounts, but background can be a problem, particularly when using HIER.31 Modifications using fluoresceinated tyramide result in marked reduction or complete disappearance of background by endogenous biotin.31–34 With standard IHC on mouse tissues, background labeling develops due to the binding of the secondary antibody (anti‐mouse immunoglobulins) to endogenous immunoglobulins in the interstitium, plasma, B lymphocytes, and plasma cells.28,35 Commercially available mouse‐specific detection systems have eliminated this problem. Secondary antibodies to mouse IgGs may also react with Igs of other species producing the same type of nonspecific background in plasma or any tissue containing IgGs and plasma cells. Proper handling and storage of reagents is essential for reproducibility of IHC tests. The shelf‐life of many reagents is directly linked to appropriate storage, and for many primary antibodies can be extended beyond the manufacturer’s expiration date with proper handling and storage.1–3 However, such use of a reagent must be properly documented. In general, antibodies should be stored at 0–4 °C, in the dark and in vials that do not promote immunoglobulin aggregation. For long‐term storage, freeze antibodies in aliquots at or below −20 °C in a non‐defrosting freezer. A positive tissue control is defined as tissue known to contain the target antigen. Positive tissue controls assess the performance of the primary antibody and ideally must be fixed, processed and stained in the same way as the diagnostic case tissue for every antibody and procedure.1–3 However, time in storage and other practical factors cannot always be controlled in a diagnostic setting. Control tissues should be from the same species as the test tissue and, when possible, should be included on the same slide as the test tissue. The amount of antigen in the positive control should not be overly abundant and should have some heterogeneity in intensity through the sample; weakly immunoreactive areas can be used to detect subtle changes in antibody sensitivity.3 The presence of the antigen in the tissue control should be confirmed by another method or proven in previous publications. Internal positive tissue controls can be present in many test tissues. In the past, the detection of smooth muscle markers or vimentin in normal blood vessels suggested that fixation did not have deleterious effects on immunoreactivity for a given antigen.4,5 However, with the current antigen retrieval procedures, the statement of an “universal” marker to detect fixation problems does not hold true. We and others have demonstrated that fixation effects vary among different markers under the same fixation conditions.6 A much better assessment of the effects of fixation for a given antigen is the detection of the antigen in the test tissue (e.g., detection of CD3 in normal lymphocytes within a node with lymphoma). A negative tissue control is tissue that is known not to contain the antigen of interest.1,3 The use of a negative control is recommended with each IHC run to verify the specificity of the primary antibody and the presence/absence of background (false‐positive) reactivity; if false‐positive reactivity (tissue labeling with a pattern similar or identical to that in the positive control) occurs in the negative control, the test should be considered invalid.1 Negative reagent controls replace the primary antibody. They are used to confirm the test specificity and to assess the degree of nonspecific background caused by the secondary (and sometimes tertiary) antibody.1 Negative reagent controls include: (1) antibody diluent; (2) same species non‐immune immunoglobulin of the same dilution and concentration; (3) an irrelevant antibody; or (4) buffer.2,3 Various methods for the simultaneous study of multiple FFPE tissues in a single histologic section have been reported. The “sausage technique” has been used heavily in the past.7 In this method, long thin strips of fixed tissues are drawn into a tube of unfixed small intestine. The entire sample is then fixed, resulting in a tight column that can be cut into blocks and embedded. Placenta as a “wrapping” tissue also has been used for this method.8 Modifications to the original method have been reported.5 Paraffin‐embedded tissue microarrays have been used as controls in human IHC laboratories, mainly for tumor diagnosis.8–12 The tissue microarray technology allows simultaneous examination of tens to hundreds of tissues on a single microscope slide. A small portion (core) of a tissue sample is taken from the “donor” paraffin block and transferred to the “recipient” block, which may contain up to 1000 cores.13 Validation studies should be carried out on multi‐tissue control blocks containing both known‐positive and known‐negative normal and neoplastic tissues.3 In veterinary medicine, species‐specific tissue microarrays are not commercially available. Species‐specific tumor cell lines are being used for IHC controls (e.g., IHC for HER‐2/neu).2,14 This approach provides an identical tissue control among different laboratories and is an excellent way to compare results.15 The advantages of the tissue microarray method include less reagent consumption, decreased technical time, decreased variability of results, the possibility of digitizing and quantifying results or interpretation of results by hierarchical cluster analysis, quality control, and standardization, evaluation of antibody sensitivity and specificity, and rapid and high‐throughput discovery and validation of biomarkers.16–20 With an adequate selection of control tissues, fewer than 12 tissue cores in an array are sufficient to evaluate more than 90% of the markers used in diagnostic IHC.20 Tissue microarray has some disadvantages when compared with the classic single‐sample microscope slide: it is technically demanding to prepare the array (with a commercial manual or automated array), and careful planning and quality control are required to ensure that cores contain representative tissue. The development of a new IHC test begins with the optimization of each of the steps in the analytical phase (phase 2) of the IHC test including: antigen retrieval, blocking nonspecific activities, and the binding and selection of primary antibody. Critical to the analytical phase is the ability of the primary antibody to specific target antigen in the host tissues for which the test is being developed. Primary antibodies are frequently raised from human or mouse cellular targets and use in veterinary species requires caution and additional testing. Interspecies variations in antibody reactions result from subtle changes in the amino acid sequence of a given antigen and identical antibody clones that target the same antigen can differ in reactivity among species.1 An example is Melan‐A, which labels melanocytes in dogs, cats and other species, but not in horses (Figure 3.6).1 As such, additional validation of an antibody is necessary to confirm that the primary antibody is specific, selective, and reproducibly detects its target antigen.2
Immunohistochemistry: Fundamentals and Applications in Oncology
Introduction
References
The immunohistochemical test
Steps
Variables
Preanalytical phase
Sample procurement
Delayed fixation, prolonged ischemia, thickness of sample
Fixation
Cross‐linking vs. coagulating fixatives, duration
Decalcification
Type of decalcification solution and duration
Tissue processing
Paraffin‐embedded vs. frozen tissues
Tissue sectioning
Thickness of tissue section; drying temperature and duration; tissue section aging
Analytical phase
Deparaffination
Dewaxing agent
Antigen retrieval
Detergents, enzymes, HIER
Blocking nonspecific reactivities
Endogenous enzymes, hydrophobic binding, pigments
Primary antibody
Monoclonal vs. polyclonal; Ag recognition (native vs. linear); specificity; species variability
Detection system
Avidin–biotin vs. polymer‐based systems; ultrasensitive methods
Enzyme–substrate–chromogen
Color detection
Multiplex IHC
Enzyme–substrate combinations
Counterstain
Contrast between chromogen and counterstain
Postanalytical phase
Control performance
Animal species compatibility, tissue processing
Interpretation
Pathologist vs. automated evaluation
Report
Percentage of positive cells; positive vs. negative threshold; standalone test vs.ancillary test
Diagnostic, prognostic or theranostic test
Fixation and processing
Fixation
Decalcification
Tissue processing and incubation buffers
Paraffin section drying and storage of paraffin blocks
References
Antigen retrieval
Enzymatic retrieval
Heat‐induced epitope retrieval (HIER)
References
Antigens and antibodies
Antigens
Antibodies
Affinity
Avidity
Polyclonal antibodies
Monoclonal antibodies
What makes an antibody good for immunohistochemistry?
References
Detection methods
Avidin–biotin methods
Polymeric labeling two‐step method
Catalyzed signal amplification
Immunohistochemistry on mouse tissues
References
Storage and handling of reagents
References
Controls in immunohistochemistry
Positive tissue control
Negative tissue control
Reagent (antibody) controls
Methods for high‐throughput evaluationof control tissues
References
Standardization (optimization) and validation of a new IHC test
Primary antibody standardization and validation
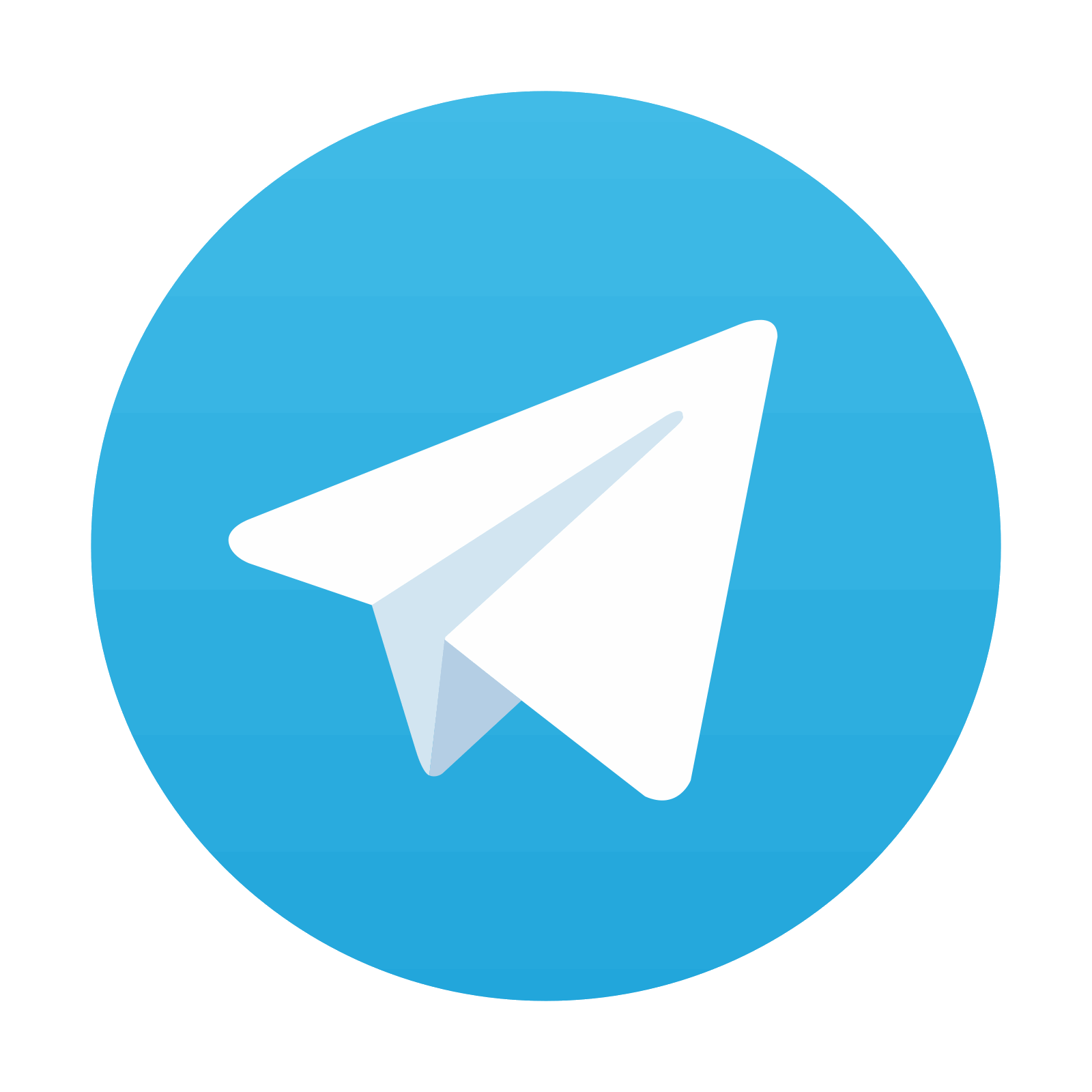
Stay updated, free articles. Join our Telegram channel
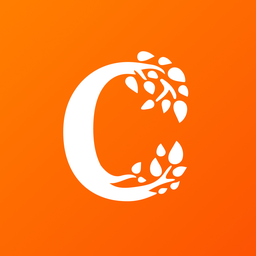
Full access? Get Clinical Tree
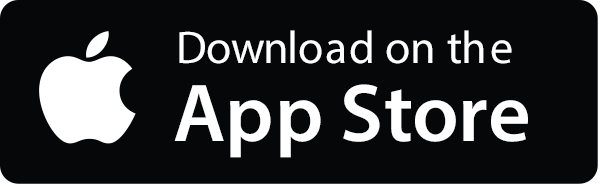
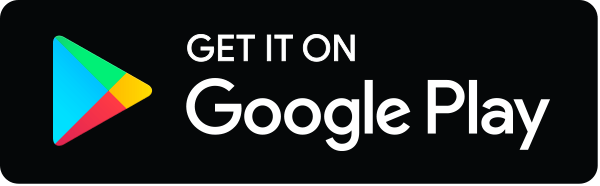