Fig. 8.1
Diagram of the key elements of a GABAergic synapse
8.2 Introduction to GABAA Receptor-Mediated Neurotransmission
8.2.1 GABA Synthesis, Release, Transport, and Degradation
GABA is synthesized from glutamate by two isoforms of glutamate decarboxylase (GAD), GAD67 and GAD65, named for the molecular size of the proteins, 67 and 65 kDa, respectively. Nearly all neurons in the SCN of the mammalian species examined contain GAD (Van den Pol 1980; Card and Moore 1984; Moore and Speh 1993; Jansen et al. 1994; O’Hara et al. 1995; Gao and Moore 1996; Belenky et al. 2003; Jobst et al. 2004). GAD67 and GAD65 genes and proteins have only 65 % identity, and these unique structural features underlie the differential functional patterns of GAD67 and GAD65 activity (Fenalti et al. 2007). In addition, GAD67 and GAD65 genes contain distinctive regulatory elements suggesting that different intracellular signaling pathways regulate the transcription of the two enzymes.
GAD67 and GAD65 are members of the pyridoxal-5′-phosphate-dependent enzyme superfamily. The enzyme is active when pyridoxal-5′-phosphate is bound and inactive when it is absent (Battaglioli et al. 2003). When glutamate is decarboxylated to produce GABA, the pyridoxal-5′-phosphate may remain bound in which case the GAD enzyme remains active. Alternately, the GAD is inactive if the pyridoxal-5′-phosphate unbinds. GAD67 is constitutively active while a significant fraction of GAD65 is inactive due to structural differences in the pyridoxal-5′-phosphate regulatory site, which is a result of the unbinding reaction occurring at a significantly higher rate in GAD65 compared to GAD67 (Fenalti et al. 2007). The different levels of constitutive activity and the localization of GAD67 to the soma and dendrites and GAD65 to membranes of axon terminals and synaptic vesicles suggest unique roles for GAD67 and GAD56 in GABAergic neurotransmission. For example, inactive GAD65 may provide a reservoir of synthetic capacity to rapidly increase the amount of GABA available for synaptic release.
GAD67 and GAD65 have different patterns of expression in the SCN. GAD67 mRNA showed no 24-h rhythm of expression in rats although one study noted higher levels at CT0 and CT 14 (Cagampang et al. 1996; Huhman et al. 1996, 1999). Panda et al. (2002) observed a 24-h rhythm of GAD67 expression in the mouse SCN. The disparate observations may reflect differences in the sensitivity and signal-to-noise ratio of the autoradiographic in situ hybridization method compared to high-density microarrays. No rhythm in GAD65 mRNA was observed in mice maintained in constant darkness (DD) (Panda et al. 2002). In rats, GAD65 expression has a diurnal rhythm with a peak at ZT0 and a trough at ZT20 (rats; light:dark (LD) 12:12 h). The increase was larger in the dorsomedial portion of the SCN (Huhman et al. 1996). A similar pattern of expression was observed in rats (LD 12:12 h) on the first day after release into DD (Cagampang et al. 1996). However, the rhythm of GAD65 mRNA expression disappeared in rats after 9 days in DD suggesting that the light input pathways regulate GAD65 expression (Huhman et al. 1999). Similarly, GAD activity showed a diurnal rhythm with levels peaking late in the day and early in the night (Aguilar-Roblero et al. 1993). Together these data indicate that GAD65 expression and activity increase in parallel to the increased frequency of GABA-mediated synaptic currents observed during the subjective day (Itri and Colwell 2003; Itri et al. 2004).
GABA is loaded into synaptic vesicles by the vesicular GABA transporter (VGAT). VGAT is also called the vesicular inhibitory amino acid transporter (VIAAT) and will also transport glycine into synaptic vesicles (McIntire et al. 1997; Gasnier 2004). The VGAT moves GABA into synaptic vesicles utilizing the H+ gradient, an electrochemical gradient established by an H+-ATPase (Hsu et al. 1999). Trafficking signals target the VGAT to specific vesicles allowing specific pools to be filled more rapidly or more fully (Santos et al. 2013). In addition, the regulation of the VGAT activity by phosphorylation or other biochemical modifications will regulate the GABA concentration in vesicles (Hsu et al. 1999). Reducing the amount of GABA in synaptic vesicles reduces the amplitude and frequency of GABAA receptor-mediated currents (Riazanski et al. 2011). However, the importance of these mechanisms in regulating SCN neural network activity has not been studied. Neither VGAT mRNA nor vesicular VGAT expression was found to be rhythmic in constant darkness or in a light–dark cycle (Panda et al. 2002; Darna et al. 2009). Interestingly, mice housed in a LD (12:12) cycle had higher levels of VGAT expression compared to animals housed in DD (Darna et al. 2009). It would be interesting to know if animals housed in LD have higher vesicular GABA concentrations and larger and more frequent GABAA receptor-mediated currents than those housed in DD.
The depolarization of a presynaptic terminal releases GABA into the synaptic cleft where it is estimated to reach a concentration of 1.5–3 mM. High affinity GABA transporters (GAT) rapidly remove GABA from the synaptic cleft with an estimated time constant of 75–125 μs (Jones et al. 2001; Mozrzymas et al. 2003). Four high affinity GABA transporters (GAT-1, GAT-2, GAT-3, BGT-1) have been identified with GAT-1 and GAT-3 being responsible for terminating GABA signaling in the brain (Dalby 2003). GATs are secondary active transporters that utilize the Na+ and Cl− electrochemical gradients to move 2 Na+ and 1 Cl− ion for each GABA molecule transported (Kavanaugh et al. 1992). This stoichiometry results in a lower limit (~400 nM) below which GAT cannot reduce extracellular GABA (Attwell and Mobbs 1994; Cavelier et al. 2005). During prolonged or high-frequency GABA release, the transporter’s capacity is exceeded, and GABA diffuses out of the synapse and activates extrasynaptic receptors (Isaacson et al. 1993; Dalby 2003; Semyanov et al. 2003; Glykys and Mody 2007). GABA that “spills over” from the synapse may also activate GABAB receptors and inhibit the release of glutamate from terminals of the retinohypothalamic tract (Moldavan and Allen 2013). Under appropriate conditions, GATs may act in reverse to move GABA from the cell cytoplasm into the extracellular space (Richerson and Wu 2003). Evidence for GAT-mediated GABA release has not been reported in the SCN.
8.2.2 GABAA Receptors
Neurotransmission at GABAergic synapses is important in the SCN neural network. GABA is the major neurotransmitter at intra-SCN synapses, and nearly all neurons in the SCN contain glutamic acid decarboxylase, the GABA-synthesizing enzyme (Van den Pol 1980; Belenky et al. 2003). The SCN is estimated to contain between 8,000 and 12,000 neurons. Each SCN neuron receives approximately 1,400 synaptic contacts located on the soma and dendrites (Guldner 1984). Approximately half of these synaptic connections stain positive for markers of GABAergic neurotransmission (Decavel and Van den Pol 1990; Belenky et al. 2008). SCN neurons have short axons that project to other SCN neurons as well as to neighboring hypothalamic nuclei (Van den Pol 1980; Strecker et al. 1997). SCN neurons communicate with each other via GABAA receptor-mediated neurotransmission (Strecker et al. 1997).
GABA activates ionotropic GABAA receptors and metabotropic GABAB receptors. GABAA receptors have a pentameric structure that forms an agonist gated Cl− channel. The activation of GABAA receptors in the adult brain has traditionally been thought to be inhibitory due to the activation of a Cl− conductance that hyperpolarizes the membrane. The activation of GABAA receptors may also produce shunting inhibition that results from an increase in membrane conductance that shunts depolarizing currents (Staley and Mody 1992). SCN neurons are a rare example of adult neurons in which GABA acts as both an inhibitory and excitatory neurotransmitter (Wagner et al. 1997, 2001; De Jeu and Pennartz 2002; Choi et al. 2008; Irwin and Allen 2009). In contrast, GABAB receptors are composed of seven transmembrane spanning subunits that signal through Gi-type G-proteins and in the SCN regulate transmitter release from presynaptic retinohypothalamic tract terminals by inhibiting voltage-gated Ca2+ channels and activating potassium currents in SCN neurons (Jiang et al. 1995; Moldavan et al. 2006; Moldavan and Allen 2013).
8.2.2.1 GABAA Receptor Structure
GABAA receptors have diverse physiological properties and sensitivity to pharmacological agents—properties conferred by the specific subunits that make up the GABAA receptor complex (Rudolph and Mohler 2004). Individual neurons express multiple types of GABAA receptors, and this diversity of GABA-mediated signaling is essential for rhythmicity and synchrony in neural networks (Aradi et al. 2002; Aradi and Soltesz 2002). Eighteen GABAA receptor subunits have been identified including α(1–6), β(1–3), γ(1–3), ρ(1–3), δ, ε, and π subunits (Barnard et al. 1998). GABAA receptors are composed of five subunits—two α subunits, two β subunits, and a γ subunit (Fig. 8.2). In some GABAA receptors, the γ subunit is replaced with a δ, ε, ρ (1–3), or π subunit (Barnard et al. 1998).
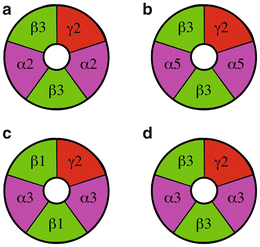
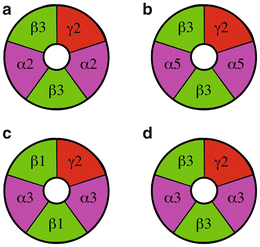
Fig. 8.2
Putative subunit composition of GABAA receptors in the SCN. The structure of GABAA receptors in the SCN can be predicted based on the subunit composition of GABAA receptors in other parts of the brain. (a) Synaptic receptors. (b) Extrasynaptic receptors. (c & d) Receptors located both synaptically and extrasynaptically
In the SCN, three α (α2, α3, and α5), two β (β1 and β3), and two γ (γ1 and γ2) GABAA receptor subunits have been identified using in situ hybridization and immunocytochemical techniques (Gao et al. 1995; O’Hara et al. 1995; Naum et al. 2001; Belenky et al. 2003; Jobst et al. 2004). β2/3 immunoreactivity was observed in the ventrolateral SCN of the hamster but not the rat (Gao et al. 1995; Naum et al. 2001; Jobst et al. 2004). β2/3 subunit messenger RNA was detected in the SCN of mice, but a concern was raised that this signal may result from the inclusion of additional hypothalamic tissue in the sample (Gao et al. 1995; O’Hara et al. 1995). Therefore, the difference in β2/3 expression may be a species difference (hamster and mouse versus rat). Messenger RNA for β1 subunit was identified in the mouse and hamster SCN (O’Hara et al. 1995; Naum et al. 2001). The β1 subunit in the SCN of Syrian hamsters housed in a light–dark cycle (14:10) had a circadian and diurnal rhythm with a peak amplitude at midnight and a nadir at midday. In contrast, the α2, α5, and β5 subunits were not rhythmic (Naum et al. 2001).
While there are several hundred possible GABAA receptor subunit combinations, only a limited number of subunit combinations are actually expressed in neurons (Barnard et al. 1998; Fritschy and Brunig 2003; Mody and Pearce 2004; Mohler 2006; Olsen and Sieghart 2008). Based on the pattern of GABAA receptor expression in other brain areas, we can surmise the subunit composition of GABAA receptors expressed by SCN neurons. First, GABAA receptors consisting of α2β3γ2 subunits are probably expressed at GABAergic synapses since these receptors are mainly synaptic in the hippocampus (O’Hara et al. 1995; Naum et al. 2001). Second, a likely GABAA subunit combination is α3β1γ2 or α3β3γ2, which may be located synaptically or extrasynaptically (O’Hara et al. 1995). Third, the GABAA receptor combination α5β3γ2 is likely to be located extrasynaptically since α5-containing receptors are found at extrasynaptic locations. Interestingly, the predominant GABAA receptor in the brain, α1β2γ2, does not appear to be present in the SCN since the α1 subunit is not expressed in hamster, rat, or mouse SCN (Gao et al. 1995; O’Hara et al. 1995; Jobst et al. 2004). Although δ subunits have not been identified with neurochemical techniques, there are pharmacological data that suggest that δ-containing GABA receptors are located in the SCN (Ehlen and Paul 2009; McElroy et al. 2009).
The subunit composition of GABAA receptors determines their activation, inactivation, and desensitization kinetics, as well as their affinity for GABA and consequently the strength of GABAA-mediated synaptic currents (Schofield and Huguenard 2007). Synaptic receptors typically have lower agonist affinities and desensitize more rapidly than extrasynaptic receptors (Mody and Pearce 2004; Mody 2005; Mohler 2006). For example, α2-containing GABAA receptors, which are generally localized to synapses, have a lower affinity for GABA and desensitize faster than extrasynaptic GABAA receptors containing the α5 subunit (Mody 2001; Semyanov et al. 2004). The high GABA affinity allows extrasynaptic GABAA receptors to respond to the low GABA concentrations (0.3–1 μM) present in the extrasynaptic space (Lerma et al. 1986; Tossman et al. 1986). The extremely slow rate of desensitization displayed by these extrasynaptic receptors underlies the continuous activation necessary for the GABA-mediated tonic current, which is observed in many neurons (Stell and Mody 2002).
The subunit composition of the GABAA receptors also determines receptor’s sensitivity to specific pharmacological agents. Several pharmacological agents that affect circadian phase also interact with GABAA receptors in a subunit-specific manner. The benzodiazepine, diazepam, which alters circadian period and blocks light-induced phase shifts, increases GABAA-mediated currents through receptor channels composed of γ2 subunits together with α1, α2, α3, or α5 subunits (Ralph and Menaker 1986; Subramanian and Subbaraj 1996). The imidazobenzodiazepine L655,708 has an affinity for GABAA receptors containing α5 subunits 50 times higher than GABAA receptors containing other α subunits (Ing and Poulter 2007). L655,708 will potentiate, at low concentrations, currents mediated by GABAA receptors containing the α5 subunit (Ing and Poulter 2007). L655,708 acting in the SCN to increase a tonic GABA current could be used to study the role of extrasynaptic GABAA receptors in the SCN network.
8.2.2.2 GABAA Receptor-Mediated Neurotransmission
GABA binding to the GABAA receptor results in a conformational change that opens an anion selective channel. GABAA receptors in the SCN are primarily permeable to Cl− (~80 %) although there is also a significant HCO3 − conductance (~20 %) (Wagner et al. 2001). For this reason, GABAA receptor-mediated current amplitude and direction depend on the chemical and electrical gradients of the Cl− ion. In most adult neurons, the intracellular Cl− concentration is low compared to the extracellular Cl− concentration. Therefore, the equilibrium potential for Cl− (E Cl) is more negative than the resting membrane potential, and GABAA receptor-mediated currents are consequently hyperpolarizing (inhibitory). However, if the intracellular Cl− concentration is high, E Cl may became more positive than the resting membrane potential, and GABA efflux will depolarize the cell membrane causing neuronal excitation (Woodin et al. 2003; Fiumelli et al. 2005).
Depending on the circadian time, GABAA receptor-mediated neurotransmission in the adult SCN can be both excitatory and inhibitory. GABA was first reported to increase the firing rate of SCN neurons during the day and inhibit SCN neurons during the night (Wagner et al. 1997). This observation was not absolute—the majority (~75 %) of SCN neurons were activated during the day compared to only 32 % at night. This circadian control of excitability was attributed to a circadian regulation of the intracellular Cl− concentration (Wagner et al. 1997). Subsequent research confirmed GABA’s dualistic role as both an inhibitory and excitatory neurotransmitter in the SCN; however, in these studies GABA was more likely to be an excitatory neurotransmitter during the night than during the day (De Jeu and Pennartz 2002; Choi et al. 2008; Irwin and Allen 2009). These inconsistencies have not been explained but may be due to differences in methodology: whole cell vs. perforated patch recording. Also, when extracellular recording electrodes are used to record the action potential firing rate, a shunting effect of GABAA receptor activation could mask a relatively depolarized E Cl. Further, all of these interpretations are complicated by differences in solutions and the fact that traumatized, damaged, and ischemic neurons have all been shown to display depolarized E Cl. Furthermore, whether or not GABA is excitatory or inhibitory or evokes no response ultimately depends on the cell’s resting membrane potential. Regardless of the differences in experimental observations, it is clear that a substantial proportion of SCN neurons respond to GABA in an excitatory manner and that the proportion of SCN neurons that are excited by GABA shows a circadian or diurnal rhythm.
In neurons, the basal intracellular Cl− concentration is set by the combined activity of several chloride channels and transporters (Fig. 8.3, however see Glykys et al. 2014). Of these, the sodium–potassium–chloride cotransporter 1 (NKCC1) has received the most attention for maintaining Cl− influx. Under normal neuronal conditions, NKCC1 moves one Na+ and one K+ ion into the neuron for every two Cl− ions. NKCC1 was found in the soma and dendrites of virtually every SCN neuron examined (Belenky et al. 2010). Further, a circadian rhythm of NKCC1 protein level was observed in the dorsal SCN, but not in the ventral SCN (Choi et al. 2008). Blocking NKCC1 with bumetanide reduces or eliminates depolarizing GABA responses and increases the magnitude of inhibitory GABA responses (Irwin and Allen 2009). These data demonstrate that in the absence of NKCC1-mediated Cl− influx, the intracellular Cl− concentration will decrease, resulting in larger inhibitory GABA responses (Choi et al. 2008; Irwin and Allen 2009).
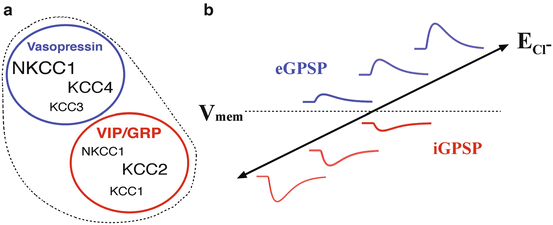
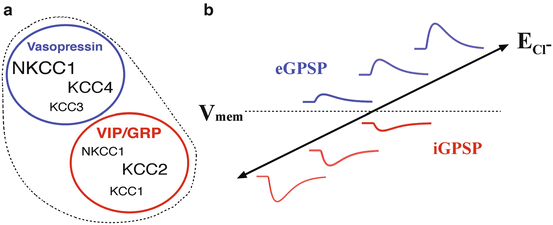
Fig. 8.3
Regulation of the intracellular Cl− gradient in SCN neurons. (a) Diagram showing the relative expression of Cl− cotransporters in SCN neurons expressing different neuropeptides (Belenky et al. 2010). (b) Schematic displaying the relationship between the Cl− equilibrium potential (E Cl), membrane potential (V mem), and the magnitude and the direction of GABA-induced changes in membrane potential. An increase in Cl− influx will increase [Cl−]i and make E Cl more positive, while Cl− efflux will decrease [Cl−]i and produce a more negative E Cl. When E Cl is more negative than V mem, GABA hyperpolarizes the membrane potential (inhibitory GABA-mediated postsynaptic potential, iGPSP). An E Cl more positive than V mem depolarizes the membrane potential (excitatory GABA-mediated postsynaptic potential, eGPSP)
Chloride efflux is mediated by the members of the potassium–chloride cotransporter family (KCC1–4), which move one K+ and one Cl− out of the neuron for each cycle. The four members of the KCC family are regionally and phenotypically distributed in the SCN. KCC3 and KCC4 are expressed in vasopressin-expressing SCN neurons, while KCC1 and KCC2 are expressed in vasoactive intestinal peptide (VIP)- and gastrin-releasing peptide (GRP)-expressing SCN neurons (Belenky et al. 2008; Belenky et al. 2010). KCC1, KCC3, and KCC4 all have a lower affinity (15–20 mM) for Cl− than KCC2 (5–7 mM). Due to its higher affinity for Cl−, KCC2 is expected to transport Cl− out of a neuron at lower Cl− concentrations compared to the other KCCs. Given the different Cl− affinities of the various KCC cotransporters, it is expected that the intracellular Cl− concentration should reach lower concentrations in cells expressing KCC2 than in the neurons expressing KCC3 and KCC4. Consequently, the lower intracellular Cl− concentration in KCC2-expressing neurons would engender more hyperpolarizing GABA responses. The overall balance between NKCC1 (Cl− influx) and KCC2 (Cl− efflux) expression and activity in SCN neurons helps determine the intracellular Cl− concentration and thereby enable both depolarizing and hyperpolarizing GABA responses.
8.3 Circadian Regulation of GABAergic Neurotransmission
Functionally, GABAergic neurotransmission in the SCN has been proposed to modulate retinal input to the SCN (Jiang et al. 1995; Moldavan et al. 2006; Moldavan and Allen 2013), synchronize short-term firing patterns between SCN neurons (Kononenko and Dudek 2004), synchronize circadian firing patterns (Liu and Reppert 2000; Shirakawa et al. 2000), and regulate the amplitude of circadian rhythms (Wagner et al. 2001; Aton et al. 2006; Choi et al. 2008). Spontaneous and miniature GABAA receptor-mediated currents are observed in the majority of SCN neurons (Kim and Dudek 1992; Jiang et al. 1997; Strecker et al. 1997; Itri and Colwell 2003; Gompf and Allen 2004; Itri et al. 2004; Gompf et al. 2006). The frequency of the spontaneous fast synaptic GABA currents shows a day–night difference in the dorsal but not the ventral SCN (Itri and Colwell 2003; Itri et al. 2004). The frequency of the GABAA receptor-mediated currents peaks late in the subjective day and early in the subjective night (Itri and Colwell 2003; Itri et al. 2004). The increased GABAergic activity precedes the peak in extracellular GABA concentration, which occurs early in the night (Aguilar-Roblero et al. 1993). The application of GABA or the GABAA agonist muscimol can elevate or reduce the intracellular calcium concentration in different populations of SCN neurons, while the GABAB agonist baclofen lowers intracellular calcium in both groups (Irwin and Allen 2009). Further, the application of gabazine, a GABAA receptor antagonist, altered the resting intracellular calcium concentration inversely to the direction induced by GABA (Irwin and Allen 2009). The strength of GABAA receptor-mediated synaptic transmission is regulated in a diurnal manner (Gompf and Allen 2004). Short-term synaptic depression exhibits a diurnal rhythm, with a majority of synapses during the day and few synapses at night showing synaptic depression, implying that neurotransmission within this local circuitry is under the control of the circadian clock. The spontaneous daytime action potential firing frequencies observed in the SCN correspond to the frequencies that elicit synaptic depression at synapses between SCN neurons (Gompf and Allen 2004). Therefore, both the frequency and synaptic strength of intra-SCN GABAergic neurotransmission may signal a cell’s relative circadian phase to neighboring neurons.
Albus et al. (2005 ) found two peaks in the ensemble firing pattern of the SCN on the first day following a 6-h phase delay. They demonstrated that the peaks could be separated into an early component intrinsic to the dorsal SCN and a late component intrinsic to the ventral SCN. Combined with findings from previous studies, these results were interpreted as evidence that (1) the two peaks reflect two pacemakers, the ventral SCN which shifts more rapidly than the dorsal SCN, (2) the ventral SCN has a strong phase-shifting effect on the dorsal SCN, and (3) GABA acting through GABAA receptors plays a critical role in synchronizing these regional oscillators, perhaps by exciting the dorsal SCN at night and inhibiting the ventral SCN during the day. Aton et al. (2006) found that blockade of GABAA receptors for up to 10 days increased the cell-to-cell synchrony and individual cell amplitude of circadian rhythms in cultured SCN. These results were interpreted as GABA is (1) not required in synchronizing daily rhythms in the SCN and (2) restricts the amplitude of circadian rhythms in firing rate.
One possible union of these data recognizes the antagonistic role of GABA in the SCN as it adjusts to large changes in ambient light cycles (e.g., transmeridian travel or perhaps seasonal) or to natural variability in the cycle-to-cycle synchrony of SCN neurons. Following a large delay in the light cycle as in Albus et al. (2005), GABA slows the shifting of neurons to local time. Addition of bicuculline (or cutting a large portion of the GABA projections) would release this antagonism so that cells in the ventral SCN would shift rapidly to the new local time. It could be that neurons in the dorsal SCN shift slowly under these conditions because GABA is excitatory instead of inhibitory, but single-cell data are needed. During stable entrainment or free-running conditions, the loss of GABA’s antagonism would lead to a tighter phase distribution of cells within the SCN and less cycle-to-cycle variability. Further studies are also required to distinguish the role of fast synaptic vs. tonic GABAA receptor-mediated neurotransmission.
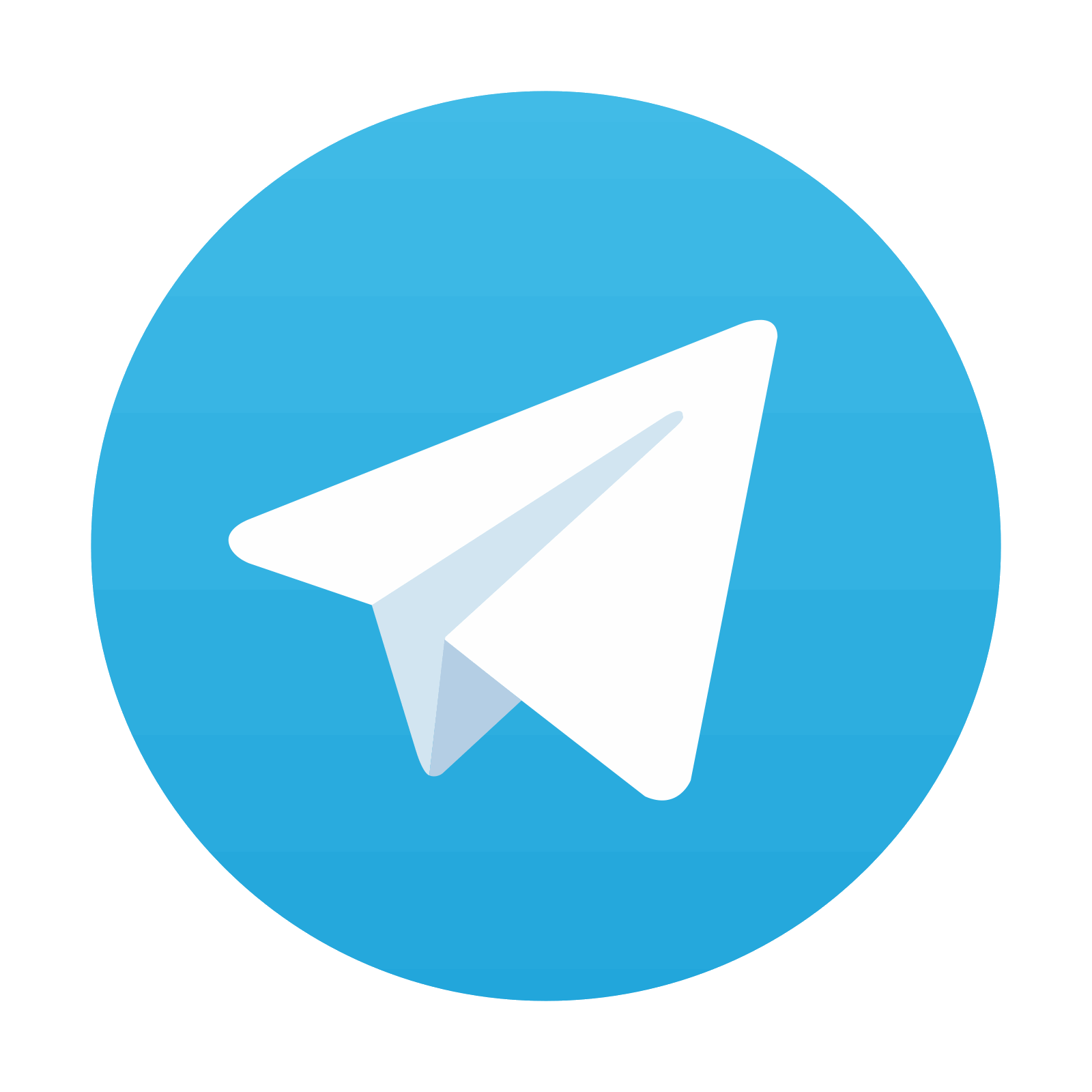
Stay updated, free articles. Join our Telegram channel
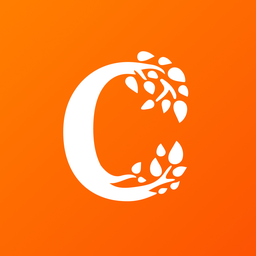
Full access? Get Clinical Tree
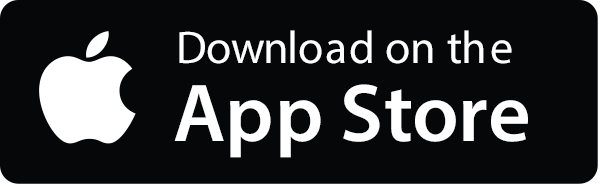
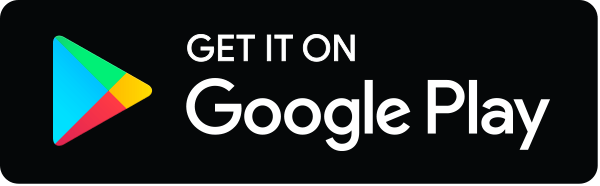