Molly Shepard1 and Benjamin Brainard2 1 MedVet Medical and Cancer Centers for Pets, 3305 N California Ave, Chicago, IL, 60618, USA 2 Department of Small Animal Medicine & Surgery, College of Veterinary Medicine, University of Georgia, Athens, GA, 30602, USA Aerobic metabolism, the bedrock physiologic process accounting for 90% of all cellular metabolism in mammals, represents an essential step in the maintenance of cell integrity and systemic organ function. For aerobic metabolism to proceed and efficiently produce the energy substrates vital for these biologic processes, a perpetual balance must be maintained between the delivery and consumption of O2 in tissues. The principal cardiovascular goal in the management of any anesthetic episode is the maintenance of oxygen delivery to tissues. Oxygen delivery (DO2) is the product of cardiac output (Q) and arterial oxygen content (CaO2). Oxygen content comprises both dissolved and hemoglobin (Hb)‐bound oxygen in the blood. The calculation of CaO2 considers the arterial partial pressure of oxygen (PaO2, in millimeters of mercury), the Hb concentration (in grams per deciliter of blood), and saturation of arterial Hb with oxygen (SaO2, percentage expressed as a decimal). This relationship is described by Eq. (14.1) [1]: Because approximately 97% of total CaO2 is bound to Hb in mammalian blood [2], the contribution of Hb concentration and SaO2 to CaO2 is much greater than that of PaO2. As a result, disorders in oxygen‐carrying capacity (e.g., anemia and dyshemoglobinemias) have a much greater impact on oxygen delivery to tissues compared to disorders of decreased dissolved oxygen stores, although low dissolved oxygen will adversely affect the Hb saturation (Table 14.1). Table 14.1 demonstrates the effects of anemia, hypoxemia, and dyshemoglobinemia (e.g., methemoglobinemia) on CaO2 and subsequent DO2. In general, mammalian CaO2 is equal to 19.9 ml O2 dl−1 blood under standard conditions (arterial partial pressure of CO2 of 40 mmHg, 37 °C body temperature, pH 7.4, and [Hb] of 15 g dl−1) [1]. While the severe hypoxemia (decreased PaO2) shown in Table 14.1 causes a relatively small decrease in overall CaO2 (17.2 ml O2 dl−1 blood), severe reductions in oxygen‐carrying capacity (anemia or methemoglobinemia) produce more profound reductions in CaO2. Changes in cardiac output (increases in heart rate, stroke volume, or both) can compensate for low CaO2 to a degree, but, at critical points, tissue oxygen delivery may be compromised. Table 14.1 Effects of anemia, hypoxemia, and methemoglobinemia on the oxygen content of arterial blood. A 50% reduction in hemoglobin concentration (e.g., severe anemia) causes a more profound decrease in oxygen delivery than a 50% reduction in dissolved oxygen (severe hypoxemia). Methemoglobinemia decreases the availability of normal hemoglobin, thereby decreasing oxygen delivery to tissues. Oxygen delivery in this table is calculated on the basis of a cardiac output of 2 l min−1. Arterial oxygen content may be monitored by blood‐gas analysis or may be estimated via oximetry and hematology tests, including packed cell volume (PCV), hematocrit (Hct), and Hb concentration. Blood‐gas analysis quantifies the partial pressure of oxygen dissolved in arterial or venous blood (PaO2, PvO2) and the saturation of Hb with oxygen (SaO2, SvO2). Patients breathing room air (approximately 21% O2) in the absence of pulmonary disease or other sources of poor gas exchange (e.g. low pulmonary perfusion), are expected to have a PaO2 between 80 and 100 mmHg, PvO2 of 42 ± 5 mmHg, SaO2 of 98–100%, and SvO2 of 75% [3]. Pulse oximetry provides a less invasive means of monitoring Hb–oxygen saturation within a specific peripheral capillary bed, yielding an “SpO2” value. The relationship between PaO2 and SaO2 (or SpO2) is described by the oxyhemoglobin dissociation curve (Figure 14.1). In general, as PaO2 increases, more oxygen is available to bind to Hb, to a maximum of 100% saturation. An SpO2 value of 100% correlates with PaO2 values ≥100 mmHg, whereas an SpO2 of 95% correlates with a PaO2 of approximately 80 mmHg. An SpO2 value of 94% correlates with a PaO2 of approximately 70 mmHg and is consistent with hypoxemia that merits further diagnostics and treatment. Various physiologic changes affect the affinity of Hb for oxygen and may result in a shift of the oxyhemoglobin curve to the right (decreased affinity, favoring offloading of oxygen to the tissues) or to the left (increased affinity, favoring onloading of oxygen to Hb). In humans, the conditions that decrease affinity of Hb for oxygen include increased partial pressure of CO2, increased body temperature, increased 2,3‐diphosphoglycerate (2,3‐DPG) concentration within the erythrocyte, and decreased blood pH. The conditions that increase affinity of Hb for oxygen include decreased blood CO2 concentration, decreased body temperature, decreased 2,3‐DPG, and increased pH (Figure 14.1). Hemoglobin structure also influences its affinity for oxygen [4]. Normal Hb must be in the reduced, ferrous (Fe2+) state to bind oxygen. Dyshemoglobinemias can be caused by the presence of methemoglobin (metHb) or carboxyhemoglobin (COHb). MetHb is an oxidation product of Hb that forms a reversible complex with oxygen and impairs offloading of oxygen to the tissues. There are congenital and acquired causes of methemoglobinemia, including toxicity from acetaminophen, nitrates, benzocaine, benzyl alcohol, and prilocaine. Feline Hb appears to be more sensitive to MetHb formation than canine Hb [5]. Carboxyhemoglobin (COHb) is formed when Hb is exposed to carbon monoxide. Carbon monoxide competitively binds to Hb with an affinity more than 200‐fold greater than Hb’s affinity for oxygen and is most commonly encountered in patients exposed to products of combustion (e.g., house fires) [6]. Figure 14.1 Oxyhemoglobin dissociation curve and regulating factors. Physiologic factors that shift the curve to the left, facilitating binding of oxygen to hemoglobin, include alkalemia (decreased circulating hydrogen ion concentration), decreased body temperature, decreased circulating partial pressure of CO2, and decreased concentration of 2,3‐diphosphoglycerate (2,3‐DPG). Factors that shift the curve to the right, facilitating offloading of oxygen to the tissues, are acidemia, increased 2,3‐DPG concentration, increased body temperature, and increased circulating partial pressure of CO2. Oxygen‐carrying capacity may be quantified by tests including Hct, PCV, red blood cell (RBC) count, and Hb concentration. Hct is the percentage of blood composed of RBCs. Centrifugal methods yield PCV, which accurately estimates Hct. Hct can be calculated by automated cell counters; however, because many hematology analyzers are designed for human blood and most domesticated mammalian species (except for dogs) have smaller erythrocytes than humans, this method of Hct calculation has more potential for error than PCV. Calculation of Hb concentration provides the most specific indicator of oxygen‐carrying capacity but may be falsely high in the presence of hemolysis, lipemia, Heinz bodies, or synthetic Hb products. When erythrocytes are normal in size and intravascular hemolysis is not significant, Hb concentrations should equal approximately one‐third of the Hct. For example, patients with a hemoglobin concentration of 10 g dl−1 of blood are expected to have a concurrent hematocrit of approximately 30%. Causes of anemia in companion animals can be generally classified into three categories, as follows (Table 14.2) [7]: (i) inadequate erythrocyte production (i.e., abnormal erythropoiesis), (ii) increased consumption or destruction (e.g., hemolysis), and (iii) loss (e.g., hemorrhage). Causes of decreased erythrocyte production include systemic disease (e.g., chronic kidney disease, neoplasia with myelophthisis), or toxicities (e.g., estrogen). Hemolysis may develop secondary to immune‐mediated mechanisms or toxicities, neoplasia, or infection with RBC parasites (e.g., Babesia spp.). Chronic hemorrhage in veterinary patients may frequently be subtle or subclinical, making signs such as melena or elevated blood urea nitrogen (BUN) (possible indicators of gastrointestinal bleeding) significant component of the preanesthetic workup. Hemorrhage may also be due to anticoagulant rodenticide toxicity, and vitamin K1 therapy may be indicated before the patient undergoes any invasive procedures (see the following section). In patients presenting for anesthesia and surgery who may have clinical signs of anemia due to any of the aforementioned causes, at minimum, a platelet count and coagulation panel are indicated to better define the patient’s hemostatic ability. It is also prudent to obtain a blood type on the patient before anesthesia, which may facilitate rapid transfusion during the procedure, if necessary. Table 14.2 Pathophysiology of anemia. Source: Adapted from: Brockus and Andreasen (2003). The chronicity of a patient’s anemia may influence the management of intraoperative hemorrhage. With chronic anemia, Hb gradually develops an increased relative ability to carry oxygen, due to an increase in 2,3‐DPG. This physiologic change may partially compensate for the anemia‐related oxygen delivery deficit [8]. Because of this physiologic adaptation, patients with chronic anemia may tolerate small intra‐anesthetic decreases in Hct without requiring RBC transfusion. It bears consideration, however, that an unpredictable degree of drug‐related vasodilation or splenic engorgement may occur during anesthesia, making even a small decrease in the circulating RBC mass a catastrophic event for animals with preexisting anemia. Adult patients with chronic hypoxemia also develop increased 2,3‐DPG levels over time, shifting their oxyhemoglobin dissociation curve to the right, which favors offloading of oxygen to their tissues [9]. Intravenous crystalloid therapy may further exacerbate this anemia, reducing blood viscosity, precipitating edema, and potentially impairing O2 delivery at the microvascular level. These special considerations for anemic patients thereby justify inclusion of monitoring which may suggest disturbances in tissue oxygen delivery (e.g., serial lactate quantification, mixed‐venous SO2 [as a surrogate of oxygen extraction], or tachycardia not attributable to hypercapnia, hypovolemia, chronotropic therapy, pain, or an inadequate plane of anesthesia). Hydration status should be considered while interpreting Hct values—anemia in a dehydrated or hypovolemic patient may only reveal itself after replacement of the fluid deficit. Serum total protein, albumin, BUN, and creatinine concentrations may also be elevated in dehydrated patients, although serum protein concentrations and decreases in body weight have proven more useful than azotemia or elevated Hct as indicators of dehydration in otherwise healthy dogs [10]. Healthy horses are likewise expected to lose body weight and have elevated blood protein concentration in the face of water deprivation, with no reliable change in PCV [11]. Interpretation of PCV may be further confounded in species known to undergo splenic contraction during times of stress (e.g., horses and dogs). Azotemia should always be interpreted with respect to urine‐specific gravity to differentiate renal, postrenal, and prerenal (dehydration or hypovolemia) causes. Hct should always be interpreted in conjunction with historical information, physical examination, and biochemistry findings in preparation for anesthetic management of individual patients. Caution should be exercised in the interpretation of PCV values in the midst of acute hemorrhage. Due to splenic contraction, acute hemorrhage is frequently accompanied only by a decreased total protein with a normal to increased PCV; changes in PCV may not be seen until hemorrhage is severe or adequate volume resuscitation has been administered, thus uncovering the degree of blood loss. Best practices in the emergent treatment of severe hemorrhage and hemorrhagic shock have evolved significantly, from a large‐volume crystalloid‐based approach to restricted and goal‐oriented fluid therapy, now integrating earlier transfusion of blood products. The understanding of the endothelial glycocalyx has revolutionized medical awareness of vascular fluid and protein dynamics and has shifted the focus of resuscitation efforts from the macrocirculation to one more inclusive of microcirculatory factors [12–14]. While traditional approaches to intraoperative hemorrhage in dogs may have consisted of boluses of isotonic crystalloid fluids in the amount of two or three times the volume of blood lost, investigations of hemorrhagic shock therapy in humans supports lower‐volume resuscitation (even using hypertonic crystalloid solutions [15, 16]) and earlier use of blood products, especially in cases of severe hemorrhage [17]. While natural and artificial colloids (e.g., albumin and hydroxyethyl starch (HES) solutions) can restore intravascular volume [18], the correlation in humans of artificial colloids with increased mortality risk, acute renal failure, and coagulopathy has deemphasized the use of hetastarches, especially in patients with sepsis or septic shock [19]. The significance of these concerns in veterinary patients is unknown [20–22]. With wider availability of canine albumin, however, this colloid may prove to be a useful adjunct to small‐volume resuscitation in canine patients when used as a 5% solution. An approach prioritizing the restoration of oxygen delivery to the microcirculation supports the earlier restoration of volume using blood products to replace shed RBCs, plasma, and platelets, ideally in a 1:1:1 ratio if there is massive hemorrhage, or at least earlier consideration of expanding the RBC mass in patients with intraoperative hemorrhage. Fresh or stored whole blood may be an optimal product to have on hand prior to surgeries where excessive hemorrhage is anticipated. Much of the data supporting a 1:1:1 ratio of blood products, with limited crystalloid, for resuscitation is derived from the human trauma literature. Human patients with traumatic shock experience impaired perfusion, resulting in acidosis, and a hyperfibrinolytic coagulopathic condition (“acute coagulopathy of trauma and shock”) caused by endothelial injury and hypothermia, all of which contribute to morbidity and mortality following shock [14]. In patients with ongoing hemorrhage following trauma, principles of low‐volume or damage‐control resuscitation advocate the maintenance of systemic hemodynamics while limiting additional hemorrhage until the source may be addressed. The techniques utilized to achieve these goals include permissive hypotension (systolic arterial pressure approximately 80–90 mmHg or mean arterial pressure approximately 60–65 mmHg), low‐volume fluid resuscitation, and titration of agents to minimize microvascular hypoperfusion (normalize systemic vascular resistance) until hemostasis is reestablished. Once hemorrhage is controlled, more aggressive fluid therapy may be considered to address systemic ischemia before irreversible endothelial injury occurs. The use of fresh or stored whole blood or a 1:1:1 ratio of platelets:plasma:RBCs is advocated for these patients, and is expected to improve outcome. Veterinary care centers face unique challenges to the implementation of low‐volume resuscitation, and its relevance for intraoperative hemorrhage, where the source may be rapidly controlled (as opposed to human patients that encounter trauma and hemorrhage outside of the hospital environment), is unclear. Blood product availability may be limited (particularly concentrated platelet products), and the cost of resuscitation using blood products may be cost‐prohibitive to pet owners. In the face of these challenges and current controversies regarding artificial colloids, the authors advocate consideration of the integration of natural colloids (fresh frozen plasma [FFP], albumin) and other blood products in the resuscitation of patients with hemorrhagic shock, in addition to careful consideration of the actual crystalloid fluid needs during procedures. Monitoring during anesthesia and resuscitation should include blood pressure, heart rate, and measures of perfusion and DO2 such as serial lactate quantification and urine output. The goal of RBC transfusions is to improve the oxygen‐carrying capacity of the blood and, thus, the oxygen delivery to tissues in anemic patients. RBCs may be transfused in the form of fresh or stored whole blood or in prepared solutions of packed red blood cells (pRBCs). With the use of appropriate anticoagulants and nutrients (e.g., as from RBC preservative solutions), pRBCs from dogs and cats may be stored refrigerated up to 35 days, although storage lesions may occur in older units. Storage lesions include a decrease in RBC deformability (due to adenosine triphosphate loss), a depletion of 2,3‐DPG, and a progressive acidosis within the unit. In humans, transfusion of RBC products stored for longer periods can result in limited improvement in tissue oxygen delivery and may be implicated in the development of transfusion‐related acute lung injury (TRALI) [23]. No reports of TRALI have been published in the veterinary literature to date, although there is some evidence that leukoreduction of pRBC units may decrease transfusion‐associated inflammation [24], and older RBC units may be more likely to cause transfusion reactions [25, 26]. The effects of different anticoagulant storage media have been described for canine, feline, and equine RBCs [27–29]. RBC transfusion is generally indicated for anesthetized or preanesthetic patients with Hb concentrations ≤5–7 g dl−1 (corresponding to a PCV of approximately 15–20%), especially if the decrease has been acute. Hb concentrations below this threshold can lead to significant decreases in oxygen delivery to tissues, and if hemorrhage occurs in these patients, the reduction in oxygen delivery may be precipitous. Hemorrhage constituting >20% of a patient’s blood volume, when accompanied by clinical signs indicating a sympathetic response to hypovolemia (e.g., tachycardia and hypotension), may also justify transfusion of RBC, in addition to volume stabilization with isotonic crystalloids and colloids. Approximate blood volumes of various species are listed in Table 14.3. Another indicator for transfusion in anemic patients may be hyperlactatemia (blood lactate > 2.0 mmol l−1), which suggests inadequate tissue oxygen delivery. Lactic acid is produced during anaerobic glycolysis and is indicative of a situation wherein the oxygen demand of the tissues exceeds the oxygen delivered [30]. Blood lactate concentration reflects the balance of lactate production by body tissues and the liver’s ability to metabolize it, which is rarely the limiting step (except for significant hepatic hypoperfusion). The blood lactate should be evaluated with respect to the patient, as it may be influenced by other factors including vessel selection (central versus peripheral venous sample), struggle during venipuncture of a peripheral vein, hypoxemia, or prolonged venous occlusion during venipuncture. Hyperlactatemia may support the decision to transfuse an anemic patient, in addition to hematology results and clinical signs indicating a sympathetic response to hypovolemia or hemorrhage (e.g., tachycardia, hypotension, and pale mucous membranes). Before transfusion of a patient with whole blood or pRBCs, the blood type of canine and feline patients should be determined. In general, type‐specific blood transfusions in small‐animal patients that have not received prior blood transfusions do not require cross‐matching. In emergent scenarios, dog erythrocyte antigen (DEA)‐1‐negative canine blood may be used in dogs without blood typing, but the possible presence of preexisting alloantibodies between feline blood types makes blood typing imperative before transfusion. In patients who have received prior transfusions or for whom more than 3 days have elapsed since a previous transfusion, cross‐matching is recommended to decrease the chance of immunologic reactions to blood transfusion. Procedures for blood collection and cross‐matching have been reviewed elsewhere [31]. Table 14.3 Approximate blood volumes for veterinary species. Blood product dosing is based on the volume of RBCs required to raise the recipient’s PCV to a desired value. An estimated volume for administration may be obtained using the following formula (Eq. (14.2)): It is generally assumed that the PCV of pRBC is 60–80%, while the donor PCV of whole blood will vary with the donor. Short‐hand calculations estimate a dose of 1 ml kg−1 of pRBC or 3 ml kg−1 of whole blood to raise the recipient PCV by 1%. A formula representing the total required volume of pRBC as 1.5 ml of pRBC × desired% PCV rise × kg body weight (BW) was also recently shown to be accurate [32]. Blood is typically supplied in aliquots of 500, 250, or 125 ml, and pRBCs are usually available in units of 250 or 125 ml. If sterile tubing welders are available, these bags may also be split into smaller volumes for administration to smaller patients. An opened (i.e., pierced with an IV administration set) bag of blood should be administered over a maximum of 4 h. Despite compatibility testing, any blood transfusion recipient may develop a reaction, although acute hemolytic reactions are uncommon in dogs [25, 33]. Identification of a transfusion reaction in an anesthetized patient can be challenging: urticaria may be hidden under surgical drapes, and changes in heart rate, blood pressure, or body temperature may be confounded by the effects of surgical stimuli, heat loss from body cavities, and anesthetic drug effects. Transfusions should be initiated at low rates; for example, 1–2 ml kg−1 h−1 for the first 15 min and then increased if no apparent reaction has developed. The duration of transfusion depends on the acuteness and severity of hemorrhage, although the transfusion should be complete by 4 h after initiation. Patients at greater risk for transfusion reaction may be administered intramuscular diphenhydramine, 2 mg kg−1 body weight. Transfusion technique significantly influences survival of RBCs in canine patients. Compared to administration by gravity flow, RBCs administered by volumetric or syringe pump may undergo more shear stress, which may cause lysis or decreased survival of the transfused cells [34]. In emergent scenarios, blood may be given rapidly either using a pressure infusion bag or by attaching an inline three‐way stopcock and 60‐ml syringe to pull blood from the unit and then rapidly infusing it into the patient through an inline filter. In scenarios such as these, with severe hemorrhage, the replacement of coagulation factors with FFP is frequently indicated, and the plasma may be used along with crystalloid and colloid fluids to maintain intravascular volume. Fresh or stored whole blood contains both RBC and plasma components. Sequelae of massive hemorrhage in anesthetized patients include dramatically reduced drug requirement (including inhalant anesthetics), cardiovascular instability, and development of metabolic acidosis, in addition to potential coagulopathy from accelerated hypothermia or the dilutional effects of fluid resuscitation and loss of coagulation factors. Patients administered large doses of blood products may also develop hypocalcemia (ionized calcium <1.2 mmol l−1) because of the citrate anticoagulant in blood products [35]. Hypocalcemia can precipitate cardiac arrhythmias and hypotension and exacerbate coagulopathy. Calcium may be supplemented intravenously as follows: 5–15 mg kg−1 of elemental calcium or 50–150 mg kg−1 of 10% calcium gluconate over a 10–20‐min period, while monitoring heart rate and rhythm via electrocardiogram. Calcium infusion should be slowed or discontinued if evidence of cardiotoxicity such as bradycardia, sudden elevation of the S‐T segment, or shortening of the Q‐T interval occurs. Due to the citrate anticoagulant in blood products, the calcium must be administered through a separate or well‐flushed catheter, and crystalloid fluids without calcium or magnesium should be used to flush the administration set of blood cells at the end of the transfusion. Anesthetic management of anemic patients should include preoxygenation, careful quantification of hemorrhage, minimization of hemodilution, and drug protocols that have a minimal influence on circulating RBC availability. Preoxygenation for patients with decreased oxygen‐carrying capacity will not greatly affect the proportion of Hb molecules bound with oxygen but will delay desaturation during anesthetic induction and may increase the oxygen transfer gradient at the level of the tissues (by increasing the amount of dissolved oxygen). In normal dogs premedicated with acepromazine and morphine and induced with propofol, 3 min of preoxygenation (100 ml kg−1 min−1 of 100% oxygen) significantly delayed Hb desaturation, compared to dogs allowed to breathe room air prior to induction [36]. Maintenance of oxygen delivery in the face of anemia depends on hemodynamic as well as metabolic compensatory mechanisms. Anemia‐related decreases in CaO2 stimulate a sympathetic response, which includes an increase in cardiac output (increased stroke volume or heart rate), as well as an increase in tissue oxygen extraction. These compensatory changes occur to fulfill the energy substrate and oxygen requirements of aerobic metabolism. The oxygen extraction ratio, normally about 25–30%, can increase to about 60–70% before oxygen consumption (VO2) is limited by oxygen delivery (Figure 14.2). Oxygen delivery in humans may remain normal in the face of Hb concentrations as low as 5 g dl−1 (caused by isovolemic hemodilution) due to compensatory increases in cardiac output and oxygen extraction [37]. When oxygen delivery decreases below the point of maximal tissue oxygen extraction (the critical DO2 point), tissue hypoxia ensues, and the tissues begin to use anaerobic metabolism for maintenance of cellular energy (Figure 14.2). Anaerobic metabolism can lead to a systemic lactic acidosis. Anesthetic agents that have minimal negative effects on cardiac output and vascular tone should be chosen for the patient with anemia, to preserve these intrinsic compensatory mechanisms as much as possible. Anesthesia can obtund the mechanisms of compensation for anemia. Humans subjected to normovolemic hemodilution while anesthetized with a fentanyl/nitrous oxide/isoflurane in oxygen combination had much smaller increases in stroke volume (and thus cardiac index) than awake patients undergoing the same hemodilution. These anesthetized humans did show an increased oxygen extraction to compensate for the decrease in DO2 [38]. From the perspective of anesthetic monitoring, hemodynamic responses to inappropriate hemodilution cannot be relied on for treatment decisions. Tachycardia, for example, may not occur in normovolemic patients as they approach their critical DO2 point. Anemic, anesthetized patients are at greater risk of reduced tissue oxygen delivery and may have reduced capacity to activate the sympathetic compensatory mechanisms seen in conscious individuals. The anesthetist must be vigilant in monitoring vital signs, blood loss, and even serial measures of DO2 indices, such as blood lactate concentration, to ensure adequate DO2. Figure 14.2 Relationship between oxygen delivery (DO2) and consumption (VO2). As flow (Q) or DO2 decreases, oxygen extraction increases, and VO2 remains stable. When DO2 decreases below the level requiring maximal tissue oxygen extraction (the critical DO2 point), VO2 concurrently decreases, resulting in tissue hypoxia. Source: Used with permission from Muir MM. (2007). Cardiovascular system. In: Tranquilli et al. editors, Lumb and Jones’ Veterinary Anesthesia and Analgesia, 4th edition, p. 109. Ames: Blackwell. Injectable and inhalant anesthetic agents may significantly decrease circulating erythrocyte mass by causing RBC sequestration in the spleen. The spleen serves as an important reservoir for RBCs, sequestering up to 40% of the RBC mass at any one time [39]. Isoflurane can decrease Hct by >30% in healthy ferrets due to splenic sequestration of RBCs [40]. Significant reductions in Hct can also occur after administration of various injectable agents; the following drugs, alone or in combination, have been shown to result in a 5–20% decrease in canine PCV: acepromazine, thiopental, propofol, and ketamine/diazepam. Combinations using thiopental and acepromazine produced greater reductions in PCV than ketamine/diazepam [41]. For this reason, acepromazine and thiopental are relatively contraindicated in anemic patients, and other drugs that may promote vasodilation and splenic engorgement (e.g., propofol) should be used sparingly. Injectable drugs known to cause significant hemolysis should be avoided in anemic patients. Formulations of etomidate and diazepam in propylene glycol may cause intravascular hemolysis due to the hyperosmolarity of the solution [42]. The administration of both of these drugs may be indicated in some compromised patients, given the relative cardiovascular stability associated with both drugs. Dilution of etomidate solution in 0.9% saline immediately before induction will decrease the osmolarity of the solution and may help to prevent hemolysis. Anesthetists concerned about hemolysis related to diazepam may select midazolam as an alternative, as it is water‐soluble and does not contain propylene glycol. Application of a balanced, multimodal approach will also reduce the doses of each drug, thereby minimizing negative side effects attributable to each individual agent. Abnormal Hct (high or low) can result in decreased oxygen delivery due to changes in blood viscosity and tissue blood flow. Hemoglobin concentration and blood viscosity for maximal relative oxygen delivery are 15 g dl−1 and approximately 2 cP, respectively. Blood viscosity affects DO2 by affecting the efficient movement of erythrocytes through capillaries. Decreases in blood viscosity can also lower DO2 beyond the effects of the anemia alone (Figure 14.3). The most important determinant of blood viscosity is Hct; patients with polycythemia or anemia are especially susceptible to decreased DO2. Polycythemia reduces RBC movement at the capillary level, creating a “sludging” effect, and prolongs capillary transit time. Inadequate blood viscosity, as seen in severely anemic patients, increases the likelihood of turbulent blood flow. This turbulence is recognized as physiologic cardiac murmurs in anemic patients. Figure 14.3 The effects of hemoglobin concentration and blood viscosity on relative oxygen delivery. Relative oxygen delivery is maximized at a hemoglobin concentration of 15 g dl−1 and blood viscosity of approximately 9 cP. From this point, decreases or increases in hemoglobin concentration and blood viscosity result in significant decreases in relative oxygen delivery. Source: Used with permission from: Muir MM. (2007). Cardiovascular system. In: Tranquilli et al. editors, Lumb and Jones’ Veterinary Anesthesia and Analgesia, 4th edition, p. 64. Ames: Blackwell. Polycythemia or erythrocytosis describes an increased RBC number. Some canine breeds (e.g., Sighthounds) may have higher erythrocyte counts than other breeds, which should be taken into consideration when interpreting Hct data [43]. Polycythemia is characterized as either absolute, denoting an increased total RBC mass, or relative polycythemia, denoting a normal RBC mass but increased Hct due to decreased plasma volume (i.e., hemoconcentration). Absolute polycythemia is further categorized into primary (polycythemia vera) and secondary polycythemia, which results from conditions including chronic hypoxia. Chronic hypoxia leading to increased release of erythropoietin and secondary proliferation of RBCs may occur as a physiologic response to high altitudes, intracardiac right‐to‐left shunting, or, less commonly, severe pulmonary disease. Relative polycythemia most commonly results from loss of body fluid and subsequent hemoconcentration. Clinical signs of polycythemia are attributable to hyperviscosity and decreased microcirculatory flow. Depending on the severity of the disease, patients may be at greater risk of tissue hypoxia and thrombosis. Hyperviscosity (caused by elevations of blood cells or plasma proteins) commonly manifests as neurologic abnormalities (seizures and collapse) secondary to compromised cerebral microcirculation.
14
Hematologic Disorders
Introduction
Patient status
[Hb] (g dl−1)
SaO2 (%)
PaO2 (mmHg)
CaO2 (ml O2 dl−1)
DO2 (ml min−1) (assuming cardiac output approximately 2 l min−1)
Normal
15
97.5
100
19.9
398
Hypoxemia
15
85
50
17.2
344
Anemia
7.5
97.5
100
10.1
202
Methemoglobinemia (33% MetHb)
10
97.5 (functional saturation)
100
13.3
266
Oxygen Content Monitoring in the Anesthetized Patient
Hemorrhage
Accelerated erythrocyte destruction
Abnormal erythropoiesis
Acute
Chronic
Intravascular hemolysis
Extravascular hemolysis
Reduced erythropoiesis
Defective erythropoiesis
GI ulcers
Hemostasis defects
Neoplasia
Thrombocytopenia
Trauma
Surgery
Disseminated intravascular coagulation (DIC)
Toxicities
Hemophilia A and B
Bacteria
RBC parasites
Chemicals and plants
Immune‐mediated
Hypoosmolality
Fragmentation
Hypophosphatemia
G6PD deficiency
Glutathione (GSH) deficiency (sheep)
Hepatic failure (horses)
Phosphofructokinase deficiency (dogs)
RBC parasites
Immune‐mediated
Intrinsic erythrocyte defects
Fragmentation
Hemophagocytic syndrome
Hypersplenism
Malignant histiocytosis
Anemia of chronic disorders
Cytotoxic bone marrow damage
Reduced erythropoietin
Immune‐mediated
Infection
Myelophthisis
Abnormal maturation
Disorders of heme synthesis
Disorders of nucleic acid synthesis
Red Blood Cell Transfusions
Species
Approximate blood volume (ml kg−1)
Canine
86
Feline
55
Equine
76
Bovine
55
Murine
79
Anesthetic Considerations for Patients with Anemia
Red Blood Cell Disorders and Blood Viscosity
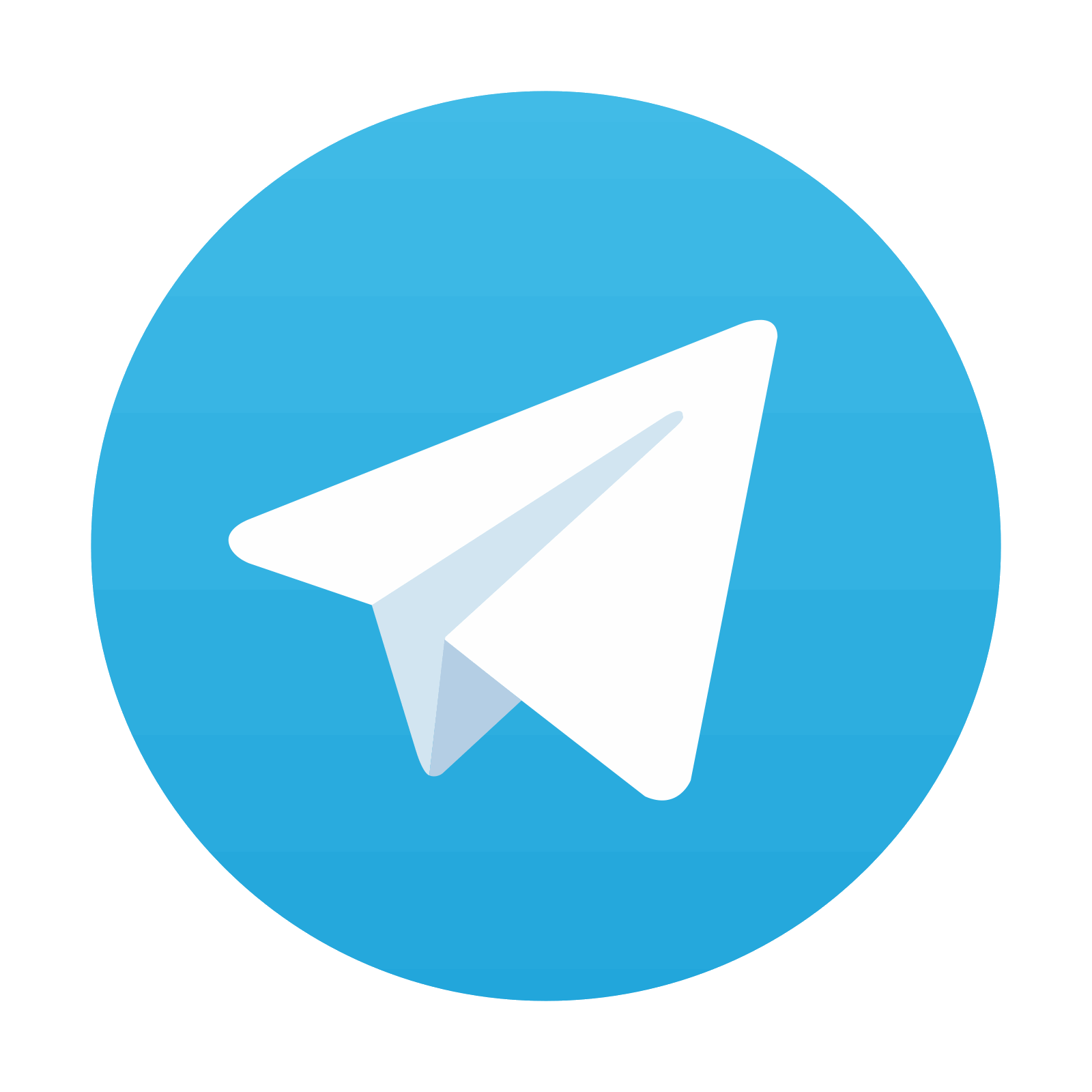
Stay updated, free articles. Join our Telegram channel
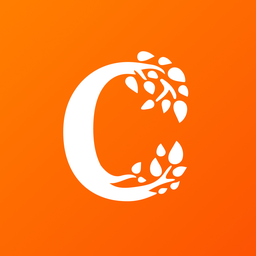
Full access? Get Clinical Tree
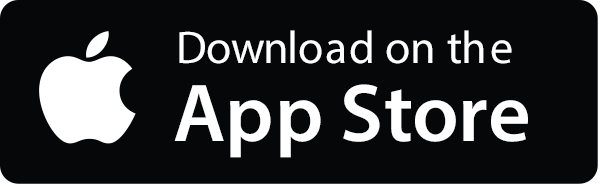
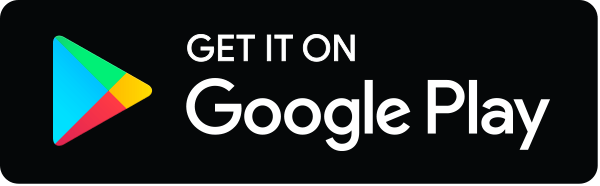