7 Genetic Aspects of Male Reproduction Peter J. Chenoweth1* and Francoise J. McPherson2 1ChenoVet Animal Andrology, Wagga Wagga, New South Wales, Australia; 2Charles Sturt University, Wagga Wagga, New South Wales, Australia Genetic disorders are those caused by abnormalities or defects in genes or chromosomes. Although many genetic disorders are transmitted via parental genes, others are a result of DNA changes or mutations that are not necessarily heritable. Congenital disorders, in contrast, are those that exist at birth (though they may become first evident either earlier or later). These may be caused by a number of factors, including genetic disorders. However, they can also be caused by developmental anomalies, infections, the uterine environment, and metabolic, nutritional and toxic factors, in addition to joint genetic–environmental and epigenetic influences. Those that are proven to be heritable may be transmitted via single gene (or Mendelian) inheritance, or via complex mechanisms that may involve multiple genes as well as environmental effects. For examples of conditions subject to the former mode of inheritance in cattle, readers are referred to the web site (http://dga.jouy.inra.fr/lgbc/mic2000/). Literally thousands of genes are involved, directly or indirectly, in the production of mammalian spermatozoa, and those genes involved in reproduction are generally less conserved than other genes (Leeb, 2007). It is estimated that 10% of genes in the human genome are associated with spermatogenesis and that genetic factors cause 15–30% of human male infertility (Yoshida et al., 1997). In turn, male genetic factors contribute to an estimated 50% of human infertility (Tanaka et al., 2007). Similar estimates may be assumed for domesticated species, in which there is potential for the widespread dissemination of genetic problems, which may be caused by chromosomal or single gene disorders, Mendelian disorders of sex differentiation, genetic disorders either directly or indirectly affecting male fertility, mitochondrial DNA (mtDNA) mutations, Y chromosome deletions, epigenetic effects and multifactorial disorders (see reviews by Wieacker and Jakubiczka, 1997; Shahet al., 2003; Martin, 2008; Visser and Repping, 2010; and Hofherr et al., 2011). The undifferentiated embryo undergoes sex determination, which is described as a developmental decision that sends it along one of two major avenues towards sexual dimorphism. Sexual differentiation then occurs, with phenotypic sex being determined under the influence of factors produced by the gonadal precursors (Biason-Lauber, 2010). In mammals, early gonadal differentiation is the major factor determining sexual dimorphism, in which distinguishing differences in structures or features between individuals of different sexes are termed secondary sexual characteristics (McPherson and Chenoweth, 2012). Early gonadal differentiation also marks an important phase in which errors in sexual development may occur, with the status of the SRY gene, located on the Y chromosome, playing a major role in establishing ‘maleness’ and also being implicated in XY female gonadal dysgenesis (Biason-Lauber, 2010). Translocation of the SRY gene to the X chromosome is strongly implicated in human cases of XX maleness (Müller, 1993). Developmental anomalies occurring in domesticated animals that adversely affect male fertility to varying degrees include the following: undescended testes, segmental aplasia/hypoplasia of the Wolffian duct system, testicular hypoplasia, hypospadia, freemartinism and different manifestations of intersex. Chromosomal anomalies represent a major cause of infertility in human males (Martin, 2008; Hofherr et al., 2011) and this probably applies to domestic animals as well. Chromosome disorders were found in approximately 2–9% of sub-fertile human males, with Klinefelter’s syndrome (47,XXY) being one of the most common (Chandley and Cooke, 1994). Those disorders involving autosomal chromosome rearrangements, such as translocations or inversions, are essentially paternal in origin. This includes approximately 35% of Robertsonian translocations (where two acrocentric chromosomes fuse near the centromere, with the loss of the short arms), which occur in cattle, with resultant reduced fertility of both males and females (Gustaffson, 1979). Reciprocal (non-Robertsonian) translocations have been associated with reduced fertility, abortions and birth defects in humans (Shah et al., 2003) as well as with early embryonic deaths and reduced litter sizes in swine (Feitsma and De Vries, 2006). Relevant reviews on chromosomal defects in domestic animals and their effects include those of Popescu and Tixier (1984) and Ducos et al. (2008). Numerical chromosome abnormalities – such as deletion, trisomy and triploidy – also contribute to early embryonic mortality in domestic animals (Courot and Colas, 1986). A notable example of this is encountered in cats, where the genes for either orange or black colours, but not both together, are located on the X chromosome. Thus, males that exhibit both colours (such as calico or tortoiseshell individuals) are polyploid and hence infertile (see Chapter 8, Root Kustritz). In infertile men with poor semen quality, a direct relationship has been suggested between the impairment of spermatogenesis (as reflected in morphologically and cytogenetically abnormal germ cells) and rates of baseline aneuploidy in normal spermatozoa (Bernadini et al., 1998), with most sperm aneuploidies being associated with lowered fertilization rates as well as reduced embryo survival. A genetic basis for spermatogenic failure has been associated with deletions on the human Y chromosome (Krausz et al., 1999). Such deletions occur at a relatively high level, indicating a susceptibility of the Y chromosome to loss of genetic material (Aitken and Sawyer, 2003; Shah et al., 2003; Hofherr et al., 2011). In man, Y micro-deletions are notable in the AZF (azoospermia factor) regions, supporting the theory that DAZ (deleted in azoospermia) genes play an important role in male fertility (Hofherr et al., 2011). In recent years, advances in intracytoplasmic sperm injection, or ICSI (Chemes and Rawe, 2003), as well as single sperm typing and synaptonemal complex analysis (Martin, 2008), have enhanced understanding of the relationships between specific characteristics of individual sperm and fertilization, as well as post-fertilization development. More specifically, strong links are now evident between sperm morphological abnormalities and structural chromosomal aberrations, with the latter being significantly elevated in human sperm with head abnormalities (Rosenbusch et al., 1992; Lee et al., 1996; Lewis-Jones et al., 2003; Sun et al., 2006; Encisco et al., 2011). For instance, chromosomal anomalies were four times more likely in sperm that were amorphous, round or elongated (Lee et al., 1996), and abnormal karyotypes were significantly higher in oocytes injected with severely deformed sperm heads (Kishikawa et al., 1999). Relationships occur between poor chromatin structure, DNA damage, abnormal morphology and fertility in bull sperm (Ballachey et al., 1987; Sailer et al., 1996; Karabinus et al., 1997; Smorag et al., 2000; Ostermeier et al., 2001; Vieytes et al., 2008; Encisco et al., 2011). As an example, the well characterized diadem/crater defect of sperm, found in many species following spermatogenic stress, is linked with failure of normal chromatin condensation (Larsen and Chenoweth, 1990) and represents part of a choreographed continuum of sperm head damage following stress (Chenoweth, 2005). In humans, large vacuoles in the sperm head have been associated with a failure of chromatin condensation (Boitrelle et al., 2011). If a significant proportion of the sperm population is observed to be morphologically abnormal, it is likely that the causative spermatoxicity has adversely affected other, apparently normal, sperm in the population (Vogler et al., 1993). Due to the limitations of sperm morphology assessment using light microscopy, sperm that appear to be morphologically normal may have defective chromatin or other cellular components (Ballachey et al., 1988; Chemes and Rawe, 2003). These latter authors proposed that certain sperm anomalies (e.g. flagellar defects) have a better fertility prognosis than others (e.g. acrosome, sperm chromatin and neck defects). Similar considerations have contributed to the development of several approaches to the categorization of sperm defects, viz.: (i) compensable and uncompensable, based on observed sperm morphology (Saacke et al., 2000); and (ii) systematic versus non-systematic (or non-specific) sperm abnormalities, based on whether they are considered to be due to pathological and/or environmental causes, or to have a proven or suspected genetic origin, respectively (Chemes and Rawe, 2003). Sperm with severe head malformations often fail to successfully traverse the female tract and reach the site of fertilization and, therefore, can be regarded as compensable defects. However, those sperm with more subtle (i.e. non-head distorting) forms of the diadem/crater defect can gain access to the ovum, leading to both lowered fertility and embryo quality (Miller et al., 1982; Saacke et al., 1994), thus fulfilling the definition of uncompensable sperm defects. Encisco et al. (2011) used the Sperm Chromatin Dispersion test in conjunction with Blom’s (1972) major/minor categorization of sperm abnormalities to illustrate the relationship between abnormal bull sperm morphology and DNA fragmentation – a relationship that accounted for 61.34% of all deformed sperm, and 46.88% of major defects. In certain major sperm defects, i.e. double forms, narrow head at base, small heads and proximal droplets, DNA fragmentation occurred in all recognized affected sperm. It can be concluded, then, that sperm with DNA/chromatin abnormalities often show structural abnormalities, which may be identified during routine assessment of sperm morphology (Martin and Rademaker, 1988; Chemes and Rawe, 2003), although caution should be exercised when attempting to extend this generalization to assume that all damaged sperm can be so recognized (Martin and Rademaker, 1988). This is because many examples of damaged sperm DNA or chromatin integrity do not display obvious morphological abnormalities (Sutovsky et al., 2001; Shah et al., 2003), even though they may cause failure of fertilization or postfertilization loss (Smorag et al., 2000). In summary, these findings support the following concepts (Chenoweth, 2011): first, that abnormal sperm head morphology is often associated with DNA damage (Erenpreiss et al., 2006); secondly, that the major cause of DNA damage in the male gamete is oxidative stress (Aitken, 2002; Lewis and Aitken, 2005); and thirdly, that sperm DNA abnormalities are a major cause of malefactor sub-fertility. Finally, routine sperm assessment parameters are only partially successful in identifying such damage. Sperm morphology, particularly of the head region, is often linked with in vitro fertilization (IVF) success rates, but it is apparent that this relationship is less strong than it is with natural fertilization (Windt and Kruger, 2004). Further, the development of ICSI for the treatment of male-factor infertility has resulted in successful fertilizations using sperm that are seriously impaired, as well as those in which normal buffering mechanisms are deficient (Horsthemke and Ludwig 2005). In both cases, a number of barriers that would normally restrict fertilization by abnormal sperm are bypassed, which increases the possibility of subsequent genetic problems, including chromosomal abnormalities, genetic disease and reproductive problems in offspring from ARTs being implicated in disturbances of epigenetic mechanisms and related disorders of genomic imprinting (Fortier and Trasler, 2011). Male effects on reproduction may be positive or negative and obvious or subtle. They include not only direct effects on the ability to mate and to achieve fertilization, but also indirect effects on the quality and viability of the conceptus. Embryo development relies not only upon maternal but also on paternal factors (Duranthon and Renard, 2001). For example, differences have been shown to occur among bulls for embryo survival and development, in both in vivo and in vitro systems (Hillery et al., 1990; Saacke et al., 2000). Earlier work showed that bulls of low fertility used for artificial insemination (AI) gave higher rates of embryonic loss than did bulls of high fertility (cited by Courot and Colas, 1986). Similarly, with sheep, Maxwell et al. (1992) reported that rams of differing genetic lines differed in subsequent rates of embryonic loss despite comparable fertilizing capacity. Differences in bull reproductive success are often not predictable on the basis of conventional semen examination. However, recent advances in technologies such as IVF and ICSI have allowed a vastly improved understanding of the causes of variation in male gamete ‘success’. Here, bull differences have been reported for IVF rates, initiation and length of the zygotic S-phase, and embryo cleavage and development (cited by Schneider et al., 1999), as well as in sperm in vitro ability to access the ovum and accessory sperm numbers (Nadir et al., 1993). The effects of the male on early pregnancy loss and abortion have been associated with implicating factors such as elevated ambient and scrotal temperatures, ‘out-of-season’ breeding, and immature and aged sperm (Saacke et al., 2000). It is interesting and useful to note that many of these stressors induce similar patterns of spermatogenic stress. For example, in bulls, a stereotyped response results in a predictable cascade of identifiable sperm abnormalities such as retained cytoplasmic droplets, head loss (decapitation) and diadem-crater defects, with consequent adverse effects on fertilization or subsequent development (Saacke et al., 2000). In pigs, those sperm characteristics associated with successful in vitro penetration of oocytes included all conventional semen parameters (Gadea and Matas, 2000), with most of these being significantly correlated with each other. Flowers (2002, 2008) reported that individuals differed in the insemination dose required to consistently produce the greatest number of pigs. Traditional semen assessments tended to be predictive of litter size up to a point, but beyond this, differences in litter size could not be attributed to observed semen differences. Such results illustrate difficulties in predicting sperm fertility from one or two tests, while reinforcing the need to identify biologic al markers that reflect unifying mechanisms for many aspects of sperm damage. Even though the heritability of male fertility in domesticated animals is generally considered to be low, some male traits associated with fertility are relatively heritable. For example, adjusted testicular size (measured as scrotal circumference) is moderately to highly heritable in beef breed bulls, where it is favourably associated with age at puberty in related females (Brinks, 1994). Comparable estimates have been obtained in boars (Toelle et al., 1984; Toelle and Robinson, 1985; Young et al., 1986). In turkeys, selection for increased semen yield doubled sperm numbers within five generations (Nestor, 1976). Semen production also increased when turkeys were specifically selected for increased egg yield, and decreased when selection was for increased body weight at 16 weeks (Nestor, 1977). In rams, scrotal circumference was favourably linked with other male fertility traits; genetic correlations were 0.54 with individual sperm motility and –0.75 with percentage abnormal sperm (Rege et al., 2000). In French dairy cattle, sperm quality traits such as motility were moderately highly heritable; genetic correlations between volume and semen quality traits tended negative, whereas those between concentration and semen quality traits were the opposite (Druet et al., 2009). Some findings from studies on the heritabilities of semen traits in boars, bucks and bulls are shown in Table 7.1. Heterosis in pigs boosts semen traits such as semen volume, total sperm and sperm viability (Smital et al., 2004). Genetic lines of boars differed in sperm output, which varied by as much as 30 × 109 spermatozoa or more per ejaculate, indicating the possibility for selection of boars on the basis of improved sperm production as well as fertility (Flowers, 2008). In a study using 463,130 litter records from Yorkshire, Duroc, Hampshire and Landrace pigs, estimates of sire effects for traits such as pigs born alive, litter weight at 21 days and number weaned ranged from 0.02 to 0.05. Although sire effects were less than environmental effects (0.03–0.08), they were greater than maternal effects (0.00– 0.02) across all breeds, providing encouragement for improving litter traits via sire selection (Chen et al., 2003). In North American Holstein cattle, sirepredicted transmitted ability for twinning was estimated to be 1.6–8.0%, which is considered sufficient to use sire selection as a means of reducing undesirable twinning in dairy cattle (Johanson et al., 2001). Perinatal calf deaths up to 48 h after birth can also be linked to the sire, though the heritability for this parameter in bulls is low (Freeman, 1984). Stallions are normally not selected on the basis of fertility, but genetic markers have been identified for stallion fertility (Leeb et al., 2005). Three candidate genes have been identified to allow selection of stallions based on reproductive potential. These candidate genes code for equine cysteine-rich secretory proteins (CRISPs) and together make up six polymorphisms as genotyped in 107 Hanoverian stallions. Stallions heterozygous for the CRISP3 AJ45996c.+622 G>A single nucleotide polymorphism (SNP) have significantly reduced fertility compared with homozygous stallions, which translates into a 7% reduction in conception rate per cycle (Hamann et al., 2007). Other marker genes affecting equine pregnancy rate per oestrus cycle are SPATA1 and INHBA (Gieseke et al., 2010). SPATA1 codes for a testis-specific protein involved in shaping the sperm head, and conception rates per oestrus cycle are significantly higher for SPATA1 heterozygous stallions than for homozygous stallions, with a dominance effect of 4.1% (Gieseke et al., 2009). Table 7.1. Heritabilities of semen traits in boars, bucks and bulls. In addition to these relationships, a large number of conditions, which are either directly or indirectly genetically influenced, can adversely affect male fertility. These may exert their influence not only via sperm and semen traits, but also in relation to mating ability and sperm delivery capabilities. Reviews of genetic disorders linked with male infertility are provided by Wieacker and Jakubiczka (1997), Shah et al. (2003) and Martin (2008). In this discussion, the focus is upon conditions affecting sperm production and semen quality. Readers interested in the other aspects, particularly in relation to bulls, may wish to consult Chapter 12 (Brito), which has descriptions of conditions such as testicular hypoplasia, congenitally short penis, congenitally short retractor penis muscle, persistent penile frenulum, penile spiral deviation, aplasia/hypoplasia of efferent duct systems and congenital erectile dysfunction. Both libido and mating ability in bulls are influenced by genetic factors (Bane, 1954; Chenoweth, 1983) and differences in sex drive and mating behaviour have been reported among breeds of bulls (Chenoweth and Osborne, 1975; Chenoweth et al., 1979) and rams (Chenoweth, 1981), and also between boar lines (Signoret, 1970). Differences in aspects of sperm production and semen collection have been observed between beef and dairy breeds of bulls in general (Chenoweth, 1983). Bulls of Bos indicus derivation have been considered to be more sexually ‘sluggish’ than their European counterparts (Chenoweth, 1981), although this does not necessarily disadvantage breeding success (Chenoweth, 1994). In a longitudinal study in which 47 stallions (aged 2–26 years), representing seven breeds, were bred to 1664 mares, breed of stallion as well as paternal age and season significantly influenced mare breeding outcomes (Dowsett and Pattie, 1982). Semen factors that were associated with percentage of pregnancies per mare service were volume, concentration, live sperm and total number of sperm (Dowsett and Pattie, 1982). In another stallion study, sire effects on semisibling semen characteristics were significant for sperm motility, semen volume and sperm concentration (Parlevliet et al., 1994). In chickens, Wyandotte and Delaware roosters were less fertile than their Leghorn counterparts (Kirby et al., 1989), and Wyandotte sperm exhibited less metabolic capacity than either Leghorn or Delaware sperm (Kirby and Froman, 1991). Roosters homozygous for the rose comb allele (R/R) were sub-fertile compared with heterozygous roosters of genotypes R/r or r/r, with this depression of fertility being associated with reduced sperm motility and metabolic activity (McLean and Froman, 1996). The reduced sperm motility of R/R roosters has been shown to be associated with a significantly reduced intracellular ATP concentration and reduced glucose uptake (McLean et al., 1997). Sperm morphology can also vary between breeds, as shown in a study comparing eight breeds of Indian buffalo (Aggarwal et al., 2007). Similarly, differences in sperm midpiece length were encountered when comparing Holstein, Friesian, Belgian Blue, Charolais, Limousin and Aberdeen Angus bulls (Shahani et al., 2010). Here, the Belgian Blue and Limousin breeds displayed the longest average midpiece length, while Angus bulls had the shortest. Furthermore, the two dairy breeds showed a negative correlation between sperm midpiece length and 49 day non-return rate. Finally, sperm head morphometry as determined by computerized Fourier analysis differed significantly among 241 mature rams from 36 different sheep dairy flocks (Maroto-Morales et al., 2010). Breeding programmes for domestic livestock generally seek to maximize genetic gain while minimizing inbreeding. Inbreeding adversely affects reproductive traits (such as sperm production and semen quality) in two ways: first by reducing heterozygosity, leading to inbreeding depression in those traits affected by directional dominance; and, secondly, via random genetic drift in which advantageous alleles may be lost (Sonersson, 2002). Earlier work (Salisbury and Baker, 1966b) indicated that inbred bulls had more sperm abnormalities than line-crossed bulls, and that the degree of inbreeding is linked with the proportion of abnormal seminiferous tubules (Carroll and Ball, 1970). More recently, inbreeding in endangered ungulates has been linked with high levels of DNA fragmentation and poor sperm quality (Ruiz-Lopez et al., 2010). In foxhounds, outbred dogs produced a greater ejaculate volume with significantly (P<0.025) higher sperm concentration and more sperm/ejaculate than did inbred males. There was also a trend towards improved sperm motility, ejaculate volume and testes volume for outbred dogs than for inbred dogs (Wildt et al., 1982). In dachshunds, Gresky et al. (2005) showed that inbreeding decreased litter sizes and at the same time increased the number stillborn. Inbreeding can affect not only sperm quality or quantity, but also the occurrence of reproductive organ disorders. For example, undescended testicles, or cryptorchidism, is a common genetic disorder in dogs (Veronesi et al., 2009). A relatively high incidence of cryptorchidism was detected in a colony of Miniature Schnauzers. Of twelve cases examined, nine of the Miniature Schnauzers were descendants of the same sire, either directly or indirectly. Bilateral cryptorchidism was associated with a higher degree of inbreeding than was unilateral cryptorchidism (Cox et al., 1978). Inbreeding in horses has been associated with increased embryonic loss in Norwegian trotter mares (Klemetsdal and Johnson, 1989) and reduced conception and foaling rates in Standardbred mares (Cothran et al., 1984), though it is conceded that mare management factors could also be involved. When the inbreeding coefficient in Shetland pony stallions exceeded 2%, there was a significant reduction in sperm motility and the percentage of morphologically normal sperm (van Eldik et al., 2006). Inbreeding in dairy cattle has resulted from extensive use of artificial insemination, with a small number of elite bulls being used for a large number of inseminations (Soares et al., 2011). An added issue is that AI success is declining in highly selected dairy cattle populations (Karoui et al., 2011). In a study by Hudson and van Vleck (1984), the percentage of inbred bulls for Ayrshire, Guernsey, Holstein, Jersey and Brown Swiss breeds in the north-eastern USA was 4.7, 2.8, 12.1, 3.9 and 7.6%, respectively. The average inbreeding coefficients were 0.39, 0.17, 0.36, 0.14 and 0.26, respectively (Hudson and van Vleck, 1984). A more recent study revealed that mean inbreeding coefficients for US dairy cattle have increased to 0.3–0.4, with some animals recording a value greater than 0.5. Milking Shorthorns recorded the highest annual increase in inbreeding level overall, while Holstein cattle recorded the greatest rate of change in the annual increase in inbreeding level (Wiggans et al., 1995). In beef bulls, semen traits deteriorated with increasing inbreeding coefficients (Smith et al., 1989). Although inbreeding coefficients in Austrian dual-purpose Simmental (Fleckvieh) bulls were low (mean 0.013), semen quality traits such as volume, concentration, motility, number of spermatozoa per ejaculate and percentage of viable sperm all showed evidence of inbreeding depression (Maximini et al., 2011). Methods for minimizing the adverse effects of inbreeding in selection and genetic conservation schemes for livestock breeding, especially in small (≤50) populations, have been described by Sonersson (2002). Classification systems for abnormal morphology of sperm have included those based on: (i) site of origin – primary or secondary (Blom, 1950); (ii) functional significance – major or minor (Blom, 1972); and (iii) relative quantitative significance – compensable or uncompensable (Saacke et al., 1994), and systematic or non-systematic (Chemes and Rawe, 2003). 1. They are characterized as ‘primary’ sperm defects (i.e. they occur during spermatogenesis). 2. They constitute a substantial proportion (at least 10–15%) of the sperm population. 3. They persist over time. 4. They are linked with male infertility or sterility. 5. They are potentially heritable. This last criterion is particularly relevant to the present discussion. Under natural selection, there is discrimination against genetic factors that result in infertility, though this is not necessarily the case in modern production systems in which artificial selection permits sub-fertile males to propagate infertility factors that are genetically influenced. These factors include genetic sperm defects, whereby, at least in bulls, ‘some types of defects appear in the semen at a fairly constant rate and in a very high proportion of the sperm population without any indication of environmental influence. Such defects may be presumed to be rooted in the bull’s genome and the prognosis for future improvements in semen quality would be very poor’ (Barth and Oko, 1989). Terms employed to describe generalized characteristics of sperm and semen include: • oligospermia or oligozoospermia – decreased number of spermatozoa in semen; • aspermia – a complete lack of semen; • hypospermia – reduced seminal volume; • azoospermia – absence of sperm in the ejaculate; • teratospermia – a majority of sperm with abnormal morphology; and • asthenozoospermia – low sperm motility. The conditions of asthenozoospermia, azoospermia and teratospermia are included in the discussions below under the heading ‘Systematic sperm defects’. Although environmental factors are considered to be the most common factors affecting sperm function and form, there is a growing list of sperm defects in domestic animals that are considered to be of genetic origin. Genetic sperm defects are identified as consistent defects that are genetically transmitted. A number of sperm defects in different species have been established to be genetic, and this number is increasing with improvements in technologies and knowledge. However, while some sperm defects may be caused either by genetic or environmental influences, and some may be due to an interaction of environment and genetic predisposition, yet others are likely to be of as yet unproven genetic origin. Thus the term ‘systematic sperm defects’ (Chemes and Rawe, 2003) is used in this chapter to allow the inclusion of all such categories. In the following discussion, and in the accompanying table (Table 7.2), an attempt is made to identify a number of sperm defects that fall into this category. Defective sperm motility, or asthenozoo-spermia, is a classic example of a trait that can be attributable to either genetic or environmental effects. For example, simple coiled tails (term that includes the distal midpiece reflex) are among the most common of sperm defects and are commonly associated with one or more of a variety of non-genetic aetiologies. In general, the condition of ‘non-specific flagellar abnormalities’ (NSFAs), which in turn results in asthenozoospermia, is most commonly reversible and is secondary to conditions such as infections, varicocele, immunological disorders and others (Chemes, 2000). However, pathological defects of flagellar structure are also implicated, as described by Chemes et al. (1998), in men with severe asthenozoospermia. A comprehensive review of structural pathologies associated with asthenozoospermia in humans is provided by Chemes (2000) and Chemes and Rawe (2003). Table 7.2. Systematic sperm defects.a a The defects of asthenozoospermia (immotile sperm), necrozoospermia (dead sperm) and teratospermia (the production of abnormal sperm) are not included in this table but are discussed in the text. Otherwise, the order of defects followed in the table is that used in the text, but with extra categories of defects added, in no particular order. A complete lack of sperm in the ejaculate can be due to either a failure of spermatogenesis or to causes in sperm transport. With the latter category, sperm may be prevented from contributing to the ejaculate by blockages or occlusions in the extragonadal duct system, or because of an ejaculatory dysfunction. In dogs, for example, the possibility of occlusion being the cause may be determined by measurement of alkaline phosphatase (ALP) in the seminal plasma, as ALP originates from the epididymis and seminiferous tubule epithelium (Kutzler et al., 2003). If the concentration of ALP in seminal fluid is greater than 5000 IU/l, the ejaculated sample contains fluid from those tissues and occlusion may be ruled out. Testicular causes of azoospermia include failure of spermatogenesis, which can be caused by a number of factors; these include hormonal, immunological, congenital, toxic, pathogenic and traumatic factors. Here, deletions within the AZF region (containing subregions AZFa, AZFb and AZFc) on the Y chromosome play an important role, representing 10–15% of cases of azooand oligospermia (Shah et al., 2003). Within the AFZc region, the DAZ gene, which is deleted in azoospermia, is considered to be important in spermatogenetic control, as its deletion is commonly detected in infertile men (Shah et al., 2003). Teratospermia, or the production of >60% morphologically abnormal sperm, can be due to either genetic or environmental causes, as well as to interactions between these two factors. This defect appears to be especially prevalent in domestic cats and wildcats, and occurs in 70% of felid species (Pukazhenthi et al., 2001). One assumption is that this high level of teratospermia is associated with high levels of inbreeding, although conclusive evidence is still pending. Inbreeding levels tend to be high in cheetahs and other endangered wild cats (O’Brien et al., 1983, 1985) that are encountering population decline due to habitat loss, poaching, disease and other causes (Wildt et al., 1995). In felids, spermatozoa from teratospermic individuals are incapable of fertilizing oocytes owing to an inability to undergo capacitation and the acrosome reaction (Pukazhenthi et al., 2006). Suggestions of a genetic basis for teratospermia have also come from studies of a stallion (Brito et al., 2010) and a dog (Rota et al., 2008). KNOBBED ACROSOME (KA). This defect was first observed in a sterile bull of the Friesian breed (Teunissen, 1946), where it was subsequently shown to have an autosomal sex-linked recessive mode of genetic transmission (Donald and Hancock, 1953). It has been associated with infertility in bulls, boars, rams and dogs (Teunissen, 1946; Saacke et al., 1968; Barth, 1986; Soderquist, 1998; Santos et al., 2006). In boars, the defect has been suspected of both dominant (Wohlfarth, 1961) and sex-linked recessive (Bishop, 1972) modes of transmission. In cattle, there are indications that it exists as a genetic defect within the Angus breed in North America (P.J. Chenoweth, unpublished) and there is a suggestion of a genetic linkage in the Charolais breed (Barth and Oko, 1989). Four related male dogs exhibiting high KA levels were incapable of achieving pregnancies with otherwise fertile bitches (Santos et al., 2006). In the bull, this abnormality is commonly observed as either a refractile, thickened, protruding acrosomal apex or as an indented sperm apex (Thundathil et al., 2000b). Electron microscopy often reveals a cystic region (the ‘cystic apical body’) containing vesicles with inclusions, as well as abnormal fusion of acrosomal membranes (Bane and Nicander, 1966; Cran and Dott, 1976). There is also often a bending back, or abrupt termination, of the sperm nuclear material (Thundathil et al., 2000b), giving the appearance that the sperm head apex has been cleanly incised. Elevated levels of knobbed acrosomes in bull semen are associated with either genetic or environmental factors. With the latter, the elevated incidence is usually transitory and occurs in concert with other signs of spermatogenic dysfunction (i.e. increased sperm abnormalities in general, including nuclear vacuoles). A genetic cause is suspected when relatively high proportions (>20%) of sperm exhibit the KA defect in the absence of frequent numbers of other sperm abnormalities, and this persists indefinitely (Thundathil et al., 2000b; Chenoweth, 2005). In Canada, 78 of 1331 (5.9%) bulls had sperm with knobbed acrosomes (Barth and Oko, 1989). In contrast, in the author’s laboratory (P.J. Chenoweth, unpublished), a prevalence of 6.74% was observed in bulls in a known affected Angus herd. In pigs, an incidence for KA of 7.6% was reported in the Finnish Yorkshire breed (Kopp et al., 2008), in which the syndrome was associated with immotile short-tail sperm defects. Two candidate genes, both located on pig chromosome 15, have been linked with knobbed acrosomes: HECW2 and STK17b (Sironen et al., 2010). In comparison with ‘normal’ boars, those with the KA defect were reported to have seminiferous tubules of smaller diameter, as well as a reduced number of Sertoli cells. Sperm containing KA are either unable to attach to ova (Buttle and Hancock 1965), or this capability is much reduced (Thundathil et al., 2000b). This defect would, therefore, appear to be eligible for inclusion within the ‘compensable’ category of sperm defects, in which increasing sperm numbers could compensate for damaged sperm in terms of inseminate fertility. In fact, several studies would appear to support this classification. For example, differences were observed in the fertility of a KA-affected ram when natural service was compared with AI (Soderquist, 1998). Another thread of evidence for including KA in the compensable category comes from work that showed that the proportion of bull KA sperm decreased during transit through the female genital tract (Mitchell et al., 1985). Despite such findings, in vitro studies have shown that apparently normal sperm in ejaculates from animals affected by KA may also have compromised fertility (Thundathil et al., 2000b). Further studies indicate that these apparently morphologically normal sperm have plasma membrane damage, and show premature capacitation, a spontaneous acrosome reaction and impaired chromatin condensation (Thundathil et al., 2002). Thus, the KA defect may encompass both ‘compensable’ and ‘uncompensable’ characteristics. As bulls with apparently similar proportions of the defect may vary in infertility, this variation may be either due to such unrecognized causes of sperm dysfunction, or to the numbers of undamaged sperm reaching the fertilization site. RUFFLED AND INCOMPLETE ACROSOMES. Ruffled and incomplete acrosomes have been reported in sub-fertile bulls (Saacke et al., 1968). Here, ruffled acrosomes had an irregular staining pattern that provided a wrinkled, or ruffled, appearance. Incomplete acrosomes showed an irregular margin, giving the appearance that part of the acrosome was missing or incomplete. A genetic basis was suggested by the occurrence of all three acrosome defects (knobbed, ruffled and incomplete acrosomes) in four sons of a sub-fertile Holstein sire. Some similarity has also been drawn with acrosome abnormalities described in ‘genetically-determined quasisterile’ male mice (Rajasekarasetty, 1954). DECAPITATED SPERM DEFECT (HEADLESS FLAGELLAE, DISINTEGRATED, ACEPHALIC, MICROCEPHALIC, PIN HEAD)
Introduction
Developmental Anomalies
Chromosomal and DNA Anomalies
Potential Adverse Genetic Sequelae of Assisted Reproductive Technologies (ARTs)
Male Fertility
Breed effects
Inbreeding
Categorization of Defects of Sperm and Spermatogenesis
Terminology for generalized sperm and semen defects
Systematic sperm defects
Asthenozoospermia
Azoospermia
Teratospermia
Defects of the sperm acrosome
Defects of the sperm head
Stay updated, free articles. Join our Telegram channel
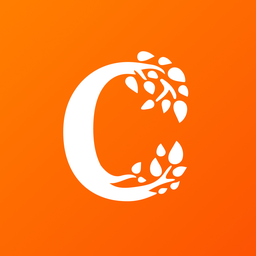
Full access? Get Clinical Tree
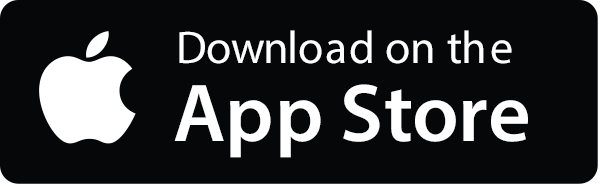
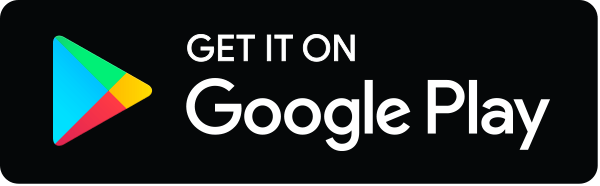