Genetic analyses among foals at an age between 4 and 9 months and in 2-year-old horses demonstrated genetic correlations between the signs for osteochondrotic lesions in foals and OCD (joint bodies at the predilection sites in horses aged 2 years) at rg = 0.84 for fetlock joints and rg = 0.99 for hock joints (Schober, 2003). The corresponding phenotypic correlations were rp = 0.66 (fetlock joints) and rp = 0.68 (hock joints), indicating a significant consistency of the radiological findings in the two different age classes of Hanoverian warmblood horses. Furthermore, genetic correlations among OC and OCD were rg = 0.80 in Dutch warmblood (van Grevenhof et al., 2009). Osseous fragments in front and hind fetlock joints represent genetically uniform traits as high additive genetic correlations between these traits were found (Stock et al., 2005b). For male horses, a higher heritability of osseous fragments in front fetlock joints was more pronounced than in fetlock joints of the hind limbs (Stock et al., 2005b). The genetic correlations between osseous fragments in fetlock and hock joints were slightly-to-moderately negative in Hanoverian warmblood (Stock et al., 2005b), and slightly positive in Dutch warmblood (van Grevenhof et al., 2009) horses.
Osseous fragments in fetlock joints showed moderately positive genetic correlation with deforming arthropathies in hock joints, whereas osseous fragments and deforming arthropathies in hock joints were slightly negatively correlated (Stock & Distl, 2006a). Pathologic changes of navicular bones were not genetically correlated with osseous fragments in fetlock or hock joints (Stock & Distl, 2006a).
Positive genetic correlations were found between height at withers and osseous fragments in fetlock and hock joints (Stock et al., 2005b). Regarding hock and fetlock joints, negative genetic correlations were found between height at withers and the incidence of hock OC (Philipsson et al., 1993, Willms et al., 1999). Large carpal circumference was genetically associated with a high incidence of hock OC (Philipsson et al., 1993).
A positive genetic correlation was estimated between show jumping and the occurrence of OCD in sport horses (Winter et al., 1996). A study in 3,725 Hanoverian warmblood horses analyzed genetic correlation between performance in sports and osseous fragments in fetlock and hock joints (Stock & Distl, 2006b). Performance was measured as the number of annual tournament entries (TE) and placings (TP) in basic buildup, dressage and show-jumping competitions. High prevalence of osseous fragments in hock joints were genetically negatively correlated with TP and TE in dressage competitions. A similar tendency was found for osseous fragments in hock and fetlock joints with TP in show-jumping competitions (Stock & Distl, 2006b). Mare performance tests and inspections at riding horse auctions showed mostly close to zero genetic correlation between osseous fragments in fetlock and hock joints (Stock & Distl, 2007).
Heritability report indicates that selection for skeletal soundness may be feasible. When weighting radiographic traits with 30–60% as opposed to the respective performance traits, and selecting only sires with above-average total indices, the prevalence of each radiographic finding can be lowered by up to 10% in the progeny. Considering only one radiographic finding at a time, the maximum attainable response to selection in a generation can be a decrease of prevalence of radiographic findings by 31–52% (Stock & Distl, 2005). Therefore, parameters of the health of the equine skeleton could be considered at this early stage of selection of breeding animals.
Quantitative trait loci (QTL) for OC
In recent years, there have been major advances in mapping the equine genome. Whole-genome radiation hybrid maps (Chowdhary et al., 2003; Raudsepp et al., 2008), comprehensive genetic linkage maps (Penedo et al., 2005; Swinburne et al., 2006), a medium-density horse gene map (Perrocheau et al., 2006), informative marker sets for QTL analyses (Mittman et al., 2010a), and a high-density microsatellite map (Mittman et al., 2010b) have been developed. The second release of the horse genome assembly became available in September 2007. EquCab2.0 is a high-quality draft sequence of the female Thoroughbred Twilight. A whole genome shotgun library was sequenced at the Broad Institute, Cambridge, Massachusetts, and BAC ends were sequenced at the University of Veterinary Medicine, Hannover and Helmholtz Centre for Infection Research, Braunschweig, Germany. A collection of more than 1 million single nucleotide polymorphisms (SNPs, one SNP per 2 kb) was generated mining the sequence from Twilight and additional sequences from seven horses of a variety of breeds, including Akhal-teke, Andalusian, Arabian, Icelandic, Quarter Horse, Standardbred, and Thoroughbred. Linkage disequilibrium is intermediate in length compared to dogs and humans, and dropping quickly down at 100–150 kb (Wade et al., 2009). With the horse genome assembly and SNP collection available, an equine beadchip comprising 54,602 SNPs has been developed and publicly released in 2009 (McCue et al., 2009).
Equine maps with a large number of highly polymorphic markers equidistantly distributed over the whole genome are prerequisites to perform linkage studies for identifying quantitative trait loci (QTL). QTL are genomic regions that are highly likely to harbor genes influencing the trait of interest. The larger the proportion of the phenotypic variation of the QTL, the more useful the QTL are for selection. QTL show linkage disequilibrium within families or in the whole population. An ideal marker for a QTL shows linkage disequilibrium in the whole population. After identification of QTL in population-wide linkage disequilibrium, marker-assisted selection provides the possibility to improve accuracy of selection in young horses and to shorten the generation interval in horse breeding.
Whole-genome scans for half-sib families with a high incidence of OC were employed to detect QTL significantly contributing to the phenotypic expression of OC. Data from 14 half-sib families of Hanoverian warmblood horses were analyzed using microsatellite markers to detect quantitative trait loci (QTL) with significant influence on the development of OC (Dierks et al., 2007). Genome-wide significant QTL for osteochondrosis in fetlock and hock joints were found on horse chromosomes (ECA) 2, 4, 5, and 16. Further, chromosome-wide significant QTL were located on ECA3, 15, 19, and 21. QTL for fetlock OC were on ECA2, 4, and 5. The locations of QTL for hock OC were on ECA2, 15, 16, and 21. To refine the identified QTL, an extended marker set containing published, as well as newly developed microsatellites and SNPs, was used. Fine-mapping confirmed and delimited QTL on ECA2 (15.65–31.91 Mb for fetlock OC and fetlock OCD; 26.89–33.05 for hock OC), ECA4 (7.42–13.10 Mb and 56.15–59.84 Mb for fetlock OC, 3.62–6.24 Mb for hock OC), ECA5 (78.03–90.23 Mb for fetlock OC and fetlock OCD), ECA16 (17.6–45.18 Mb for hock OCD), and ECA21 (5.45–17.14 Mb for hock OC and hock OCD) (Lampe, Dierks, & Distl, 2009a, 2009b). On ECA18, a new QTL for OC and hock OC at 74.94–82.25 Mb could be identified in Hanoverian warmblood horses (Lampe et al., 2009c). On ECA2, a SNP within the neurochondrin (NCDN) gene was significantly associated with OC traits. On ECA4, three significantly associated SNPs have been identified within the genome-wide significant OC-QTL region: two SNPs were located in intergenic regions and one SNP was in intron 2 of the HECT, C2, and WW domain containing E3 ubiquitin protein ligase 1 (HECW1) gene.
In 117 South German coldblood horses a whole genome scan for OC was carried out (Wittwer et al., 2007b). Chromosome-wide significant QTL were found on 10 horse chromosomes, including 7 QTL for fetlock OC and 1 QTL on ECA18 for hock OC and fetlock OC. On ECA4, a significant QTL for fetlock OCD was detected and within this QTL six candidate genes at 50–67 Mb were screened using 22 SNPs. A significantly associated SNP in the 3′-untranslated region of the acyloxyacyl hydrolase (AOAH) gene could be identified (Wittwer et al., 2008). All horses homozygous for this SNP were affected by fetlock OCD. The odds ratio of being affected for the homozygous genotype was 2.71–3.67 in comparison to the heterozygous and wildtype homozygous genotypes. On ECA18, the QTL for fetlock OCD and hock OC was refined to an interval of 35.5 to 47.1 Mb. Screening 18 candidate genes with 71 SNPs in this QTL revealed 2 significantly associated intronic SNPs in the xin actin-binding repeat containing 2 (XIRP2) gene (Wittwer, Hamann, & Distl, 2009). Homozygous and heterozygous horses were at a 1.3- to 2.4-fold higher risk for fetlock OC, fetlock OCD, and hock OC.
Gene expression analyses and candidate genes
Studies on the variation in gene expression of key chondrogenic genes and genes differentially expressed between normal and OC chondrocytes may also help identify candidate genes and their potential role in the pathogenesis of osteochondrosis and repair mechanisms in osteochondrotic lesions. Potential candidate genes may code for hormones, enzymes, metabolic factors, and/or their receptors involved in the process of cartilage maturation and differentiation during endochondral ossification. Differential expression studies between neonates and adults showed 7% of the 9,367 probe sets differentially expressed. Up-regulated genes primarily included ontologies related to extracellular matrix proteins, matrix modifying enzymes, skeletal development, and cell adhesion (Mienaltowski et al., 2008). Candidate genes could also be those involved in epiphyseal dysplasia such as collagen genes, cartilage oligomeric matrix protein genes, or diastrophic dysplasia sulfate transporter genes.
The endocrinological procedures of skeletal development and growth are controlled by hormones that are most likely to participate in enchondral ossification, such as insulin, thyroxine, growth hormone, parathyroid hormone, and calcitonin (Glade, 1986). Of the regulating proteins involved in enchondral ossification, the transforming growth factor ß (TGF-ß) plays an important role in growth cartilage metabolism, particulary in the control of chondrocyte differentiation and hypertrophy (Glade, 1986; Henson, Schofield, & Jeffcott, 1997; Jeffcott & Henson, 1998). Henson et al. (1997) described a reduced expression and immunoreactivity in focal lesions compared to normal cartilage, but strong expression of TGFß1 in the chondrocyte clusters immediately surrounding the lesion, and therefore a possible involvement in OC. Semevolos, Nixon, and Brower-Toland (2001) found a higher expression of TGF-ß1 in osteochondrosis cartilage and suggested that this might explain a healing response to the OC lesion. Hypertrophic differentiation and enchondral ossification of growth cartilage are regulated by a complex array of signaling peptides, including parathyroid hormone related protein (PTHrP), Indian hedgehog (Ihh), TGFß, runt-related transcription factor 2 (Runx2), and bone morphogenetic proteins (BMPs). PTHrP maintains chondrocytes in a proliferative state by limiting the number of cells capable of expressing Ihh, and thus participates in a negative feedback loop controlling the rate of hypertrophic differentiation (Chung, Lanske, & Lee, 1998). The main regulator of chondrocyte hypertrophic differentiation is the transcription factor Runx2. PTHrP inhibits Runx2 expression, whereas Ihh expression is stimulated by Runx2. Further repressors of Runx2 activity are Sox9 and the histon deacetylase-4 (HDAC4). Nkx3/Bpax1 mediates the actions of PTHrP and inhibits Runx2 expression (Provot et al., 2006). A balance between the expression level of the transcription factor myocyte enhancer factor-2 (MEF2C) and repression by HDAC4 seems necessary for normal progression of chondrocyte hypertrophy (Arnold et al., 2007). Hedgehog signaling occurs through the transmembrane receptor, Patched (Ptc), which upon binding with Ihh releases its inhibitor, a transmembrane receptor, Smoothened (Smo). In turn, Smo activation results in stimulation of transcription factors, Gli1, Gli2, and Gli3. While a significant increase of PTHrP and Ihh expression in chondrocytes from OC-affected cartilage and a decrease of Gli1 expression could be observed, no difference in expression patterns were identified for BMP, Gli2, Gli3, Ptc, and Smo (Semevolos et al., 2002; Semevolos, Nixon, & Strassheim, 2004; Semevolos et al., 2005). RUNX2 showed the greatest differences of gene expression between lesions and normal cartilage (Mirams et al., 2008).
Insulin like growth factors (IGFs) play an important role in cartilage metabolism and growth, including the introduction of increasing cellular proliferation and the synthesis of cartilage aggrecan and collagen (Semevolos et al., 2001). An interdependency of OC in hock joints and plasma IGF-I levels has been observed (Sloet van Oldruitenborgh-Oosterbaan, Mol, & Barneveld, 1999). Foals with osteochondrotic findings showed significantly lower IGF-I levels than unaffected foals. Reduction in chondrocyte differentiation caused by lower plasma IGF-I concentrations may contribute to the development of osteochondrosis. Such an endocrine pathway to OC may be related with faster growth and earlier physical maturation in horses fed with high-energy diets and high genetic growth capacity. The significantly higher expression of IGF-I in cartilage obtained from osteochondrotic lesions in 8- to 12-month-old horses was correlated with the proliferation of cells repairing the cartilage damage, and therefore reflects a healing response to injured tissue rather than a primary alteration (Semevolos et al., 2001; Verwilghen et al., 2009).
The composition of the extracellular matrix has been targeted as another molecular mechanism involved in the development of OC. Various collagen types that are represented in the extracellular cartilage matrix are known to play a role in the development and maturation of cartilage. It is well known that the extracellular matrix of the articular cartilage goes through a phase of rapid remodelling in the neonatal animal (Van Weeren, 2005). Additional evidence for the crucial role of collagen was provided by the demonstration of differences in post-translational modifications of collagen type II in samples from early osteochondrotic lesions (Van de Lest et al., 2004). The expression of collagen type I, II and X in chondrocytes from OC cartilage was significantly higher than in normal cartilage (Garvican et al., 2008). These results could partly be confirmed by Mirams et al. (2008) who found a significantly higher expression of collagen type I and X in the osteochondrosis lesions, but no differences in the expression patterns of collagen type II. Also, Semevolos et al. (2001) could not find any significant differences in the expression of various collagen types I, II, and X between OC and normal joints. Cartilage-specific matrix genes (COL2A1, COL3A1, COL11A1, COL1A2) are abundantly expressed in normal articular cartilage (Gläser et al., 2009). Increased COL2A1, SOX9, and AGGRECAN expression was found in human articular chondrocytes during chondrogenesis (Miyaki et al., 2009). In osteoarthritic cartilage, COL2A1 and SOX9 expression was down-regulated, while cartilage-degrading enzymes (ADAMTS5 and MMP-13) were up-regulated.
Peansukmanee et al. (2009) found a reduced glucose transporter 1 (GLUT1) expression in either OCD or osteoarthritis (OA) cartilage. The equine GLUT1 gene is located on ECA2 at 15.56 to 15.57 Mb and consequently close to a detected QTL for OC in fetlock joints of Hanoverian warmblood horses (15.65 to 30.94 Mb). Peansukmanee et al. (2009) analyzed effects of hypoxia on glucose transport in equine chondrocytes and compared expression of the hypoxia responsive GLUT1 gene in either OCD- or OA-affected and in normal cartilage. They suggested that reduced GLUT1 might contribute to degenerative cartilage defects.
Metalloproteinases are considered to be a key feature in the loss of articular cartilage seen in many joint diseases. Different studies on the expression of matrix metalloproteinases MMP-1, MMP-3, and MMP-13 came up with the same results showing there was no significant difference in the expression of either MMP-1 or MMP-3, but a significant up-regulation of MMP-13 in OC-chondrocytes (Garvican et al., 2008, Mirams et al., 2008). Brama et al. (2000) investigated the role of MMP-3 activity in synovial fluid in common joint disorders in the horse and concluded that MMP-3 activity in OC joints appears not to be different from normal joints, but was four times higher in osteoarthritis joints. The ADAM metallopeptidase with thrombospondin type 1 motif 4 (ADAMTS4) gene encodes an enzyme responsible for the degradation of aggrecan, a major proteoglycan of cartilage. Aggrecan degradation is an important factor in the erosion of articular cartilage in arthritic diseases, which is also reflected in a significantly higher expression of ADAMTS4 in OC cartilage than in chondrocytes from normal cartilage (Garvican et al., 2008). However, aggrecan itself was not differently expressed among cartilage from OC lesions and normal cartilage (Semevolos et al., 2001; Garvican et al., 2008; Mirams et al., 2008). The proteins encoded by the tissue inhibitor of metalloproteinase (TIMP) gene family are natural inhibitors of the matrix metalloproteinases (MMPs). While TIMP-1 showed a significant increase of expression in chondrocytes from OC cartilage in comparison to normal cartilage, the expression of TIMP-2 and TIMP-3 in OC chondrocytes was significantly less (Garvican et al., 2008).
Navicular Disease
Navicular disease is one of the main causes of chronic, often therapy-resistant forelimb lameness in middle-aged horses. Pathological alterations can primarily affect the navicular bone (os sesamoideum distale), the navicular bursa (bursa podotrochlearis), or the distal end of the deep digital flexor tendon. Radiographic findings have been associated with navicular bone pathology such as branched or lollipop-shaped canales sesamoidales, an irregular, sclerosed or radiolucent structure, and a contour with exostoses (Brunken, 1986; Hertsch & Steffen, 1986; Kaser-Hotz & Ueltschi, 1992). Several evaluation schemes have been developed for radiographs of the navicular bone. Dik and van den Broek (1995) classified navicular bones on the basis of the shape of the proximal border, whereas Lukas (1987) considered both shape of the proximal border and of the medial and lateral extremities of the navicular bones. The evaluation scheme of Brunken (1986) permits a classification of radiographic findings in the navicular bone according to size, shape, and distribution of canales sesamoidales and the structure and contour of the navicular bone. Riding horses show very often (up to 70–80%) slight or moderate changes in the navicular bone, whereas moderate to severe changes are at low frequencies of 2–5%. Heritabilities for radiological changes of different severity in the navicular bone were at h2 = 0.10 to 0.34 (KWPN, 1994; Willms et al., 1999; Stock & Distl, 2006b). Chromosome-wide significant QTL were located on five different equine chromosomes (ECA2, 3, 4, 10, and 26). Genome-wide significant QTL on ECA2 and 10 (Diesterbeck, Hertsch, & Distl, 2007) were confirmed by fine-mapping revealing associated haplotypes and marker alleles. These studies represent the first step to get more insight into the molecular genetic determination of radiological alterations in the equine navicular bone.
Conclusions
Osteochondrosis and navicular disease are among the most common skeletal diseases in horses. The aetiology of these conditions is still not fully understood, but more research has focused on the molecular mechanisms involved. The substantial progress of comparative genomics and the horse genome sequencing project provide a very effective approach to unravelling the genetic basis of OC and navicular disease. The genomic regions containing QTL for OC, as identified by whole genome scans and genome-wide association studies, provide good chances for the detection of causal genes.
References
Arnold, M. A., Kim, Y., Czubryt, M. P., Phan, D., McAnally, J. et al. (2007). MEF2C transcription factor controls chondrocyte hypertrophy and bone development. Developmental Cell, 12, 377–389.
Barneveld, A., & Van Weeren, P. R. (1999). Conclusions regarding the influence of exercise on the development of the equine musculoskeletal system with special refernce to osteochondrosis. Equine Veterinary Journal, 31(Suppl.), 112–119.
Brama, P. A. J., TeKoppele, J. M., Van El, B., Barneveld, A., & Van Weeren, P. R. (2000). Influence of development and joint pathology on stromelysin enzyme activity in equine synovial fluid. Annuals of the Rheumatic Diseases, 59, 155–157.
Brunken, E. (1986). Röntgenologische Verlaufsuntersuchungen am Strahlbein des Pferdes. Master’s thesis, University of Veterinary Medicine Hannover.
Chung, U. I., Lanske, B., & Lee, K. (1998). Parathyroid hormone/parathroid hormone-related peptide receptor coordinates endochondral bone development by directly controlling chondrocyte differentiation. Proceedings of the National Academy of Sciences of the United States of America, 95, 13030–13035.
Chowdhary, B. P., Raudsepp, T., Kata, S. R., Goh, G., Millon, L. V. et al. (2003). The first-generation whole-genome radiation hybrid map in the horse identifies conserved segments in human and mouse genomes. Genome Research, 13, 742–751.
Dierks, C., Löhring, K., Lampe, V., Wittwer, C., Drögemüller, C., & Distl, O. (2007). Genome-wide search for markers associated with osteochondrosis in Hanoverian warmblood horses. Mammalian Genome, 18, 739–747.
Diesterbeck, U., Hertsch, B., & Distl, O. (2007). Genome-wide search for microsatellite markers associated with radiological alterations in the navicular bone of Hanoverian warmblood horses. Mammalian Genome, 18, 373–381.
Dik, K. J., & Van den Broek, J. (1995). Role of navicular bone shape in the pathogenesis of navicular disease: A radiological study. Equine Veterinary Journal, 27, 390–393.
Garvican, E. R., Vaughan-Thomas, A., Redmond, C., & Clegg, P. D. (2008). Chondrocytes harvested from osteochondrosis dissecans cartilage are able to undergo limited in vitro chondrogenesis despite having pertubations of cell phenotype in vivo. Journal of Orthopaedic Research, 26, 1133–1140.
Glade, M. J. (1986). The control of cartilage growth in osteochondrosis: A review. Journal of Equine Veterinary Science, 6, 175–192.
Gläser, K. E., Sun, Q., Wells, M. T., & Nixon, A. J. (2009). Development of a novel equine whole transcript oligonucleotide genechip microarray and its use in gene expresson profiling of normal articular-epiphyseal cartilage. Equine Veterinary Journal, 41, 663–670.
Grøndahl, A. M., & Dolvik, N. I. (1993). Heritability estimations of osteochondrosis in the tibiotarsal joint and of bony fragments in the palmar/plantar portion of the metacarpo- and metatarsophalangeal joints of horses. Journal of the American Veterinary Medical Association, 203, 101–104.
Henson, F. M., Schofield, P. N., & Jeffcott, L. B. (1997). Expression of transforming growth factor-beta 1 in normal and dyschondroplastic articular growth cartilage of the young horse. Equine Veterinary Journal, 6, 434–439.
Hertsch, B., & Steffen, D. (1986). Röntgenologische und vergleichen patho-morphologische Untersuchungen an Strahlbeinen unter besonderer Berücksichtigung der Canales sesamoidales – Ein Beitrag zur Diagnose der Podotrochlose. Deutsche Tierärztliche Wochenschrift, 93, 353–359.
Jeffcott, L. B. (1996). Osteochondrosis – an international problem for the horse industry. Journal of Equine Veterinary Science, 16, 32–37.
Jeffcott, L. B., & Henson, F. M. D. (1998). Studies on growth cartilage in the horse and their application to aetiopathogenesis of dyschondroplasia (osteochondrosis). The Veterinary Journal, 156, 177–192.
Kaser-Hotz, B., & Ueltschi, G. (1992). Radiographic appearence of the navicular bone in sound horses. Veterinary Radiology and Ultrasound, 33, 9–17.
KWPN (1994). The frequency and heredity of navicular disease, sesamoidosis, fetlock joint arthrosis, bone spavin, osteochondrosis of the hock. A radiographic progeny study. KWPN (Koninklijke Vereniging Warmbloed Paardenstamboek Nederland), Zeist.
Lampe, V., Dierks, C., & Distl, O. (2009a). Refinement of a quantitative trait locus on equine chromosome 5 responsible for fetlock osteochondrosis in Hanoverian warmblood horses. Animal Genetics, 40, 553–555.
Lampe, V., Dierks, C., & Distl, O. (2009b). Refinement of a quantitative gene locus on equine chromosome 16 responsible for osteochondrosis in Hanoverian warmblood horses. Animal, 3, 1224–1231.
Lampe, V., Dierks, C., Komm, K., & Distl, O. (2009c). Identification of a new quantitative trait locus on equine chromosome 18 responsible for osteochondrosis in Hanoverian warmblood horses. Journal of Animal Science, 87, 3477–3481.
Lukas, B. 1987. Radiographic demonstration of the development of the navicular bone (distal sesamoid bone) from birth to three years of age, with special reference to the sesamoid canals, in horses. Hanover: Tierarztliche Hochschule.
McCue, M., Mickelson, J., Bannasch, D., Penedo, C., Bailey, E. et al. (2009). The horse gentrain project: Initial evaluation of the Equine SNP50 BeadCips. Paper presented at The 8th Dorothy Russell Havemeyer Foundation International Equine Genome Mapping Workshop, July 22–25, Suffolk, UK.
Mienaltowski, M. J., Huang, L., Stromberg, A. J., & MacLeod, J. N. (2008). Differential gene expression associated with postnatal equine articular maturation. BMC Musculosceletal Disorders, 9, 149.
Mirams, M., Tatarczuch, L., Ahmed, Y. A., Pagel, C. N., Jeffcott, L. B. et al. (2008). Altered gene expression in early osteochondrosis lesions. Journal of Orthopaedic Research, 27, 452–457.
Mittmann, E. H., Lampe, V., Momke, S., Zeitz, A., & Distl, O. (2010a). Characterization of a minimal microsatellite set for whole genome scans informative in warmblood and coldblood horse breeds. Journal of Heredity, 101: 246–250.
Mittmann, E. H., Momke, S., & Distl, O. (2010b). Whole-genome scan identifies quantitative trait loci for chronic pastern dermatitis in German draft horses. Mammalian Genome, 21: 95–103.
Miyaki, S., Nakasa, T., Otsuki, S., Grogan, S. P., Higashiyama, P. et al. (2009). MicroRNA-140 is expressed in differentiated human articular chondrocytes and modulates interleukin-1 responses. Arthritis and Rheumatism, 60, 2723–2730.
Peansukmanee, S., Vaughan-Thomas, A., Carter, S. D., Clegg, P. D., Taylor, S. et al. (2009). Effects of hypoxia on glucose transport in primary equine chondrocytes in vitro and evidence of reduced GLUT1 gene expression in pathologic cartilage in vivo. Journal of Orthopaedic Research, 27, 529–535.
Penedo, M. C. T., Millon, L. V., Bernoco, D., Bailey, E., Binns, M. et al. (2005). International equine gene mapping workshop report: a comprehensive linkage map constructed with data from new markers and by merging four mapping resources. Cytogenetic and Genome Research, 111, 5–15.
Perrocheau, M., Boutreux, V., Chadi, S., Mata, X., Decaunes, P. et al. (2006). Construction of a medium-density horse gene map. Animal Genetics, 37, 145–155.
Philipsson, J., Andreasson, E., Sandgren, B., Dalin, G., & Carlsten, J. (1993). Osteochondrosis in the tarsocrural joint and osteochondral fragments in the fetlock joints in Standardbred trotters. II. Heritybility. Equine Veterinary Journal, 16(Suppl.), 38–41.
Pieramati, C., Pepe, M., Silvestrelli, M., & Bolla, A. (2003). Heritability estimation of osteochondrosis dissecans in Maremmano horses. Livestock Production Science, 79, 249–255.
Provot, S., Kempf, H., Murtaugh, L. C., Chung, U. I., Chyung, J. et al. (2006). Nkx3.2/Bapx1 acts as a negative regulator of chondrocyte maturation. Development, 133, 651–662.
Raudsepp, T., Gustafson-Seabury, A., Durkin, K., Wagner, M. L., Goh, G. et al. (2008). A 4103 marker integrated physical and comparative map of the horse genome. Cytogenetic Genome Research, 122, 28–36.
Sandgren, B., Dalin, G., Carlsten, J., & Lundeheim, N. (1993). Development of osteochondrosis in the tarsocrural joint and osteochondral fragments in the fetlock joints of Standardbred trotters. II. Body measurements and clinical findings. Equine Veterinary Journal, 16(Suppl.), 48–53.
Schober, M. (2003). Schätzung von genetischen Effekten beim Auftreten von Osteochondrosis dissecans beim Warmblutpferd. Dissertation, Georg-August-Universität-Göttingen.
Schougaard, H., Ronne, J. F., & Philipsson, P. (1990). A radiographic survey of tibiotarsal osteochondrosis in a selected population of trotting horses in Denmark and its possible genetic significance. Equine Veterinary Journal, 22, 288–289.
Semevolos, S. A., Nixon, A. J., & Brower-Toland, B. D. (2001). Changes in molecular expression of aggrecan and collagen types I, II and X, insulin-like growth factor-I, and transforming growth factor-beta 1 in articular cartlage obtained from horses with naturally acquired Osteochondrosis. American Journal of Veterinary Research, 7, 1088–1094.
Semevolos, S. A., Brower-Toland, B. D., Bent, S. J., & Noxon, A. J. (2002). Parathyroid hormone-related peptide and Indian hedgehog expression patterns in naturally acquired equine osteochondrosis. Journal of Orthopaedic Research, 20, 1290–1297.
Semevolos, S. A., Nixon, A. J., & Strassheim, M. L. (2004). Expression of bone morphogenetic protein-6 and -2 and bone morphogenetic antagonist in horses with naturally acquired osteochondrosis. American Journal of Veterinary Research, 1, 110–115.
Semevolos, S. A., Strassheim, M. L. Haupt, J. L., & Nixon, A. J. (2005). Expression patterns of hedgehog signaling peptides in naturally acquired equine osteochondrosis. Journal of Orthopaedic Research, 23, 1152–1159.
Sloet van Oldruitenborgh-Osterbaan, M. M., Mol, J. A., & Barneveld, A. (1999). Hormones, growth factors and other plasma variables in relation to osteochondrosis. Equine Veterinary Journal, 31(Suppl.), 45–54.
Stock, K. F., & Distl, O. (2005). Prediction of breeding values for osseus fragments in fetlock and hock joints, deforming arthropathy in hock joints and pathologic changes in the nacivular bones of Hanoverian Warmblood horses. Livestock Production Science, 92, 77–94.
Stock, K. F., & Distl, O. (2006a). Genetic correlations between osseus fragments in fetlock and hock joints, deforming arthropathy in hock joints and pathologic changes in the navicular bones of Warmblood riding horses. Livestock Science, 105, 35–43.
Stock, K. F., & Distl, O. (2006b). Correlations between sport performance and different radiographic findings in the limbs of Hanoverian Warmblood horses. Animal Science, 82, 83–93.
Stock, K. F., & Distl, O. (2007). Genetic correlations between performance traits and radiographic findings in the limbs of German Warmblood riding horses. Journal of Animal Science, 85, 31–41.
Stock K. F., Hamann, H., & Distl, O. (2005a). Prevalence of osseous fragments in limb joints of Hanoverian Warmblood horses. Journal of Veterinary Medicine, 52, 388–394.
Stock, K. F., Hamann, H., & Distl, O. (2005b). Estimation of genetic parameters for the prevalence of osseous fragments in limb joints of Hanoverian Warmblood horses. Journal of Animal Breeding and Genetics, 122, 271–280.
Stock, K. F., Hamann, H., & Distl, O. (2006). Factors associated with the prevalence of osseous fragments in the limb joints of Hanoverian Warmblood horses. The Veterinary Journal, 171, 147–156.
Swinburne, J. E., Boursnell, M., Hill, G., Pettitt, L., Allen, T. et al. (2006). Single linkage group per chromosome genetic linkage map for the horse, based on two three-generation, full-sibling, crossbred horse reference families. Genomics, 87, 1–29.
Van de Lest, C. H., Brama, P. A., Van El, B., DeGroot, J., Van Weeren, P. R. (2004). Extracellular matrix changes in early osteochondrotic defects in foals: A key role for collagen? Biochimica et biophysica Acta, 1690: 54–62.
Van der Harst, M. R., Van de Lest, C. H. A., DeGroot, J., Kiers, G. H., Brama, P. A. J., & Van Weeren, P. R. (2005). Study of cartilage and bone layers of the bearing surface of the equine metacarpophalangeal joint relative to different timescales of maturation. Equine Veterinary Journal, 37, 200–206.
Van Grevenhof, E. M., Schurink, A., Ducro, B. J., van Weeren, P. R., van Tartwijk, J. M. F. M. et al. (2009). Genetic variables of various manifestations of osteochondrosis and their correlations between and within joints in Dutch warmblood horses. Journal of Animal Science, 87, 1906–1912.
Van Weeren, P. R. (2005). Osteochondrosis: A challenging enigma. Pferdeheilkunde, 21, 285–292.
Van Weeren, P. R., & Barneveld, A. (1999). The effect of exercise on the distribution and manifestation of osteochondrotic lesions in the Warmblood foal. Equine Veterinary Journal, 31(Suppl.), 16–25.
Van Weeren, P. R., Sloet van Oldruitenborgh-Oosterbaan, M. M., & Barneveld, A. (1999). The influence of birth weight, rate of weight gain and final achieved height and sex on the development of osteochondrotic lesions in a population of genetically predisposed Warmblood foals. Equine Veterinary Journal, 31(Suppl.), 26–30.
Verwilghen, D. R., Vanderheyden, L., Franck, T., Busoni, V., Enzerink, E. et al. (2009). Variations of plasmatic concentrations of Insulin-like growth factor-I in post-pubescent horses affected with developmental osteochondral lesions. Veterinary Research Communications, 33, 701–709.
Wade, C., Giulotto, E., Sigurdsson, S., Zoli, M., Gnerre, S. et al. (2009). Genome sequence, comparative analysis, and population genetics of the domestic horse. Science, 326, 865–867.
Wilke, A. (2003). Der Einfluss von Haltung und Aufzucht auf die Häufigkeit von Osteochondrose. Dissertation, Tierärztliche Hochschule Hannover.
Willms, F., Röhe, R., & Kalm, E. (1999). Genetische Analyse von Merkmalskomplexen in der Reitpferdezucht unter Berücksichtigung von Gliedmaßenveränderungen. Züchtungskunde, 71, 330–345.
Winter, D., Bruns, E., Glodek, P., & Hertsch, B. (1996). Genetische Disposition von Gliedmaßenerkrankungen beim Reitpferd. Züchtungskunde, 68, 92–108.
Wittwer, C., Hamann, H., Rosenberger, E., & Distl, O. (2006). Prevalence of osteochondrosis in the limb joints of South German Coldblood horses. Journal of Veterinary Medicine, 53, 531–539.
Wittwer, C., Hamann, H., Rosenberger, E., & Distl, O. (2007a). Estimation of genetic parameters for the prevalence of osteochondrosis in the limb joints of South German Coldblood horses. Journal of Animal Breeding and Genetics, 124, 302–307.
Wittwer, C., Löhring, K., Drögemüller, C., Hamann, H., Rosenberger, E., & Distl, O. (2007b). Mapping quantitative trait loci for osteochondrosis in fetlock and hock joints and palmar/plantar osseus fragments in fetlock joints of South German Coldblood horses. Animal Genetics, 38, 350–357.
Wittwer, C., Dierks, C., Hamann, H., & Distl, O. (2008). Associations between candidate gene markers at a quantitative trait locus on equine chromosome 4 responsible for osteochondrosis dissecans in fetlock joints of South German Coldblood horses. Journal of Heredity, 99, 125–129.
Wittwer, C., Hamann, H., & Distl, O. (2009). The candidate gene XIRP2 at a quantitative gene locus on equine chromosome 18 associated with osteochondrosis in fetlock and hock joints of South German Coldblood horses. Journal of Heredity, 100, 481–486.
Ytrehus, B., Carlson, C. S., & Ekman, S. (2007). Etiology and pathogenesis of osteochondrosis. Veterinary Pathology, 44, 429–448.
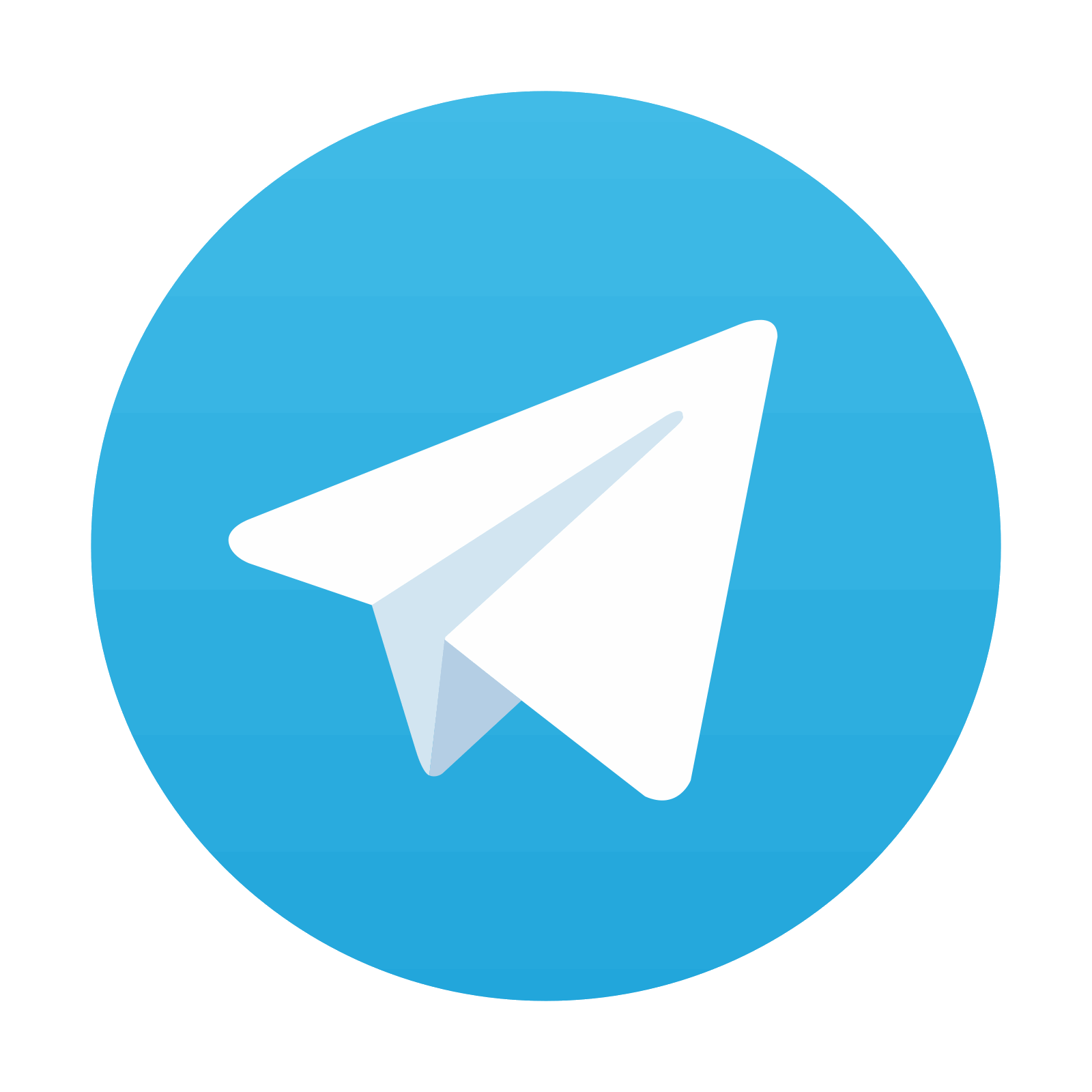
Stay updated, free articles. Join our Telegram channel
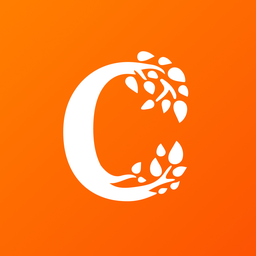
Full access? Get Clinical Tree
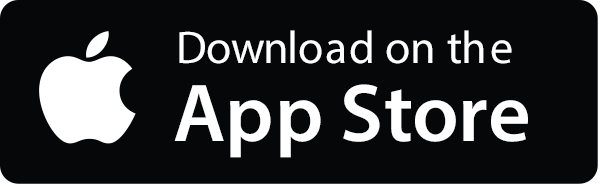
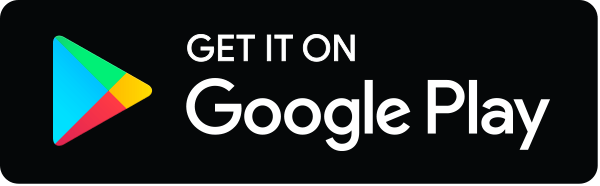