Functional knowledge of ocular physiology in animal species provides a critical foundation for clinicians practicing comparative and veterinary ophthalmology. This chapter presents the physiology of the eye, especially regarding the adnexa, anterior segment, ocular circulation, aqueous humor (AH) dynamics, lens, and vitreous; optics; and vision. The rate at which both relatively simple and complex ocular physiological mechanisms are being studied is incredible. This chapter presents the essential physiological phenomena of the eye, optics, and vision required by the clinical veterinary ophthalmologist. The eyelids of domestic animals protect the eye, particularly the cornea. All domestic animal species have a superior (upper) and an inferior (lower) eyelid; most have a nictitating membrane (NM, third eyelid). The eyelids contain the meibomian glands; these are large sebaceous glands that secrete the outer, oily layer of the precorneal tear film (PTF). The conjunctiva lines the inside of the eyelids and reflects onto the globe contains goblet cells that contribute the mucin to the PTF; accessory lacrimal glands are also present in some species. The normal blinking of the eyelids maintains the physiological thickness of the preocular tear film, aids movement of the tears both to and within the nasolacrimal system, and helps eliminate small particles from the corneal and conjunctival surfaces. Reflex closure of the eyelids protects the anterior segment from external trauma. The eyelids determine the shape and width of the palpebral fissure, along with the associated medial and lateral canthal ligamentous and muscle attachments. For example, a wide, round palpebral fissure is normal among brachycephalic breeds, and a narrow, almond‐shaped palpebral fissure is normal among dolichocephalic breeds. The shape of the palpebral fissure also depends on the relationship of the globe to the orbit. A small globe in a deep orbit allows a narrow palpebral fissure; the opposite occurs with a large globe in a shallow orbit. The NM aids in protection of the conjunctiva and cornea by moving, either passively or actively, over the cornea when the globe is retracted. Eyelid closure is mediated by the efferent fibers of the facial nerve (CN VII) and their effects on the orbicularis oculi muscles. The oculomotor nerve (CN III) innervates the levator palpebral superioris, which is responsible for opening the upper eyelids. Eyelid closure is the end result of two eyelid reflexes, the corneal and palpebral reflexes, and the menace response (Table 2.1). The corneal and palpebral reflexes are primitive reflexes with a purely subcortical course. Both are elicited by touch, with the afferent pathway being the ophthalmic branch of the trigeminal nerve (corneal) or the ophthalmic and maxillary branches of the trigeminal nerve (palpebral). The efferent pathway of these two reflexes as well as the menace response is the facial nerve stimulating the orbicularis oculi muscles, resulting in a blink. These reflexes are present immediately following birth or eyelid opening. In contrast, the menace response is cortically mediated and is initiated by a threatening gesture or loud sounds. Table 2.1 Reflexes involving the blink response. a If sufficient cortex of one cerebral hemisphere is damaged, the menace reaction cannot be elicited in the contralateral eye of the dog. Pathology of the cerebellar cortex can also affect the menace reaction. b The menace response can also involve turning the head or moving away from the stimulus. Table 2.2 Blinking rates of domestic animals. a Dogs have partial blinks every few seconds between complete blinks. Blinking and blink rates have been studied in many species under varying circumstances and methodologies, making comparisons and generalized statements difficult (Table 2.2). Blinking does not occur randomly, and blinks are often associated with gaze shifts and saccades. One of the theories for this timing is that blinking temporarily blocks visual information, and blinking during gaze shifts and saccades takes advantage of blocking vision when the images are already degraded from movement. Diurnal nonhuman primates and birds have higher blink rates than nocturnal nonhuman primates and birds, and in general, larger mammals and primates blink more often than smaller mammals. Blink rates and evaporation of the PTF can affect topical drug activity. In dogs, the larger upper eyelid, which contains the cilia or eyelashes, is more mobile than the lower eyelid. When the eyelids are closed, most of the ambient light is prevented from entering the eyes. Restrained dogs blink 10–20 times/min in comparison to unrestrained dogs. Some 50% of dogs’ blinks are incomplete. Puppies normally open their eyelids between 10 and 15 days of age. In cats, both eyelids lack cilia. The eyelids of pigmented cats allow no more than 5% of light at longer wavelengths to be transmitted. Kittens normally open their eyelids between 10 and 15 days of age; however, both eyes do not always open on the same day. The NM of the feline species is large and active, but in most other species it moves passively. In cats, it actively covers most of the cornea; it can extend at least two‐thirds of the way across the cornea and contains nine smooth muscles that lead to active retraction or protrusion. These smooth muscles draw the membrane into the medial canthus and are innervated exclusively by postganglionic adrenergic sympathetic nerve fibers, with cell bodies located in the anterior cervical ganglion. Their axons follow the oculomotor nerve. Normally, the NM shows no spontaneous activity in most species, because the smooth muscle lacks tight junctions like those of the visceral smooth muscle. Each muscle cell is innervated by one or more axons, thus confirming that activation of the smooth muscle in the NM is neurogenic and that the myogenic conduction normally found in visceral smooth muscles does not occur. Thus, cats are the only common domestic animal in which sympathetic stimulation will cause the NM to move. In horses, the cilia are long and numerous on the upper eyelid, except near the medial canthus. Horses blink at about 19 blinks/min, approximately one‐third of which are minimal incomplete, one‐third of which are moderate incomplete, and one‐third of which are complete, with only 6% of the blinks having a complete squeeze. Lid closure is approximately twice as rapid as lid opening. Eyelids are open at birth. Also, long tactile hairs, or vibrissae, are present on both the dorsal brow and lower eyelid. The vibrissae are long, stout, single shafts of hair that are usually thicker than adjacent skin hair; they may provide additional sensation for the eyelids. In cattle, sheep, and pigs, the upper eyelid is the most mobile, largest, and has the majority of cilia. Pigs, rabbits, rodents, and some ruminants have a deeper separate structure, the Harder’s gland or harderian gland, in addition to the superficial gland of the NM. This gland secretes lipids, porphyrins, indoles, and growth factors, and is thus also important for lubrication of the eye. In the pig, the meibomian glands are poorly developed, and the primary eyelid glands are sweat glands. Eyelids are open at birth in these species. In birds and certain reptiles, the lower eyelid is larger and more mobile than the upper eyelid. There are no feathers corresponding to eyelashes on the lids. The superciliary line refers to feathers that correspond to the eyebrow and are often of different colors than surrounding feathers. The superciliary or supraorbital ridge refers to the unfeathered bony protuberance just dorsal to the orbital rim that is seen in many raptors, such as eagles and hawks. The ridge is thought to provide shade to the eye. Birds may blink with both eyelids or the NM alone. In contrast to mammals, the thin nearly transparent NM of birds is under direct skeletal muscular control with two muscles extraneous to the lid that can pull the NM over the entire cornea as many as 15–20 times/min, even with the other eyelids closed. Blinks in peacocks are strongly associated with gaze shifts. The NM also contains a superficial tear gland, and some species have a deeper harderian gland. Chicks hatch with their eyes open. Both the optical and normal functions of the cornea depend on the integrity of the lacrimal system. The PTF maintains an optically uniform corneal surface by smoothing out minor irregularities, removing foreign matter from the cornea and conjunctiva, lubricating the conjunctiva and cornea, providing nutrients to the avascular cornea, and controlling the local bacterial flora. The PTF also undergoes constant evaporation and formation of transient “dry spots.” Hence, the rate of tear evaporation appears to be directly related to the rate of blinking, since the rate of blinking is faster than the development of these dry spots. Actual tear flow rates are difficult to measure in most species; however, in the horse, the tear flow rate has been estimated to be 34 μl/min with a tear volume of 234 μl, which indicates a turnover of the tear volume in approximately 7 minutes. By comparison, tear turnover and tear evaporation rates in humans are ~1 ± 0.4 and 0.14 ± 0.07 μl/min, respectively. In all species studied, the PTF can be loosely divided into three layers that intermix (Figure 2.1). The outer oily layer (~0.1 μm) is very thin and forms a reversible, noncollapsible, multilayer film with the primary purpose of stabilizing the air–tear interface (and preventing evaporation). The primary constituent of this lipid layer is the meibomian gland secretion (MGS), or meibum, a composite lipid‐rich mixture. Up to 22 wt% comprises nonlipid components (proteins, salts, and polysaccharides). The main lipid classes found in canine MGS are very long chain cholesteryl esters, wax esters, (O‐acyl)‐omega‐hydroxy fatty acids (OAHFAs), and cholesteryl esters of OAHFAs. Dogs have a relatively larger proportion of OAHFAs than humans, which could be related to a higher tear film stability and lower blink rate in dogs versus humans. The same types of molecules are found in the MGS of cattle, rodents, and marsupials. This outer lipid layer prevents not only evaporation of the underlying layers but also the overflow of tear film onto the eyelids, spreads over the aqueous subphase, imparts stability to the tear film, thickens the aqueous subphase, provides a smooth optical surface for the cornea, constitutes a barrier against foreign particles, provides some antimicrobial activity, and seals the lid margins during prolonged closure. Additionally, it prevents maceration of the lid skin by the tears. Figure 2.1 The tear film is a complex multilayered fluid phase. This figure represents the classic three‐layered model, composed of a mucin‐gel layer adjacent to the epithelial surface, an aqueous layer containing mucin and other soluble proteins, and a thin lipid film on the outermost surface. The middle aqueous layer (∼7 μm) is the thickest (>60% of the total tear film thickness) and performs the primary nutritional functions of the tear film. This layer is composed of ~98% water and ~2% solids, comprising predominantly proteins. The aqueous layer contains inorganic salts, glucose, urea, proteins, glycoproteins, and soluble mucins. The lacrimal gland, gland of the NM, harderian gland, and accessory lacrimal glands in the conjunctiva all contribute to its formation. Destruction or excision of the canine lacrimal gland or NM gland results in a variable reduction in aqueous tear production, and indicates that approximately two‐thirds of the aqueous tear production is produced by the lacrimal gland, approximately one‐third by the gland of the third eyelid, and a very minor amount by the accessory lacrimal glands in the conjunctiva. The aqueous portion is evaluated clinically primarily through use of the Schirmer tear test (STT) I; the phenol red thread test can be used in very small animals. The deep, or mucin, layer (∼1 μm) is composed of tear mucins produced by the apocrine conjunctival goblet cells, as well as an underlying glycocalyx that is associated with the corneal and conjunctival microvilli. The distribution of goblet cells varies among species, but the fornix is rich in goblet cells in dogs, cats, and horses, whereas the highest density in chinchillas and guinea pigs is in the palpebral conjunctiva. All species have lower concentrations of goblet cells in the bulbar conjunctiva. Mucin is produced by goblet cells in response to mechanical, immune, histamine, antigenic, or (direct or indirect) neural stimulation. The glycocalyx comprises polysaccharides that are produced by the stratified squamous epithelial cells of the cornea and conjunctiva and project from the surface microvilli of those cells. Mucins play a critical role in lubricating the corneal surface, thus making its hydrophobic surface more hydrophilic (to permit spreading), and in stabilizing the PTF. The mucin layer as well as the integrity of the outermost layer of corneal epithelium is necessary for retention of the tear film on the cornea. Tears are a clear and slightly alkaline solution, with a mean pH of 8.3, 8.1, and 7.8 in cattle, dogs, and horses, respectively. In humans, horses, cattle, and rabbits, tear electrolyte concentration is similar to that in plasma, except for potassium, which is three to six times more abundant in tears, thus indicating an active transport mechanism. Tear film osmolarity/osmolality is influenced by the rate of tear secretion, evaporation, and composition. It is similar in cats (329 mOsm/l), dogs (356 mOsm/l), and rabbits (376 mmol/kg), whereas humans (283 mmol/kg) and horses (284 mmol/kg) have a lower osmolarity. The PTF contains both nonspecific and specific antimicrobial substances. Nonspecific substances include lysozyme, lactoferrin, α‐lysine, and complement. Specific antimicrobial substances include secretory immunoglobulins A, G, and M. Toll‐like receptors that play a role in the defense against many types of microbial infections are expressed by the corneal and conjunctival epithelial cells in humans and horses. Protein concentrations in canine tears average 0.35 g/dl, with 93% globulin, 4% albumin, and 3% lysozyme, which is a ubiquitous antibacterial enzyme that hydrolyzes bacterial cell walls. Lysozyme is produced by the conjunctival goblet cells and has antibacterial and antifungal properties; its concentration increases with conjunctivitis. Relative to humans and nonhuman primates, domestic animals have very low amounts of lysozyme (e.g., the horse has one‐half to one‐fourth that of human tears) and the cat has none. Lysozyme activity has not been detected in cattle, but it has been detected in sheep and goats. Lactoferrin has been identified in the PTF of humans, dogs, cats, cattle, and other mammals, and reversibly binds the iron that is available for bacterial metabolism and growth. Immunoglobulin A (IgA) contributes to ocular defense by coating bacterial and viral microorganisms, leading to agglutination, neutralization, and lysis. IgA is present in greater concentrations in the PTF than immunoglobulins G and M. Cat tears have a 6.6 mg/ml total protein concentration with 9.7% IgA. The lacrimal nerve, a branch of the trigeminal nerve, is primarily sensory but also provides the lacrimal gland with its parasympathetic (release acetylcholine and vasoactive intestinal peptide neurotransmitters) and sympathetic (release norepinephrine and neuropeptide Y neurotransmitters) innervation. Both adrenergic and cholinergic distribution patterns around the acini and blood vessels of the canine lacrimal gland are similar; however, the cholinergic fibers appear to be greater in number than the adrenergic fibers. Lacrimation is stimulated by painful irritants, eye diseases, mechanical or olfactory stimuli of the nasal mucous membranes, and sinus diseases. Tear production as assessed with the external ocular surfaces anesthetized and the lower conjunctival fornix dried by Dacron swabs (STT II) measures ~50% of that measured without manipulation (STT II) in the cat and dog. Larger dogs also have greater wetting per minute than smaller dogs as measured with STT I. Additionally, canine neonates have lower tear production than adults. Clinical estimation of the rate of evaporation (and, indirectly, of the mucus component of the PTF) is performed through determining the time (in seconds) for the tear film using topical fluorescein to break up (development of “dry spots”). The nasolacrimal drainage system eliminates used tear film and any excessive tears. The PTF accumulates along the palpebral margin of each eyelid and is forced by blinking to move medially into the upper and primarily the lower lacrimal puncta. When the tears are in the lacrimal pool and the facial muscles relax, the tears flow into the lacrimal canaliculi by capillary action. Normal breathing movements also facilitate this flow into the canaliculi. Reflex blinking of the eyelids closes the lacrimal sac, which acts as a passive pump. Pseudoperistaltic motion of the nasolacrimal duct allows movement of the tears into the nasal cavity. Autoregulation of the lacrimal system with receptors in the excretory portion has been suggested in studies of human tear flow. Evaluation of canalicular function in humans suggests that destruction of either canaliculus alone does not affect excretion of tears; in domestic animals, the lower canaliculus is considered to be the primary site for tear drainage. The clear cornea serves as a window for the eye with two critical optical properties, transparency and refractive power, both of which are essential for vision. The cornea, with the sclera, protects the inner components of the eye from injury through its exquisite structure, biomechanics, and sensitivity. The cornea serves as the most powerful refractive structure of the eye. Corneal clarity or transparency is a result of the lattice‐like organization of the stromal collagen fibrils as well as the transparency of the cells within the cornea (Figure 2.2). The state of relative dehydration, hypocellularity, unmyelinated nerve fibers, a nonkeratinized epithelium, and absence of blood vessels and pigment also contribute to corneal transparency. The corneal stroma comprises the bulk of the cornea and is responsible for 90% of its thickness. It is predominantly composed of water that is stabilized by an organized network of collagens, glycosaminoglycans (GAGs), and glycoproteins. The GAGs are important for maintaining the regular spacing between fibrils. The uniform thickness, small collagen fibrils arrange into parallel lamellae running at oblique angles to each other, and are separated by less than a wavelength of light (Figure 2.3). This formation results in a highly ordered, lattice‐like arrangement whereby short‐range order results in corneal transparency via destructive interference. Figure 2.2 In the normal cornea (a), a cross section of the corneal fibrils demonstrates a nearly perfect lattice arrangement, with equidistant collagen fibrils permitting light transmission and concomitant transparency. In contrast, swelling of the cornea with edema (b) disrupts this highly ordered arrangement, resulting in light diffraction and an opaque, bluish cornea. Quiescent keratocytes lie between collagenous lamellae to form a closed, exquisitely structured syncytium. These three‐dimensional, stellate‐shaped cells comprise a cell body with multiple, extensive dendritic processes that interact with other keratocytes. Abundant corneal crystallins (~25–30% of the intracellular soluble protein), such as aldehyde dehydrogenase and transketolase, minimize refractive differences in the keratocyte cytoplasm, thus ensuring transparency of these cells. Figure 2.3 Schematic of collagen fiber organization in the canine cornea. The epithelium produces an anterior basement membrane with a complex surface topography consisting of a meshwork of fibers and holes. The anterior 10% of the cornea comprises unidirectional, interwoven collagen lamellae, while the posterior 90% consists of unidirectional, nonwoven collagen lamellae with a random orientation. Descemet’s membrane, the specialized basement membrane of the endothelium, can be divided into the anterior banded and posterior nonbanded layers. The anterior banded layer is dominated by collagen VIII, which appears as a hexagonal network en face and parallel bands in transverse section. The surface topography posterior nonbanded layer has a rich network of intertwined fibers, but with a smaller pore size in comparison to the anterior basement membrane. Upon corneal wounding, transformation of keratocytes to activated fibroblasts and myofibroblasts results in a dramatic increase in cell volume and subsequent dilution of corneal crystallins, with a concomitant increase in light scatter. Corneal scarring is thought to be due to alterations in the light scattering properties of keratocytes, in addition to changes to the extracellular matrix (ECM). The epithelium and endothelium are responsible for maintaining the cornea in a relatively dehydrated state. Specifically, loss of the corneal epithelium or endothelium results in a 200% or 500% increase in corneal thickness, respectively, due to stromal edema. Anatomical integrity of the epithelium and endothelium provides two‐way physical barriers against the influx of tears and AH, respectively. However, the multiple‐layered epithelium provides a relatively impermeable barrier versus the leaky, single‐layered endothelium. The endothelium primarily maintains stromal deturgescence via active transport that is energetically maintained by sodium–potassium‐activated adenosine triphosphatases (Na+–K+‐ATPases). Steady‐state hydration in the cornea occurs when the endothelial leak and pump rates are equivalent; this process is termed the “pump–leak” mechanism. The leaky barrier function of the endothelium may at first seem counterintuitive, but most nutrients for the cornea, except oxygen, come from the AH. Thus, leakiness of the endothelium is essential to providing bulk fluid flow through a tissue that lacks blood and lymphatic vessels. Glucose transporters are found on both the apical and basolateral endothelial cell membranes that face the AH and stroma, respectively, to ensure transcellular glucose flux. The corneal epithelium converts glucose to glucose 6‐phosphate, where it is subsequently metabolized to pyruvate via glycolysis. Most of this pyruvate is then metabolized into lactate, but some is diverted into the tricarboxylic acid cycle to produce ATP. Glucose is also stored in the epithelium as glycogen, which can be used for energy under stressful conditions such as corneal injury. The corneal epithelium and keratocytes in the anterior stroma obtain oxygen for aerobic glycolysis from the PTF, while the endothelium and keratocytes in the posterior stroma receive their oxygen from the AH. The cornea is a thick‐walled, pressurized, partially intertwined, unidirectionally fibril‐reinforced laminate biocomposite, which imparts stiffness, strength, and extensibility to withstand both inner and outer forces that may alter its shape or integrity. A soft, fibrous connective tissue, like the cornea, usually is much stronger in the parallel versus perpendicular direction to the collagen fibrils. Consequently, the collagen fibrils are arranged into complex hierarchic structures, which give the cornea its anisotropic mechanical properties. The collagen lamellar architecture of the cornea varies dramatically between vertebrate species, with nonmammalian vertebrates exhibiting an orthogonal‐rotation arrangement with a marked increase in lamellar branching in species such that birds >> reptiles > amphibians > fish; in contrast, the mammalian species exhibit a random pattern. Tissues are biomechanically characterized by measuring the elastic modulus, a property that defines a material’s ability to resist deformation under an applied stress, which approximates its stiffness. The elastic moduli of the corneal layers as measured by atomic force microscopy have been reported in the human and the rabbit (Table 2.3); all layers of the human cornea were stiffer than those of the rabbit. This variability in corneal collagen fiber organization and matrix properties between species likely contributes to their diverse mechanical properties, and may influence indentation tonometry (estimation of intraocular pressure [IOP]). The cornea is an exquisitely sensitive tissue, and this sensitivity provides a critical protective function. Upon stimulation of the cornea, involuntary blinking occurs via intermediate relays from the ophthalmic branch of the trigeminal nerve to orbicularis oculi innervation from the facial nerve – a fundamental reaction termed the corneal or blink reflex. Concomitant with the blink reflex is reflex tearing from parasympathetic innervation to the lacrimal gland. During extreme pain, the corneal reflex is exaggerated, and blepharospasm sometimes occurs such that the eyelids cannot be opened voluntarily. Corneal sensitivity varies by species, region of the cornea, and, in the dog and cat, skull conformation. For example, corneal sensitivity in dogs, as measured by the Cochet–Bonnet esthesiometer and histology of the corneal nerves, was highest, intermediate, and lowest in the dolichocephalic, mesaticephalic, and brachycephalic skull types, respectively. Similarly, the central cornea is less sensitive in brachycephalic cats than domestic shorthair cats. Corneal sensitivity is greatest in the central cornea and lower in the peripheral cornea. Table 2.3 Elastic moduli of layers of the cornea as determined by atomic force microscopy in rabbits and humans. The cornea is one of the most richly innervated tissues in the body. Most corneal nerve fibers are sensory in origin and respond to mechanical, chemical, and thermal stimuli via the ophthalmic branch of the trigeminal nerve. However, a small proportion of nerves are sympathetic or parasympathetic in origin and derive from the superior cervical ganglion or ciliary ganglion, respectively. Corneal nerve organization is similar across mammalian species, with only minor interspecies differences. All mammalian corneas contain a dense limbal plexus, multiple radially directed stromal nerve bundles, a dense highly anastomotic subepithelial plexus, and a richly innervated epithelium (Figure 2.4). In the dog, corneal innervation arises from the corneal limbal plexus, which comprises a 0.8–1 mm wide, ring‐like band, surrounding the peripheral cornea. The majority of sensory fibers that innervate the cornea are activated by a variety of exogenous mechanical, chemical, and thermal stimuli, as well as endogenous factors released by tissue injury, and are thus termed polymodal nociceptors. The remainder of the sensory fibers innervating the cornea comprise mechano‐nociceptors and cold thermal receptors, which are only activated in response to mechanical forces or changes in temperature, respectively. In addition to their contributions to corneal protection via the blink reflex and reflex tearing, corneal nerves maintain corneal epithelial health through the secretion of trophic factors and maintenance of basal tear secretions. Pupillary functions include regulating light entering the posterior segment of the eye, increasing the depth of focus for near vision, and minimizing optical aberrations by the lens. The iris muscles consist of a constrictor (sphincter) that encircles the pupil and radial dilator muscles to expand the pupil. The sphincter muscle is an annular band of smooth muscles near the pupillary margin of the iris and is derived from neural ectoderm. The dilator muscle, also derived from neural ectoderm, consists of a series of myoepithelial cells that extend from near the pupillary margin to the base of the iris and are contiguous posteriorly with the pigmented epithelium (PE) of the ciliary body. Pupil size varies on the basis of the balance between these two muscle groups. The constrictor muscle, which is the stronger of the two, is innervated by the oculomotor nerve (CN III) and provides primarily parasympathetic control; in contrast, the dilator muscle is innervated primarily by sympathetic nerves. The constrictor muscle causes miosis, and the dilator muscle is responsible for mydriasis. Bright light decreases pupil size. The sympathetic activity in the iridal dilator muscle and ciliary body musculature (discussed later) is mediated by a combination of β‐receptors (β1 and β2) and α‐receptors (α1 and α2). Components of the pupillary light reflex are listed in Table 2.4. Figure 2.4 Schematic of corneal innervation. The limbal plexus is a ring‐like band of predominantly myelinated fibers in the sclera adjacent to the cornea. From the limbal plexus, nerve fibers enter into the corneal stroma as nerve bundles and lose their myelin as they traverse to the central cornea. The subepithelial plexus is a dense, anastomosing network of thin axons immediately underlying the anterior basement membrane. The subepithelial plexus gives rise to the subbasal plexus, a whorl‐shaped network of axons between the anterior basement membrane and basal epithelium where nerve fibers run horizontally as long parallel nerves, termed leashes. The axons of the subbasal plexus then vertically ascend to terminate in various layers of the epithelium. Species differences of the α‐ and β‐receptors have been demonstrated among humans, rabbits, nonhuman primates, cats, and dogs, and they are summarized in Table 2.5. These receptors alter the effects of drugs on the eye. For example, feline pupils constrict with the use of timolol, a nonselective β‐adrenergic antagonist, because the feline iris sphincter muscle has primarily β‐adrenergic nerve fiber. Because β‐adrenergic nerve fibers are inhibitory to the sphincter muscle, the miosis in response to topically applied timolol is suspected to be the result of its antagonism of inhibitory input to the sphincter muscle. Most synapses in the ciliary ganglion are involved in relaying impulses that result in accommodation; the remainder are concerned with constriction of the pupil. Endogenous prostaglandin F2α appears to be involved in maintaining muscle tone in the sphincter muscle of the iris. Prostaglandins most likely act directly on these muscles, and they appear to act to a lesser extent on the dilator muscles of the canine iris. Exogenous prostaglandin analogues cause miosis in cats, dogs, and horses, and the receptors have been detected in the iris and ciliary body of several mammals. Iris color, or the amount of melanin, influences the effects of many drugs, as melanin can bind drugs, increasing or prolonging their time to onset and duration. Table 2.4 Components of the pupillary light reflex. Table 2.5 Adrenergic receptors in the iris and ciliary body. Pupil shape also varies among species. Vertical pupils are most commonly seen in terrestrial mammals and reptiles that are ambush predators, and are diurnal. Prey species tend to have horizontally elongated pupils. These respective variations are thought to keep images on the vertical and horizontal contours sharp. In domesticated cats, the constricted pupil is a vertical slit, whereas in the larger, wild felines, it is circular. On dilation, the vertical sides of the domestic feline pupil expand to produce a circular pupil. The constrictor muscle fibers are vertically oriented, and therefore contraction leads to a vertically oriented slit pupil. In young horses, the pupil is more circular than in adults. Under illumination, the ends of the oval pupil of mature horses do not constrict, but the dorsal and ventral borders do. In bright daylight, the superior granula iridica occludes the central papillary opening, resulting in two apertures and assisting with focusing through the creation of Scheiner’s disc phenomenon. With very low illumination or administration of a mydriatic, the dorsal and ventral borders of the pupil dilate, thereby forming a circular pupil. The avian pupil is circular and highly motile. The consensual pupillary reflex is usually absent (because of total decussation of nerve fibers at the optic chiasm), but occasionally a strong beam of light may traverse the posterior ocular layers and the thin medial orbital bones to stimulate the opposite retina. As the constrictor and dilator muscles are mainly striated with varying amounts of nonstriated fibers, the avian pupil is not affected by traditional mydriatic agents, but it can be dilated variably by various neuromuscular‐blocking drugs. While allowing light transmission through the eye, nutrients are provided and waste is removed by two systems of blood vessels (i.e., retinal vessels and uveal vessels), the formation and egress of AH, and the vitreous body. Intraocular tissues lack a typical lymphatic system, and the uveal tract (i.e., iris, ciliary body, and choroid) provides this function. The choroid, ciliary body, and iris are supplied primarily by the uveal vessels. The outer retina in some animals (e.g., dogs, cats, ruminants, and pigs) and almost all or the entire retina in others (e.g., horses and guinea pigs) is nourished by diffusion from the choroidal vessels. The inner retina is supplied by retinal vessels in many species. Blood vessels supplying the cornea and lens in the embryo regress before birth or shortly thereafter, leaving the AH as the primary source of nutrients for the cornea and lens. Birds have a unique structure, the pecten oculi, which is a heavily pigmented, highly vascularized, and usually fanlike structure projecting from the surface of the optic nerve into the vitreous. A similar structure occurs in reptiles, termed the conus papillaris. The avian pecten likely functions as an important source of nutrients for the inner retina. This assumption is based on the observations that the avian retina is thicker than oxygen could perfuse from the choroid and that the pectinate artery resistive and pulsatility indices are low. Several marine mammals, the bottlenose dolphin (Tursiops truncatus), spotted seal (Phoca largha), and California sea lion (Zalophus californianus), have an ophthalmic rete from which the retinal and choroidal arteries are derived. The vascular pressure promoting flow, the resistance of blood vessels, and the viscosity of the blood all influence the blood flow through all tissues, including the eye. The pressure head for blood flow (i.e., perfusion pressure) in most tissues is the difference in pressure between the arteries and the veins. However, in the eye, the IOP approximates the venous pressure, so the perfusion pressure is the difference in pressure between the small arteries entering the eye and the IOP. Of clinical importance is that the perfusion pressure to the eye is reduced by lowering the blood pressure or raising the IOP, as occurs in glaucoma. Studies of hemodynamics in the rabbit ophthalmic artery demonstrate that autoregulation maintains normal blood velocity and resistance when the IOP is below 40 mmHg. However, at higher pressures the autoregulatory capacity is limited. As a result, an IOP of about 15–17 mmHg is related to episcleral venous pressure of about 10–12 mmHg and about 5–7 mmHg of resistance from passage through the AH pathways! In most species, the major arterial circle of the iris is formed by the nasal and temporal long posterior ciliary arteries. The iris and ciliary body receive approximately 1% and 10%, respectively, of the total ocular blood flow. In humans and rabbits, additional iridal blood flow occurs from the anterior ciliary arteries via the extraocular muscles (EOMs). Blood flow to the ciliary body in most species that have been studied is provided by the iridal major arterial circle, branches of the anterior ciliary arteries, and branches of the long posterior ciliary arteries. The cat and monkey iris and ciliary body have autoregulation of their blood flow. Carbon dioxide dilates the anterior uveal vessels, and sympathetic α‐adrenergic receptors cause vasoconstriction in the anterior uvea. Parasympathetic muscarinic receptors and prostaglandins, however, cause vasodilation. Prostaglandins E1 and F2α appear to cause a two‐ to threefold increase in blood flow to the anterior uvea when applied topically. The outer retina (and the entire retina in some species) depends heavily on choroidal blood flow for nutrients and waste removal. In the animal species studied, most of the blood supply to the choroid is supplied by the short posterior ciliary arteries, but some of the peripheral choroid receives blood from the major arterial circle of the iris. The choroidal capillaries are fenestrated and large (diameter 15–50 μm). These vessels are highly permeable and permit glucose, proteins, and other substances (including fluorescein) of the blood to enter the choroid. Within the choroid, these proteins create a high osmotic pressure gradient that assists in removal of fluids from the retina. The short posterior ciliary arteries appear to supply well‐defined territories within the choroid. As a result, these “watershed zones” can develop with marked elevations of IOP (often >50 mmHg), and appear in the dog and nonhuman primate as pyramidal‐shaped areas of choroidal and retinal degeneration extending from the optic nerve head. The rate of uveal blood flow is rapid (1.2 ml/min in the cat), with a mean combined retinal and choroidal circulation time of 3–4 s. In monkeys, 95% of the ocular blood flows through the uveal tract, of which 85% is through the choroid. With this high rate of blood flow, oxygen extraction from each millimeter of blood is low (∼5–10%). The oxygen content of choroidal venous blood is 95% of that in arterial blood. Reduced flow rates result in higher oxygen extraction, so that total extraction is reached. This protects the oxygen supply to the retina, and it also protects the eye from light‐generated thermal damage. Choroidal vessels have little to no autoregulatory mechanisms, but carbon dioxide is a potent vasodilator of choroidal vessels. Choroidal vessels are under the strong influence of sympathetic stimulation, which can result in a 60% reduction of choroidal blood flow. The α‐adrenergic drugs cause vasoconstriction of choroidal vessels, but β‐adrenergic drugs have no effect. The retina receives 4% of the ocular blood flow in the monkey. In cats, 20% of the oxygen consumed by the retina is delivered through the retinal circulation and the remaining 80% is delivered via the choroidal circulation. Similar data are not available for other domestic animals. Blood flow in the innermost retina is practically unaffected by moderate changes in perfusion pressure. Autoregulation of retinal blood flow is extensive in the cat, monkey, and pig, and protects the retinal circulation from large variations in perfusion pressure. Both metabolic and myogenous autoregulation are present in the eye. Metabolic control of retinal blood flow is similar to that of blood flow to the brain. In the cat, maximum retinal vasodilation occurs with an increased Blood flow of the optic nerve head is usually provided primarily by branches from the short posterior ciliary arteries. In humans, cats, and rabbits, optic nerve head blood flow possesses autoregulation over a wide range of IOPs (∼30–75 mmHg), but in humans, this autoregulation is most efficient when IOP is 6–30 mmHg. Ocular perfusion pressure, the relationship between systemic blood pressure and IOP, determines blood flow in the optic nerve head. The autoregulatory capacity of optic nerve head blood flow is more susceptible to an ocular perfusion pressure decrease induced by lowering the blood pressure, compared with that induced by increasing the IOP. Studies of blood flow in the optic nerve head have been limited by the small tissue mass involved. The optic nerve head is subjected to several different pressures as well as to the tissue stress at the different levels of the scleral lamina cribrosa. Blood–ocular barriers contain endothelial and epithelial tight junctions with varying degrees of “leakiness.” These barriers prevent nearly all protein movement and are effective against low molecular weight solutes such as fluorescein and sucrose. The complexities of these structures differ between the various vascular beds, which allow movement of some substances from one compartment to the other. The two primary barriers within the eye are the blood–aqueous barrier (BAB) and the blood–retinal barrier (BRB). With inflammation, these barriers may be compromised, and allow fibrin and other proteins into the ocular tissues and space. Other minor barriers of the eye exist as well. The zonula occludens of the corneal epithelium prevents the movement of ions and therefore fluid from the tears into the stroma, prevents some evaporation, and protects the cornea from pathogens. The partial obliteration of the intercellular spaces provided by the macula occludens of the corneal endothelial cells prevents bulk flow of AH into the corneal stroma but allows moderate diffusion of small nutrients and water. The BAB depends primarily on the tight junctions in the nonpigmented ciliary body epithelium, the nonfenestrated iris capillaries, and the posterior iris epithelium. The anterior BAB in the iris allows transcellular transport by means of vesicles. Paracellular transport is controlled by tight junction extensions. The anterior surface of the iris does not serve as a barrier as it does not have a continuous cellular layer. The epithelial portion of the BAB is the inner, nonpigmented ciliary epithelium, and it controls the flow of fluid into the posterior chamber. The BAB is less effective than the retinal epithelial barrier, because protein can pass into the AH through leakage in other parts of the anterior uvea. Both the ciliary body and choroidal blood vessels are highly fenestrated and thus leak most of their plasma components, including protein, into the stroma. No barrier is present between the AH and the vitreous humor, which allows the diffusion of solutes from the posterior aqueous into the vitreous humor, or between the anterior uvea and the sclera. Breakdown of the BAB is seen clinically as an “aqueous flare” in anterior uveitis or secondary to loss of AH, as in anterior chamber paracentesis. The tight junctions between the retinal pigmented epithelial cells comprise the bulk of the epithelial portion of the BRB. The nonfenestrated retinal capillary endothelium with tight junctions between the cells comprises the endothelial portion of the BRB. The most permeable point of the BRB is the optic nerve head, at which substances from the choroid can pass into the nerve. The choroidal capillaries are highly permeable to permit passage of all low molecular weight compounds and proteins. Thus, nutrients from the choroidal blood supply pass readily into the retinal pigment epithelium, where numerous transport systems account for the selectivity of the barrier and elaborate transcellular pathways exist to pass them on into the retina. This high protein permeability of the choroidal vessels also elevates osmotic pressure, which helps fluid to pass out of the retina. The transport of water from the retina to the choroid is driven by the active transport of chloride to prevent water accumulation in the subretinal space. No significant barrier exists between the vitreous body and the retina. In the normal eye, IOP is generated by flow of AH against resistance, and is necessary to maintain the appropriate shape and optical properties of the globe. AH is a clear, colorless liquid that fills the anterior and posterior chambers as well as the pupil. It has a refractive index of 1.335, which is slightly denser than water, and is a critical constituent of the eye’s optical system. As AH is formed by the ciliary body processes, it enters the posterior chamber and flows through the pupil into the anterior chamber, where it leaves the eye through the corneoscleral trabecular and uveoscleral outflow pathways. The rate of AH formation equals the outflow, so the IOP is maintained relatively constant, and the refractive surfaces of the eye are kept in a normal position. This continuous flow of AH supplies the avascular cornea and lens with nutrients and also removes their waste products. A convection current exists within the anterior chamber whereby warm AH circulates from the pupil downward adjacent to the air‐cooled cornea and upward near the lens where the temperature is warmer. This thermal circulation is responsible for the deposition of cellular material – termed keratic precipitates – on the inferior aspect of the corneal endothelium. The ciliary body has several critical functions, including production of AH by active secretion, ultrafiltration, and diffusion; generation of IOP through the aqueous dynamic process; influencing through its musculature the conventional (i.e., corneoscleral trabecular meshwork [TM] or pressure‐sensitive) AH outflow; provision of blood and nerve supplies for the anterior segment; control of accommodation via its musculature; formation of the BAB; and provision of the entry for nonconventional (i.e., uveoscleral or pressure‐insensitive) AH outflow. Furthermore, the ciliary body is also rich in antioxidant systems, with significant concentrations of catalase, superoxide dismutase, and glutathione peroxidase types I and II. In addition, the ciliary body is the major drug detoxification center in the eye, with its microsomes containing the cytochrome P450 proteins, which catalyze many drugs. The bilayered ciliary epithelium, consisting of the outer PE and inner nonpigmented epithelium (NPE), is the site for AH secretion. At their apical borders, the PE and NPE connect via gap junctions to form an intricate network (Figure 2.5). Adjacent NPE cells are joined by tight junctions to form a barrier that inhibits paracellular diffusion. AH is formed by three basic mechanisms: (i) diffusion, (ii) ultrafiltration, and (iii) active secretion by the NPE. The processes of diffusion and ultrafiltration form the “reservoir” of the plasma ultrafiltrate in the stroma of the ciliary body, from which the AH is derived via active secretion by the ciliary epithelium. Energy‐dependent active transport is required to secrete solutes against a concentration gradient across the basolateral membrane of the NPE; it is the most important factor in AH formation. Two enzymes critical in this process, Na+–K+‐ATPase and carbonic anhydrase (CA), are abundantly present in the NPE. The Na+–K+‐ATPase is membrane bound and is found in the highest concentrations along the basolateral interdigitation of these cells. Inhibition of the Na+–K+‐ATPase with cardiac glycosides (e.g., ouabain) or vanadate causes a marked decrease in aqueous formation. Figure 2.5 Schematic of AH production across the PE and NPE of the ciliary body. Note the position of the critical enzyme Na+–K+‐ATPase on the basolateral enzyme of the NPE. CA, also critical to AH formation, is abundant in the cytoplasm of both the NPE and PE. Ion transporters and channels facilitate transfer of Na+, K+, chloride (Cl−) and bicarbonate (HCO3 −) into, between, and out of the NPE and PE, while aquaporins enable water movement. Relative solute concentrations that most markedly differ between aqueous humor and plasma can be found at the bottom. Due to the primary active secretion of sodium, other molecules and ions cross over the epithelium by secondary active transport. As a consequence, increased concentrations of ascorbate, amino acids, and chloride are observed in AH relative to plasma in most mammalian species. Electroneutrality is maintained by anions accompanying the actively transported sodium; channels allow passage of chloride on the basolateral NPE membrane and a passive transporter exchanges bicarbonate for chloride. CA is abundant in the cytoplasm and on the basal and lateral membranes of the NPE and PE and catalyzes the following reaction: Formation of bicarbonate by CA is essential for secretion of AH, such that inhibition of CA results in decreased active transport of sodium by the NPE; it is unclear how this process occurs, although several hypotheses exist. Topical and systemic CA inhibitors substantially decrease AH production, therefore reducing IOP, and are thus useful in the management of glaucoma in animals and humans. As an ultrafiltrate of plasma, the compositions of AH and plasma are similar, with a few notable exceptions: a low protein concentration, high ascorbate and lactate concentrations, and reduced amounts of urea, glucose, and nonprotein nitrogen occur within AH versus plasma (Figure 2.6). Thus, breakdown of the BAB modifies the composition of the AH, primarily by the addition of proteins and prostaglandins, and increases light scattering. The resultant Tyndall effects makes a slit‐lamp beam evident within the anterior chamber, an observation clinically known as “aqueous flare.” With the addition of proteins, the aqueous composition closely approximates that of plasma and is termed plasmoid aqueous. Plasmoid aqueous in domestic animals forms fibrin clots easily due to high concentrations of the glycoprotein fibrinogen. Unless treated pharmacologically, these fibrin clots can cause numerous complications, including anterior and/or posterior synechiae or adhesions between the iris and the cornea and/or lens. Figure 2.6 AH drainage occurs via the traditional and uveoscleral outflow pathways in the iridocorneal angle of the dog. The ciliary body epithelium produces AH, which flows from the posterior chamber, through the pupil, and into the anterior chamber. Then, AH drains through the pectinate ligament to enter the TM. In the traditional outflow pathway, AH enters the AAP to drain anteriorly to the episcleral and conjunctival veins or posteriorly into the scleral venous plexus (SVP) and vortex veins. With uveoscleral outflow, AH flows through the ciliary muscle interstitium to the supraciliary and suprachoroidal spaces to diffuse out the sclera. In addition to protein and ascorbate, other organic compounds constitute the AH, and their concentrations vary relative to plasma. In most mammalian species, the concentration of amino acids in the AH is higher than that in the plasma, suggesting that active transport of amino acids is occurring across the ciliary epithelium. In the dog, however, amino acid concentrations are less in AH than in plasma. In this species, the vitreous may act as a “sink” for some of the amino acids, thus causing the deficiency. The major cations in the AH are sodium, potassium, calcium, and magnesium, with sodium comprising 95% of the total cation concentration. Sodium enters the AH via active transport, with a net flow of water into the posterior chamber. The major anions in AH are chloride, bicarbonate, phosphate, ascorbate, and lactate. The chloride and bicarbonate ions enter with sodium, but their concentrations vary among species. The rate of aqueous formation by the ciliary epithelium is influenced by sympathetic and parasympathetic innervation as well as humoral mechanisms to maintain a steady‐state IOP. Adrenergic regulation of AH formation is complex and the role of some receptor subtypes remains unclear. The β‐adrenergic antagonists, such as timolol, lower IOP by decreasing AH production. During sleep, AH formation decreases ~50% via modulation of the β‐arrestin/cAMP signaling pathway by β‐adrenergic receptors in humans and reducing the ocular hypotensive effects of timolol when instilled at night. Thus, IOP exhibits a circadian rhythm, which varies depending on whether animals are nocturnal or diurnal. For example, diurnal species such as dogs and nonhuman primates exhibit the greatest IOP during the early day, while in nocturnal species such as cats and rats IOP peaks at night. Cholinergic regulation of AH formation and composition is similarly ambiguous. For example, parasympathomimetic nerve stimulation or drugs have been demonstrated to increase, decrease, or not change the rate of AH production; these differences are likely due to species and technique‐related effects. Cholinergic agents may regulate amino acid transport from the blood to the AH as well as modulate inorganic ion concentrations within the AH. In aggregate, these studies suggest that the influence of parasympathetic drugs such as pilocarpine is relatively minor in AH formation and that their efficacy in decreasing IOP is likely due to increased AH outflow. AH dynamics involve the balance between production (i.e., active secretion) and outflow via the conventional and nonconventional routes (Figure 2.7). Conventional outflow consists of AH flow through the corneoscleral TM, while the nonconventional route involves uveoscleral outflow. Depending on the species, ~50–95% of AH drains through the TM via conventional outflow (Table 2.6). The TM is a complex, three‐dimensional structure comprising cells supported by an intricate ECM. The TM can be divided into three portions: uveal, the innermost portion; corneoscleral, the middle region; and the juxtacanalicular zone, the outermost section nearest the sclera (probably the site of greatest outflow resistance). The pore size of each TM zone decreases progressively from inward to outward, resulting in progressive increases in outflow resistance to the passage of AH. The juxtacanalicular zone consists of several endothelial cell layers that produce a matrix comprising GAGs, collagen, fibronectin, and other glycoproteins in which these cells are embedded. Thus, the juxtacanalicular zone, which is immediately adjacent to Schlemm’s canal in primates or the angular aqueous plexus (AAP) in most domestic animals, is thought to be the major site of aqueous outflow resistance. Figure 2.7 Chemical composition of the aqueous humor and lens. Water and protein are expressed as percentages of lens weight. Na+, Cl−, K+, and Ca2+ ions are expressed in microequivalent per milliliter of lens water. Other compounds are expressed in micromole per gram of lens weight or micromole per milliliter of aqueous humor. AA, amino acid; RNA, ribonucleic acid. Table 2.6 Estimates of aqueous humor dynamics in selected species. Other studies suggest that the main site of resistance to outflow is the endothelial lining of the AAP and its ECM. However, the site of filtration may be different from the site of flow resistance. AH transport through the endothelium of the AAP (or Schlemm’s canal in nonhuman primates and domestic chickens) is thought to occur via transcellular pores, large vacuoles, or pinocytotic vesicles. However, paracellular routes between the endothelial cells of Schlemm’s canal have also been proposed and may be pressure sensitive, particularly at higher IOPs. As the ciliary body produces AH, the tissues comprising the ICA resist AH outflow, thus generating IOP. Steady‐state IOP occurs when the rates of AH inflow and outflow are equivalent. The AH exits the eye by passive bulk flow via two routes in the ICA: The traditional pathway is dependent on IOP, while the uveoscleral pathway is not as long, since IOP is greater than 7–10 mmHg. At very low IOP, the net pressure gradient across the nonconventional pathway declines, so that uveoscleral outflow subsequently decreases. It is unknown why uveoscleral outflow is largely independent of IOP, but it may relate to complex relationships between pressure and resistance between the fluid compartments and the soft tissues that comprise this route. For example, the pressure gradient between the anterior chamber and suprachoroid is independent of IOP; thus, fluid flow between these compartments is also IOP independent. Uveoscleral outflow is primarily impacted by the state of the ciliary body and by the hydrostatic pressure difference between the anterior chamber and the suprachoroidal space. Contraction of the ciliary body musculature decreases unconventional outflow, possibly by reducing the extracellular spaces; in turn, relaxation increases outflow via this route. Thus, pilocarpine, a parasympathomimetic drug, and atropine, a parasympatholytic drug, will decrease and increase uveoscleral outflow by contracting and relaxing the ciliary body musculature, respectively. Because of general venous pressure, IOP in the eye under general anesthesia will decrease to only 10–12 mmHg. Table 2.7 Aqueous humor dynamics formulas. Formulas can be used to describe the formation and drainage of AH (Table 2.7). Episcleral venous pressure or the “backpressure” created by the venous portion of the conventional pathway in the AAP or Schlemm’s canal constitutes approximately 50–75% of the resistance (10–12 mmHg) that determines IOP. While minor anatomical variations in the venous system exist between species, results of pressure studies in humans, nonhuman primates, rabbits, and dogs reveal episcleral venous pressure to be between 8 and 12 mmHg. Arteriovenous anastomoses within the episcleral vasculature have been demonstrated in the rabbit, dog, owl monkey, and cynomolgus monkey. These vascular shunts may function in rabbits and dogs, where the episcleral vasculature appears to lack a capillary system, and in the monkey species as an emergency system to elevate IOP after globe perforation or to retrogradely flush the outflow channel. Episcleral venous pressure can be measured by direct cannulation (using very fine glass pipettes) or indirect partial to complete compression schemes (using a string‐gauge system or a fluid‐filled chamber). Results of limited studies indicate that the volume of the anterior chamber directly relates to the rate of aqueous outflow, so that animals with large eyes have faster outflow rates per minute. The resistance to aqueous outflow may be inversely proportional to the facility of outflow (C total). Cholinergic agonists such as pilocarpine decrease outflow resistance by contraction of the ciliary muscle and subsequent spreading of the TM. This effect is rapid, such that intravenous administration of pilocarpine to vervet monkeys results in a near‐instantaneous decrease in outflow resistance, suggesting that the effect may be mediated by a structure perfused by arteries. Ciliary muscle disinsertion and removal of the iris in nonhuman primates obliterate this acute response to pilocarpine, suggesting that it is mediated completely by ciliary muscle contraction rather than a direct effect on the TM. The M3 subtype of the muscarinic receptor is strongly expressed in the ciliary muscle and thought to mediate the changes in outflow facility in response to cholinergic agonists. Because the effect of cholinergic agonists on trabecular outflow (increase) is greater than that on uveoscleral outflow (decrease), the net effect is an increase in AH outflow and concomitant decrease in IOP. As expected, cholinergic antagonists, such as atropine, decrease traditional outflow and increase nontraditional outflow by similar mechanisms. Many other influences on the rate of AH formation and regulation of IOP have been proposed. For example, a center in the feline diencephalon has been found that, when stimulated, causes alterations in the IOP. Central nervous system (CNS) regulation of IOP is poorly understood, however, and hormonal control of AH production may be involved. Both invasive and noninvasive methods are used to investigate AH dynamics, and normative values have been described in domestic and laboratory animal species (Table 2.8). Invasive studies are more difficult to study in animals as the uveal tissues quickly respond to these “invasions” and can reduce the study objectives, but these early methods were essential and formed the basis for later noninvasive methods. They usually measured the dilution of intracamerally injected substances over short time periods. With the AH volume within the anterior and posterior chambers measured and the amount of dilution of the tracer estimated, the total amount of AH produced per unit of time could be determined. Knowledge of anterior and posterior chamber volumes is critical to determining the rate of AH production (Table 2.9). Fluorophotometry is a noninvasive method for studying AH flow dynamics, for evaluating ocular pharmaceutical agents used to treat glaucoma, and for determining iris permeability in both normal and disease states. Fluorophotometry of the anterior chamber and vitreous can noninvasively assess the permeability of the BRB in the normal and diseased eye. Fluorophotometry has been used extensively in humans, nonhuman primates, rabbits, cats, dogs, and, most recently, the red‐tailed hawk. This tool can also be used to assess permeability coefficients of the BAB involved in health and disease, determine the effects of selected drugs on the BAB, and mechanisms by which drugs affect the AH dynamics. Table 2.8 Methods to investigate aqueous humor dynamics. To determine AH outflow invasively, perfusion of the anterior chamber of in vivo and ex vivo eyes has been performed in numerous species. The constant pressure perfusion technique is the most frequently used. It involves maintaining a constant level of IOP with periodic, intermittent, or continuous minivolumes of perfusate. In the perfusion decay test, either a preselected volume of perfusate is injected or a preselected IOP is achieved. Once the perfusate has been injected, the time for the IOP to regain the baseline or preexisting measurement is obtained. In many ways, the perfusion techniques are similar to the noninvasive tonography methods (and yield similar results). Table 2.9 Comparative volumes of the chambers and select structures of the eye. The percentages reported for normal uveoscleral outflow range from 30% to 65% in nonhuman primates, 15% in dogs, 13% in rabbits, 4–14% in humans, and 3% in cats. The horse appears to have an extensive uveoscleral outflow system, but the volume and percentage of the total outflow system have not been reported. Often uveoscleral outflow is now calculated as the difference between applanation tonography and the results from fluorophotometry. Uveoscleral outflow pathway has been demonstrated using observable tracers measuring from 10.0 nm to 1.0 μm in diameter. As one would anticipate, the smaller‐diameter (i.e., pore) tracers penetrate into the different tissues to greater extents. After perfusion at different IOPs and for different time intervals, the eyes (especially the root of the iris, entire ciliary body, suprachoroidal space, and choroid, even as far posterior as the optic nerve) are examined by light microscopy, scanning electron microscopy, and transmission electron microscopy for these markers. These same methods have also demonstrated the ability of the trabecular endothelium and wandering macrophages to phagocytize particulate material within the outflow pathways. An alternative method to estimate the amount of uveoscleral outflow (either as μl or %) is by using radioactive isotopes injected into the anterior chamber; the time, amount of the isotope, or both are standardized. At the conclusion of perfusion, either the ocular tissues are dissected into the different sections and analyzed for radioactivity or the entire globe is sectioned and the radioactivity of each area is measured by scintillation counters. Another key concept in the measurement of IOP is ocular rigidity (k), or the resistance offered by the fibrous tunics of the eye (i.e., sclera and cornea) to a change in intraocular volume. Ocular rigidity may also be defined as the change in IOP per incremental change in the intraocular volume; this resistance manifests as a change in IOP. Ocular rigidity is determined by Schiotz indentation tonometry, and it estimates the change in volume (open manometer system) when the instrument is placed on the cornea as well as after injections of exact volumes or preselected elevations in IOP. With applanation tonometry, ocular rigidity is not a factor! This logarithmic relationship between IOP and volume of the globe is
2
Ophthalmic Physiology and Vision
Section I: Physiology of the Eye
Anterior Eye Structures
Eyelids
Cornea reflex
Palpebral reflex
Menace responsea
Dazzle reflex
Stimulus
Corneal touch
Eyelid touch
Menacing gesture
Bright light
Receptors
Somesthetic
Somesthetic
Photoreceptors
Photoreceptors
Afferent pathway
Trigeminal nerve (ophthalmic)
Trigeminal nerve (ophthalmic and maxillary)
Optic nerve
Optic nerve
Interneuron
Subcortical
Subcortical
Cortical, cerebellum
Subcortical
Efferent pathway
Facial nerve
Facial nerve
Facial nerve, VI, IX
Facial nerve
Effectors
Orbicularis oculi muscle
Orbicularis oculi muscle
Orbicularis oculi muscle, retractor bulbi muscle
Orbicularis oculi muscle
Response
Blink
Blink
Blink, retract globeb
Blink
Species
Blinks/min
Interblink period
Concurrent blinks (%)
Dog
3–5
20–30 sa
85
Cat
1–5 per 5 min
18.5 s
70
Horse
19
77
Cattle
5
60
Pigs
10
90
Eyelids in the Dog
Eyelids in the Cat
Other Species
Horse
Cattle, Sheep, and Pigs
Birds and Reptiles
Tear Production and Drainage
Cornea
Transparency
Metabolism
Biomechanics
Sensitivity and Innervation
Corneal layer
Elastic modulus (kPa)
Rabbit (Thomasy et al., 2014)
Human (Last et al., 2009, 2012)
Epithelium
0.6 ± 0.3
Not assessed
Anterior basement membrane
4.5 ± 1.2
7.5 ± 4.2
Bowman’s layer
Absent
110 ± 13
Stroma
1.1 ± 0.6 (anterior)
0.4 ± 0.2 (posterior)
33 ± 6 (anterior)
Descemet’s membrane
12 ± 7.4
50 ± 18
Endothelium
4.1 ± 1.7
Not assessed
Iris and Pupil
Stimulus
Illumination of the retina
Receptors
Photoreceptors (rods and cones)
Afferent pathway
Optic nerve–optic tract to pretectal area (ipsi‐ and contralateral via posterior commissure)
Efferent pathway
Pretectal area to the parasympathetic nucleus of CN III (ipsi‐ and contralateral), and then parasympathetic fibers to ciliary ganglion (via CN III)
Postganglionic fibers to the iris
Effector
Sphincter muscle of the iris
Response
Miosis (constriction of the pupil both direct and consensual reflex)
Species
Iris sphincter
Iris dilator
Ciliary muscles
Human
α and β equally
Mainly α, very few or no β
Mainly β, very few α
Rabbit
Mainly β, few α
Mainly α, few β
Mainly α, few β
Monkey
Mainly α, perhaps β
Mainly α, few β
Only β, no α
Cat
Mainly β, some α
Mainly α, some β
Mainly β, some α
Dog
α and β
α and β
?
Nutrition of Intraocular Tissues
Ocular Circulation
Ocular Blood Flow
Anterior Uveal Blood Flow
Choroidal Blood Flow
Retinal Blood Flow
of 75–80 mmHg, so as to increase flow from 15 to 50 ml/min. Neural control of retinal blood flow is limited to those vessels indirectly affecting retinal blood flow. Retinal vessels have α‐adrenergic binding sites that, when stimulated, cause vasoconstriction, thus increasing retinal vascular resistance. Retinal arteries most likely autoregulate through a myogenic mechanism, which is activated based on stretch. During sympathetic stimulation, myogenic autoregulatory responses appear to increase. Opening and closing of capillary beds in many tissues occur with varying metabolic needs. The vascular endothelial cells may produce nitric oxide, endothelins, prostaglandins, and renin–angiotensin products in response to chemical stimuli (e.g., acetylcholine and bradykinin), changes in blood pressure and blood vessel wall stress, changes in local oxygen levels, and other stimuli. As the mechanisms of local autoregulation become better understood, pharmacological modulation of these processes may become possible. The theoretical oxygen diffusion maximum of 143 μm plays a significant role in animal species with avascular retinas; as a result, avascular retinas are usually very thin, and have short photoreceptors, no tapeta, high glycogen levels in the Müller cells, and no retinal taper.
Blood Flow of the Optic Nerve Head
Ocular Barriers
Blood–Aqueous Barrier
Blood–Retinal Barrier
Aqueous Humor and Intraocular Pressure
Aqueous Humor Formation
Aqueous Humor Composition
Aqueous Humor Regulation
Aqueous Humor Outflow
Structural and Biomechanical Attributes
Dog
Cat
Rabbit
Cow
Horse
Nonhuman primate
Estimated normal IOP (mmHg)
15–18
17–19
15–20
20–30
17–28
13–15
“C” outflow (μl/mmHg/min by tonography)
0.24–0.30
0.27–0.32
0.22–0.28
—
0.90
0.24–0.28
Uveoscleral outflow (μl/min)
15%
3%
13%
—
—
30–65%
Episcleral venous pressure (mmHg)
10–12
8
9
—
—
10–11
Aqueous formation (μl/min)
5.22
6.00–7.00
1.84
—
—
2.75
Fluid Dynamics
I
F in = F at + F uf
F = flow (μl/min)
F in = total AH inflow
F at = inflow from active transport
F uf = inflow from ultrafiltration
II
F out = F trab + F uveo
F out = total AH outflow
F trab = outflow via the TM
F uveo = outflow via the uveoscleral pathway
III
C total = C trab + C uv + C pseudo
C = facility or conductance of flow (μl/min/mmHg)
C total = total AH outflow facility
C trab = facility of outflow via the TM
C uv = facility of outflow via the uveoscleral pathway
C pseudo = pseudofacility
III
At steady state, F = F in = F out
IV
F = C trab (P i − P e) (Goldmann equation)
P = pressure (mmHg)
P i = IOP
P e = episcleral venous pressure
V
F in = C trab(P i − P e) + F uveo
VI
P i = P e + (F in − F uveo)/C trab
Regulation of Outflow
Methods to Measure Aqueous Dynamics
I
Techniques to investigate the formation of aqueous humor
Cannulation of anterior chamber: constant rate/constant pressure perfusion
Direct view/measurement of newly formed aqueous humor
Use of markers in aqueous humor (radioactive, fluorescein, para‐aminohippuric acid). Measure the decay rate of intracamerally injected isotopes. Fluorophotometry, a noninvasive method, is primarily used today
II
Procedures to investigate the exit of aqueous humor
Ocular perfusion to lower IOP
Perilimbic suction cup
Tonography (conventional outflow/pressure sensitive)
Use of markers (fluorescein, nitrotetrazolium, latex spheres, radioactive tracers). Both conventional and uveoscleral outflow routes are measured
III
Methods to measure the episcleral venous pressure
Partial to complete collapse of the episcleral veins to affect alteration in the blood flow
Torsion balance
Pressure chamber (filled with air or saline)
Air jet
Ocular compression
Direct cannulation and measurement by transducer
Species
Anterior chamber (ml)
Posterior chamber (ml)
Lens volume (ml)
Vitreous volume (ml)
Human
0.2
0.06
0.2
3.9
Rabbit
0.3
0.06
0.2
1.5
Pig
0.3
—
—
3.0
Dog
0.8
0.2
0.5
3.2
Cat
0.8
0.3
0.3
2.8
Cow
1.7
1.5
2.2
20.9
Horse
2.4
1.6
3.1
28.2
Ocular Rigidity
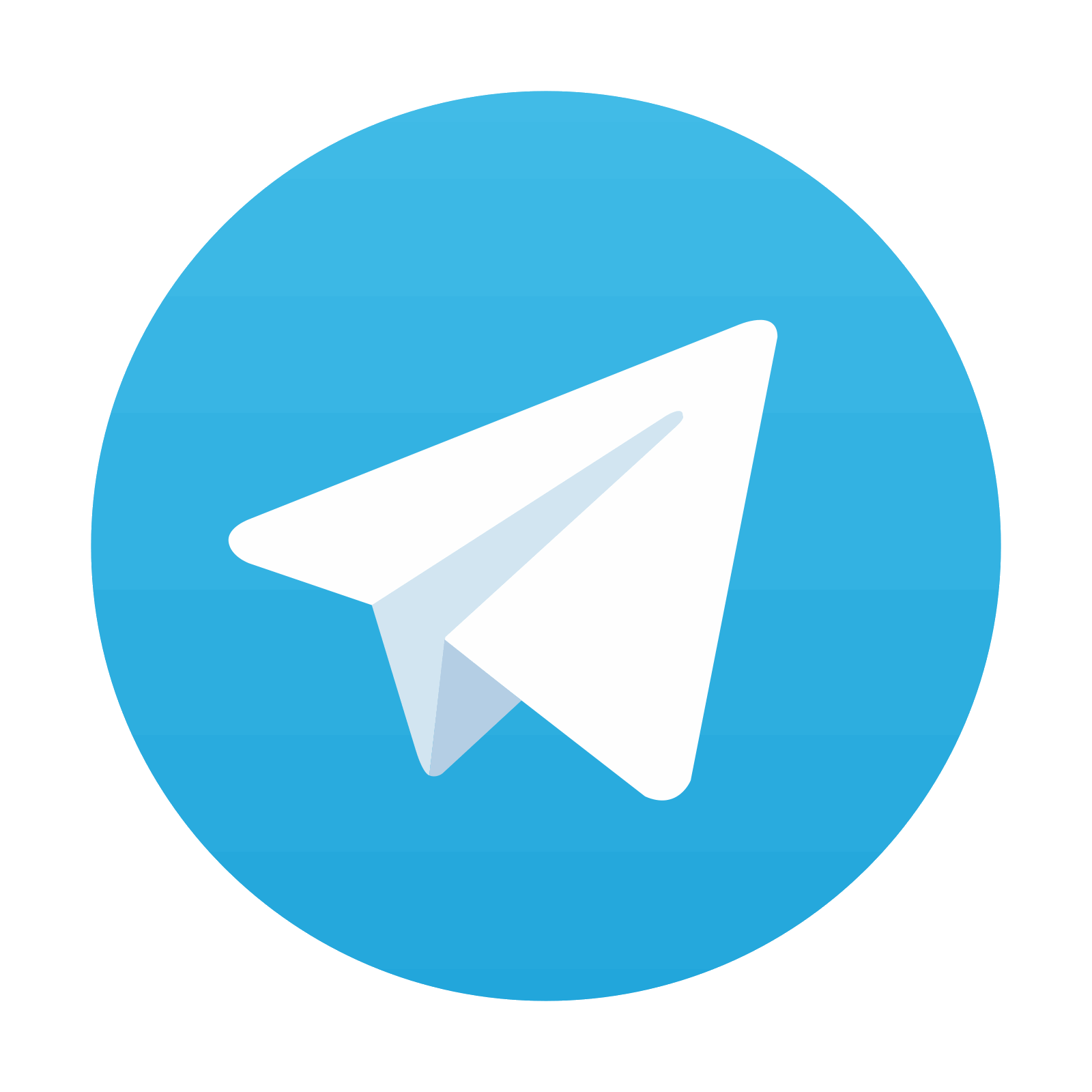
Stay updated, free articles. Join our Telegram channel
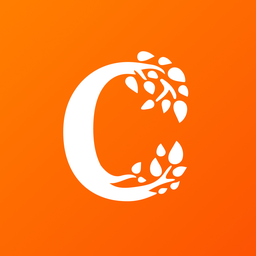
Full access? Get Clinical Tree
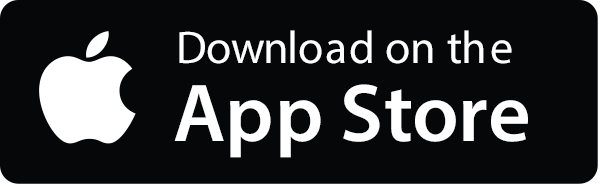
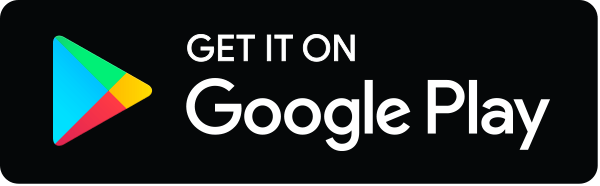