5 Fundamental and Practical Aspects of Semen Cryopreservation William V. Holt1* and Linda M. Penfold2 Following the discovery in the middle of the last century that glycerol can be used successfully as a cryoprotectant (Polge et al., 1949), thus allowing spermatozoa to be stored for months, years or decades, many aspects of reproductive technology were revolutionized. The most obvious results of this revolutionary discovery include the massive development of selective breeding in agriculture, whereby semen from desirable bulls can be frozen, stored and shipped within a single country or exported around the world, and then used for artificial insemination (AI). While the dairy industry has been a major global beneficiary of this technology, similar benefits have been derived for human infertility treatment, for which semen from fertile men is banked and used for donor insemination programmes both at home and abroad. Other applications of semen freezing technology have also developed in a variety of different contexts, including: (i) the cryopreservation of genetic resources for food fishes (Solar, 2009) and fishes used in biomedical research (i.e. zebra fish and medaka) (Yang and Tiersch, 2009; Tiersch et al., 2011); (ii) the storage of mouse, rat and primate spermatozoa for biomedical research purposes (Shaw and Nakagata, 2002; Mazur et al., 2008); (iii) the establishment of semen banks for the preservation of regional and national agricultural breeds (Woelders et al., 2006); and (iv) the establishment of semen banks for the support of conservation breeding programmes (Holt et al., 1996; Wildt et al., 1997; Watson and Holt, 2001; Bartels and Kotze, 2006). A number of important reviews have been written about semen cryopreservation, both with focus on a particular species, such as sheep (Salamon and Maxwell, 1995a,b), humans (Royère et al., 1996), dogs (Pena et al., 2006) or mice (Marschall and Hrabe de Angelis, 1999), and with a more general approach (Watson, 1979, 1990; Holt, 2000a,b; Bailey et al., 2003). This list is not exhaustive, but it is surprising that most of the reviews of semen cryopreservation were published more than a decade ago; more recent reviews include those by Chen and Liu (2007), Curry (2007) and Dinnyes et al. (2007). Watson’s review in the 1990 edition of Marshall’s Physiology of Reproduction (Watson, 1990) is particularly interesting for its detailed account of the history of semen collection and preservation. He recounts some of the earliest scientific investigations into semen freezing and artificial insemination, which were documented in the 18th and 19th centuries. These include, notably: the observations of Lazzaro Spallanzani (1776), an Italian priest, who described the first successful AI procedure in a dog, and also noted that frog, human and stallion semen could be cooled in snow and their motility restored by rewarming; and Paolo Mantegazza (Mantegazza, 1866), who described the effects of temperature changes on human sperm motility. Mantegazza also forecast correctly that one day in the future it would be possible to collect and store semen from soldiers who died in battle, so that it could be used posthumously to inseminate their wives. This procedure has actually been used on a few occasions over the last 20 years, when it has provoked vigorous ethical debates and outright bans in some countries (for a commentary, see Nakhuda, 2010). A great deal has been written about the general theory of cryobiology, especially as the discipline involves a diversity of species and taxonomic groups that include plants (seeds, meristems, etc), bacteria, fungi, as well as a diversity of animal cells and tissues. The general principles governing our understanding of cell survival during freezing and thawing rely on knowledge of water transport through membranes and interactions between solutes, cryoprotectants, low temperature and membranes. As shown in Fig. 5.1, when the temperature falls, the water in the sample actually remains liquid unless active steps (seeding) are taken to induce ice crystallization; the solution at this stage is said to undergo ‘supercooling’ (Fig. 5.1: step 1). Ice formation occurs randomly at some point below the freezing point; the randomness of this stage is dictated by the probability that groups of water molecules organize themselves into clusters and initiate a chain reaction. As this is an exothermic reaction, sufficient heat is released to increase the sample temperature to a significant extent (Fig. 5.1: step 2). Depending on the sample being frozen, and the technique being used to cool it, the sample temperature remains static for a few minutes before cooling is resumed (Fig. 5.1: step 3) or can even result in a transient increase in temperature before cooling resumes. These temperature excursions invariably occur when (plastic) straws of semen are frozen in cold nitrogen vapour (for instance, a typical technique is to place the straw horizontally about 4 cm above the surface of liquid nitrogen), and researchers have been divided as to whether this is a harmful process. To address this issue, directional freezing units that promote seeding of the sample in a linear fashion are thought to minimize these temperature excursions. When ice begins to form during the cooling process, the dissolved salts and other compounds are excluded from the ice and become concentrated into the ever-decreasing spaces between the developing ice crystals. Biological cells interact osmotically with these unfrozen pockets of high solute concentration and water tends to be drawn out of the cells, resulting in cell shrinkage. This process results in two opposing deleterious effects that counteract each other: if the cooling rate is slow, the cell membranes are exposed for longer periods to the pockets of hypertonic solutions, with possible deleterious effects such as protein and lipid extraction and the generation of reactive oxygen species (ROS); conversely, if the cells are cooled too rapidly their cytoplasmic water content remains high and they run the risk of lethal intracellular ice formation. In practical terms, the optimal cooling rate has to be regarded as a compromise between these opposing effects. Experimentally it is possible to illustrate some of these effects by setting up idealized conditions using a cryomicroscope. Figure 5.2a shows what happens if some cryopreservation medium lacking cryoprotectant is spiked with a fluorescent dye and frozen at a slow cooling rate (about 5°C/min) to –20°C. The bright lines in the image represent inter-ice crystal regions where the fluorescent dye has become highly concentrated. If cryoprotectant has been added, or if a faster cooling rate had been used, the structure would have been more complex. Figure 5.2b shows a snapshot taken as the ice crystallization front was moving across the field of view (left to right). A number of spermatozoa can be seen near the leading edge of the ice front and can later be seen distributed across different regions of the ice crystal formations (Fig. 5.2c). Figure 5.2d shows a snapshot taken as the sample is being thawed; it is notable that the heterogeneity displayed while the sample is frozen is still evident at the time of thawing. These observations indicate that individual spermatozoa interact with both ice crystal regions and the interspersed regions of high salt content, and that this level of heterogeneous distribution is established around the time of freezing and then maintained until the sample is thawed. The photographs in Fig. 5.2 were set up to exaggerate the degree of organization visible within the preparations; however, a number of ultrastructural studies have confirmed that spermatozoa within frozen straws of semen become partitioned within regions of high solute concentration, and that they are separated by regions of pure ice (Courtens and Paquignon, 1985; Ekwall et al., 2007). In addition to the purely osmotic interactions associated with the freezing and thawing processes, the cell membrane lipids undergo partially irreversible phase transitions (Holt and North, 1984), with the appearance of semi-crystalline lipid arrays as the temperature declines during cooling and freezing. This effect is thought to be responsible for the damaging effects of ‘cold shock’, which occurs when spermatozoa are rapidly cooled in the absence of protective additives (Quinn and White, 1966; Quinn et al., 1969; Moran et al., 1992); cold shock is not only recognizable because of the structural damage caused to the plasma and acrosomal membranes, but is also known to induce loss of cellular homeostasis through inappropriate membrane permeabilization, excessive uptake of calcium and uncontrolled loss of potassium. In keeping with the long-established fluid mosaic model of membrane structure and organization (Singer and Nicolson, 1972), sperm plasma membrane lipids have long been envisaged as being free to diffuse laterally within the two-dimensional plane of the sperm surface (Wolf and Voglmayr, 1984). This broad view, although a useful conceptual model for thinking about cryopreservation, has been modified somewhat with the realization that membrane lipids and proteins are highly organized into structurally, biochemically and functionally defined regions, or domains and subdomains, within the plane of the membrane (for reviews, see Zitranski et al., 2010 and Zhu and Inaba, 2011). If anything, gaining further insights into sperm membrane domain organization has strengthened the realization that cryopreservation technology has the potential to cause huge amounts of cellular dysfunction through inappropriate and disruptive phase transitions. Unfortunately, changes in temperature are not the only cause of membrane lipidphase transitions; they can also be induced by the removal of water, such as occurs during the osmotic fluxes associated with the freezing process or during freeze-drying (Chiantia et al., 2005; Bennun et al., 2008). The occurrence and effects of these drying-induced phase transitions can be offset to some extent by certain sugars, especially trehalose (Rudolph et al., 1986; Chandrasekhar and Gaber, 1988; Crowe et al., 1988). In fact, some authors have reported that the presence of trehalose is beneficial during the cryopreservation of spermatozoa (see, for example, Hu et al., 2010b). In practice, it is not possible to cryopreserve spermatozoa, and most other cell types, without the presence of cryoprotectants. These chemicals, which belong to several distinct groups of compounds, interact with water molecules and inhibit the formation of the hydrogen bonds that are essential for the formation of ice crystals. The theory of cryoprotectant action is highly complex and multifactorial, but essentially it is understood that these compounds lower the freezing point of water and, thereby, at any given temperature, reduce the extent of ice crystal formation. This has the complementary effect of reducing the formation of unfrozen pockets of high solute concentration and, importantly, permitting these hyperosmotic regions to undergo transition to the glassy phase without necessarily reaching the same high solute concentrations (for a comprehensive review of cryoprotectant action, see Fuller, 2004). The effectiveness of cryoprotectants varies between cell types, partly because they operate via different mechanisms, but also because they can be differentially toxic to cells. This introduces more variables that need to be considered when examining the way that cryoprotectants work. Even a simple compound such as glycerol has been found to induce sterility in poultry spermatozoa (Hammerstedt and Graham, 1992), where the effects may be due as much to direct alterations of membrane and cytoplasmic structures as to purely anisosmotic damage; it can also cause acrosomal damage in boar spermatozoa if used at concentrations exceeding 3% (w/v) (Hofmo and Almlid, 1991; Gutierrez-Perez et al., 2009). The permeability of the cellular plasma membrane to both water and cryoprotectants is an important variable that governs the optimal design of protocols for cell freezing as well. Some cells behave as perfect osmometers when confronted with solutions of different tonicity and will shrink or expand as appropriate, in ways that can be predicted from biophysical principles. When their surrounding media begin to freeze, with the formation of a hyperosmotic external environment, these cells begin to shrink as their intracellular water is drawn across the membrane. The ideal cooling rates for these cells would be sufficiently fast to minimize exposure to the deleterious effects of unfrozen, extracellular, hypertonic solutions, but not too fast, otherwise the excess water remaining inside the cells would turn into ice crystals, with lethal effects. The optimal cooling rate is indeed predictable for certain, regularly shaped cell types, such as mammalian oocytes, for which measurements of membrane permeability to water and to cryoprotectant can be made. Spermatozoa are not ideal candidates for modelling their optimal cooling rates because their high degree of structural and functional differentiation has resulted in irregularly shaped cells whose volume is difficult to calculate. A number of researchers have, nevertheless, attempted to measure sperm membrane permeability and predict optimal cooling rates (Gao et al., 1992, 1993; Noiles et al., 1993), but until about 10 years ago these predictions were wildly inaccurate. More accurate predictions have now been obtained for several mammalian and non-mammalian species by applying differential scanning calorimetry and measuring sperm membrane permeability to water and cryoprotectants at sub-zero temperatures, instead of supra-zero temperatures, which would not necessarily be relevant (Devireddy et al., 1999, 2000, 2004, 2006; Thirumala et al., 2006; Alapati et al., 2009; Hagiwara et al., 2009). Fourier-transform infrared spectroscopy (FTIR) is an alternative biophysical approach to the investigation of sperm membrane phase transitions during cooling, and has been used successfully to investigate cold shock (Drobnis et al., 1993) and freezing (Ricker et al., 2006; Oldenhof et al., 2010). This technique is extremely sensitive to lipid conformational order (Mendelsohn and Moore, 1998) and allows the direct measurement of changes in the CH2 symmetric stretching frequency (which reflects lipid acyl chain conformation) over a range of temperatures, even in the presence of cryoprotectants and cryoprotective additives. Two recent papers about the cryopreservation of stallion spermatozoa are of considerable interest here: Oldenhof et al. (2010) were able to determine the membrane permeability parameters necessary for modelling the optimal cooling and freezing rates for individual animals, and showed that they were not all the same; and Ricker et al. (2006) showed that the presence of egg yolk lipoproteins during freezing prevented serious changes in phase transition behaviour that were seen in spermatozoa frozen and thawed in the absence of egg yolk. Traditionally, sperm cryopreservation diluents have included various sources of lipid and proteins (e.g. egg yolk, milk proteins, coconut milk, soybean lecithin), as well as sugars such as sucrose and trehalose, to assist with cryoprotection. There have been numerous publications over the last several decades dealing with the merits of various diluent additives as they apply to individual species, and the reader is referred to other publications for further detailed information (Watson, 1979, 1981, 1990; Watson and Holt, 2001). Unfortunately, despite the large amount of research that has been invested in improving the performance of semen cryodiluents, it is clear that the mechanism of action of these additives still remains obscure, which makes it difficult to exploit them further and gain more improvement. For example, the active ingredient of egg yolk was narrowed down to a low-density lipoprotein component (LDL) in the 1970s (Watson, 1976), but even though the use of LDL has recently seen a resurgence as an effective way to enhance semen preservation in several species (Bencharif et al., 2010; Hu et al., 2010a, 2011; Dong et al., 2011; Vera-Munoz et al., 2011), its mode of action is still largely unexplained at the level of the sperm plasma membrane. The protective effects of exogenous lipids during the cooling and freezing of spermatozoa have been recognized for many years (Evans and Setchell, 1978; Graham and Foote, 1987; Holt and North, 1988) and substances such as lecithin (phosphatidylcholine), often of plant origin, form the basis of several commercial cryopreservation media. However, the mechanisms underlying these effects have been difficult to understand, especially as there has been no evidence that the lecithin intercalates into the sperm plasma membrane, with resultant modulations of phase transitions. FTIR studies (Ricker et al., 2006) have, nevertheless, revealed that the presence of soy- or egg yolk-derived lecithin during sperm cryopreservation prevents the deleterious phase transitions that are suffered when spermatozoa are frozen–thawed without the presence of lecithin, and that the lecithin becomes tightly associated with the sperm plasma membrane. These authors also believed that the presence of lecithin as a cryoprotective additive was equally as effective as that of egg yolk lipoprotein for the cryopreservation of stallion spermatozoa. This is consistent with an earlier study (Kolossa and Seibert, 1990), in which the authors developed a chemically defined cryoprotective medium for bovine spermatozoa that had commercial lecithin as the main ingredient, and claimed that it was only slightly less effective than egg yolk-based diluents. These results are especially topical in view of the upsurge of interest in avoiding animal products in semen diluents for the sake of enhanced biosecurity. Media for semen cryopreservation often contain Equex, an anionic surfactant (sodium triethanolamine lauryl sulfate – SLTS, also known as Orvus ES) (Howard et al., 1986; Pontbriand et al., 1989; Kaplan and Mead 1992; Montfort et al., 1993). This compound is usually used in combination with egg yolk and is thought to emulsify the egg yolk, possibly assisting in its ability to interact with the plasma membrane. It is especially prevalent in diluents for boar semen, but is hardly ever used for bull semen; it has also been tested in a number of wild species, including the African elephant (Howard et al., 1986), and in the domestic dog (Rota et al., 1998). It would be of interest to investigate SLTS in more detail, possibly using FTIR, to find out more about its mechanism of action. Interest in cryopreserving mouse spermatozoa from defined genetic lines for biomedical research produced significant advances in appropriate technology in the early 1990s (George et al., 1992; Nakagata and Takeshima 1992; Nakagata et al., 1992; Sztein et al., 1992), and it is interesting that the more successful approaches often employed 18% raffinose, together with skimmed milk. Differences in the post-thaw fertility (from in vitro fertilization or tubal insemination) of spermatozoa of various mouse strains have, none the less, remained a problem (e.g. C57BL/6J mice show a 0–20% fertilization rate). Recent advances have shown that supplementing the 18% raffinose medium with L-glutamine and methyl-β-cyclodextrin enormously improves the fertilization rate for this specific mouse strain (bringing it to 69.2%; Takeo and Nakagata, 2010). A similarly dramatic improvement in fertilization rate has also been reported following supplementation of the raffinose medium with the reducing agent monothioglycerol (Ostermeier et al., 2008; Takahashi and Liu, 2010); these authors reported that the fertilization rates of several mouse genetic lines, including C57BL/6, were restored almost to the same level as those of fresh spermatozoa. The recent findings that cyclodextrin can improve the cryosurvival of mouse spermatozoa are mirrored to some extent by equivalent findings with bull, ram, stallion and goat spermatozoa (Mocé et al., 2010a,b; Moraes et al., 2010; Oliveira et al., 2010; Spizziri et al., 2010). Here, the cyclodextrin is used to load the sperm plasma membrane with cholesterol before cryopreservation. This effectively chan ges the membrane lipid composition and hence the phase transition behaviour during cooling and freezing. To our knowledge, monothioglycerol has not been tested with domestic livestock, but if it improves cryosurvival by reducing oxidative damage associated with freezing and thawing, it is likely to be successful to some extent. Other thiols have been tested with bull semen (cysteine, N-acetyl-L-cysteine and 2-mercaptoethanol; Bilodeau et al., 2001) and at concentrations above 0.5 mM have been shown to have some beneficial effects on post-thaw motility. There are many other studies reporting the inclusion of other antioxidants in semen extenders (liquid diluent added to semen to preserve its fertilizing ability) and cryoprotective media (Pena et al., 2004; Funahashi and Sano, 2005; Gadea et al., 2005; Pagl et al., 2006), but none has been demonstrated to provide beneficial effects matching those seen by adding monothioglycerol to the mouse spermatozoa. While conventional freezing requires the use of cryoprotectants to prevent damage caused by ice crystal formation, the use of increased cryoprotectant concentrations (up to 6 M) and rapid cooling allows solutions to be cooled to extremely low temperatures without any ice being formed at all. This process is termed vitrification; the name refers to the fact that the solution undergoes a glass transition and forms a stable structure without the presence of ice crystals. Cells that have been vitrified in this way can be returned to ambient temperature without the distortion that normally accompanies conventional freezing. There are, however, a few drawbacks to this technique; the ‘glassy’ state of vitrified samples tends to be rather fragile and easily damaged but, more importantly, there is a high risk of ice crystal formation during the process of rewarming. Vitrification techniques have been applied to mammalian oocytes (Shaw et al., 1992; Succu et al., 2008) and embryos (Rall and Fahy, 1985; Rall and Wood, 1994), but success with spermatozoa has been very limited until quite recently. One report (Isachenko et al., 2008) showed that if small (30 µl) droplets of human spermatozoa, suspended in media consisting of human tubal fluid supplemented with 1% human serum and 0.25 M sucrose, were dropped directly into liquid nitrogen; on warming they recovered about 65% progressive motility and retained mitochondrial function. Similar results were also obtained with rainbow trout spermatozoa when 20 µl droplets of spermatozoa, suspended in a mixture containing a standard buffer for fish spermatozoa (Cortland medium), supplemented with combinations of 40% seminal plasma, 1% bovine serum albumin and 0.125 M sucrose, were plunged directly into liquid nitrogen (Merino et al., 2011); these authors reported the recovery of >80% motility upon rewarming and the retention of mitochondrial function. These results are encouraging because they suggest that alternative approaches to semen cryopreservation are worth investigating. The limitation on droplet size may not turn out to be a serious problem if suitable methods for delivering large numbers of droplets into liquid nitrogen could be found. The more serious downside of this approach might be the high risks of microbial contamination through the liquid nitrogen itself. The research group that has been developing these approaches has evaluated several methods (cryoloops, droplets, open pulled straws – OPS, and open straws) to avoid risks of contamination via liquid nitrogen, and have found that only the OPS method can be considered to be a ‘clean’ technique (Isachenko et al., 2005a). The advent of intracytoplasmic sperm injection (ICSI) technology, whereby individual spermatozoa are microinjected into the oocyte cytoplasm, has led to considerable interest in the possibility of storing the spermatozoa in the freeze-dried state. Logically, the micro-manipulation technique needed for ICSI can be considered as a replacement for sperm transport and any steps requiring motility or the acrosome reaction, and, at least theoretically, the only sperm component necessary for the production of a zygote is the nucleus. From that argument, it can be concluded that damage to the mitochondria, axoneme and other cytoplasmic components should not be relevant to the developmental potential of freezedried spermatozoa. A number of reports have shown that this approach is feasible (Wakayama and Yanagimachi, 1998; Kaneko et al., 2003a,b; Ward et al., 2003; Liu et al., 2004; Kawase et al., 2007; Kusakabe et al., 2008) and that live offspring have been produced in mice and rabbits following ICSI using freeze-dried nuclei. This mirrors the use of freeze-dried nuclei for nuclear transfer (Loi et al., 2008) after 3 years storage at room temperature. The principles of anhydrobiosis (i.e. the natural freeze-dried state) mentioned above as occurring in tardigrades and eelworms are very relevant in this context. The presence of trehalose confers protection against DNA fragmentation (Loi et al., 2008), as expected from theoretical principles (Rudolph and Crowe, 1985; Rudolph et al., 1986; Beattie et al., 1997). The disulfide status of the spermatozoa is also important in determining the success of freeze-drying; Kaneko et al. (2003b) showed that the ability of cauda epididymal spermatozoa to support normal development could be enhanced if they were first treated with diamide, which oxidises free sulfhydryl (SH) groups to form disulfide bonds. Conversely, if the disulfide bonds were first reduced with dithiothreitol (DTT), their ability to support development was lost. These findings are significant because they directly suggest a crucial role for the nuclear basic proteins – protamine 1 and protamine 2 – as determinants of success in freeze-drying. These protamines package sperm DNA into very compact and highly stable chromatin structures, a function that is largely mediated by the presence of cysteine groups and the formation of disulfide bonds (Balhorn et al., 2000; Balhorn, 2007). Although the number of cysteine residues found in the protamine 1 of eutherian mammals is species specific, and typically varies from five to ten, it is notable that the protamine 1 of most marsupials completely lacks cysteine groups (Retief et al., 1995). It is possible, therefore, that this will have negative implications for any attempts to freeze-dry marsupial spermatozoa. Semen cryopreservation has been applied successfully in only a relatively small number of species, and despite the commercial species such as cattle, sheep and pigs having attracted large amounts of research investment, considerable and unexplained variation in post-thaw semen quality exists between individuals. Although this phenomenon is a nuisance for those involved in routine semen cryopreservation, it might, nevertheless, be possible to exploit these differences and find a way to refine our knowledge of sperm cryoinjury and, also, a way to improve the between-species situation, which is much less consistent. Recent studies have suggested that there may be a genetic basis for the variation in post-thaw semen quality, and these indicate that modern molecular technologies should enable the identification of markers linked to genes that influence the stability of spermatozoa in the face of extreme stress. Although there are long-standing hypotheses, especially on sperm membranes and their lipid content (Parks and Lynch, 1992), that attempt to explain interspecies variation in the susceptibility of spermatozoa to cryoinjury, these seem rather unsatisfactory when the issue of within-species variation is considered. If sperm quality and fertility across males of a single species is normally sufficient to achieve successful mating and conception, it is likely that the sperm membrane lipid composition would have to be maintained within certain limits in order to preserve appropriate sperm membrane fluidity and functionality. If that is the case, explanations for inter-male variations in sperm survival during and after cryopreservation may be more complex than previously thought. The existence of inter-male variation in terms of semen freezing is well known (at least anecdotally), and males are often known as ‘good’ and ‘bad’ freezers. More formal demonstrations of these differences have been published for a number of species: mice (Songsasen and Leibo 1997); dogs (Yu et al., 2002); rhesus monkeys (Leibo et al., 2007); pigs (Thurston et al., 2002); and stallions (Ortega-Ferrusola et al., 2009). The mouse studies noted above are of particular interest because they demonstrated that there were major differences in the postthaw fertility (using in vitro fertilization – IVF) of spermatozoa from different genetic lines. Slight but consistent strain-dependent morphological differences in sperm tail and cytoplasmic droplet morphology are known to correlate with in vitro fertilization rates (Kawai et al., 2006), although epididymal dysfunction is partly responsible for this effect by inducing tail bending at the sperm neck region in some lines. An earlier series of studies in which different mouse strains were compared (Krzanowska et al., 1991, 1995) had linked the ability of spermatozoa to penetrate the zona pellucida with subtle differences in sperm head shape and, moreover, had shown that these differences were dependent upon genotype. Given that these differences exert influences on fertilizing ability even when no freezing methods are involved, it is not surprising that cryopreservation only amplifies the genetically based variation in fertility. The observation that the mouse genotype might be responsible for differences in post-thaw fertility variation is consistent with other studies in pigs. In an effort to minimize genetic variation due to other factors, Thurston et al. (2002) categorized more than 100 boars from a genetically homogeneous line into ‘good’, ‘bad’ and ‘intermediate’ freezers, based on extensive analysis of their post-thaw semen quality. She then compared the genetic characteristics of the ‘good’ and ‘bad’ freezers by using amplified fragment length polymorphism (AFLP) and was able to show that there were at least 16 polymorphic regions of the genomic DNA that correlated with post-thaw semen quality. In a parallel study of sperm head morphology, she also demonstrated systematic variation in pre-freeze sperm head shape that correlated with the post-thaw semen quality (Thurston et al., 2001). A more recent study based on pre-freeze and post-thaw semen quality parameters (Safranski et al., 2011) has used quantitative genetic approaches to implicate genotypic differences in this relationship.
1University of Sheffield, Sheffield, UK;2 South-East
Zoo Alliance for Reproduction & Conservation, Yulee, Florida, USA
Introduction
Cryobiological Theory
Physical changes during freezing
Cryoprotectants
Developments in the use of cryoprotective additives
Vitrification
Freeze-drying
Genetic Influences on Semen Cryopreservation and Cryoinjury
Pathogens in Semen, Semen Preservation and Biosecurity
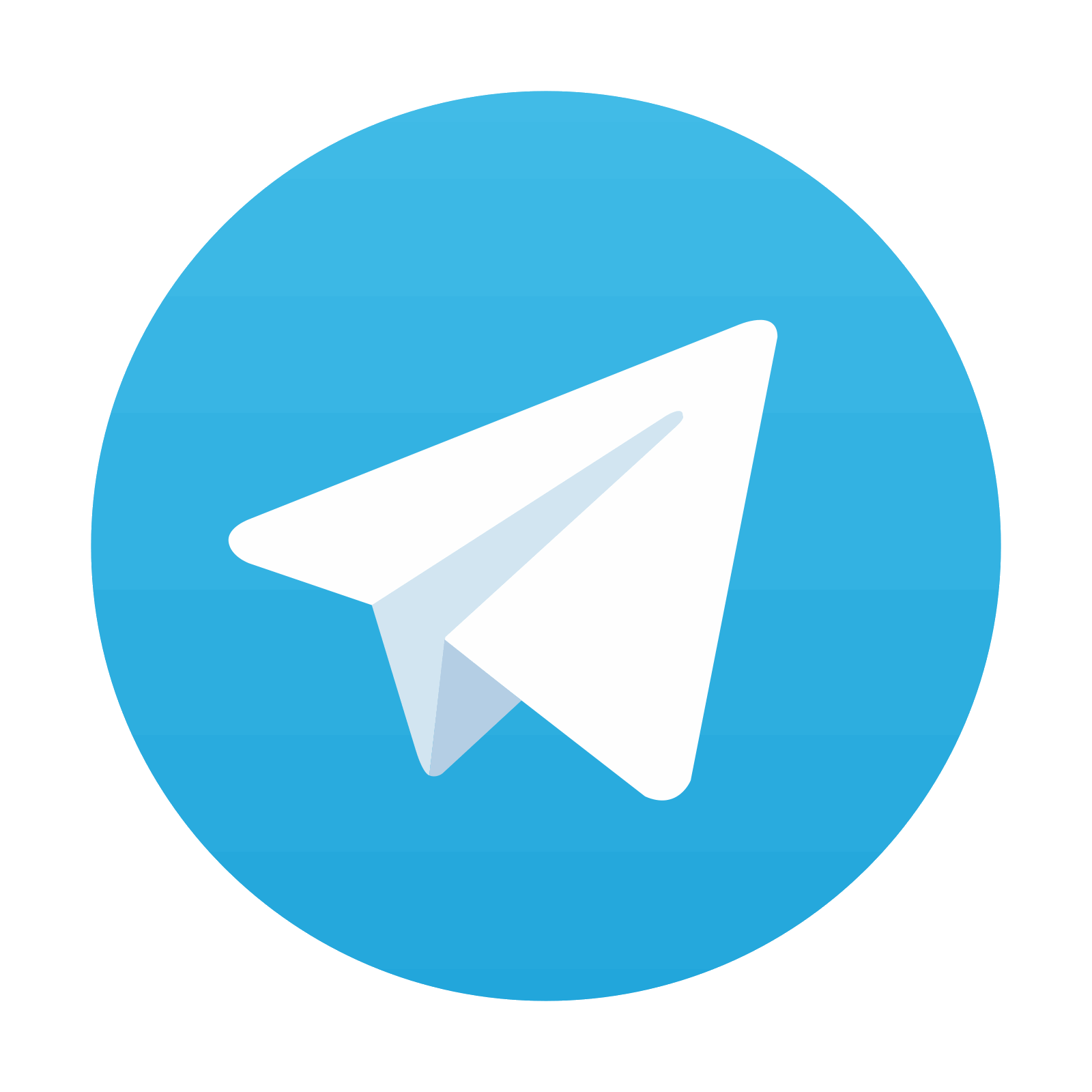
Stay updated, free articles. Join our Telegram channel
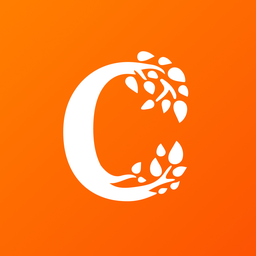
Full access? Get Clinical Tree
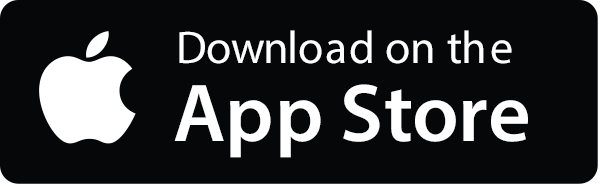
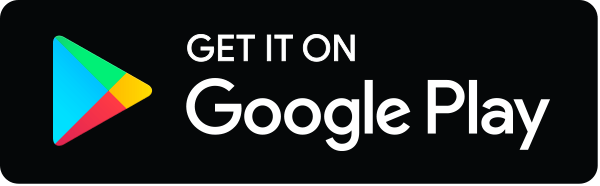