Fig. 3.1
Fluoride metabolism in animal body after its oral intake
3.1 Absorption
Fluoride compounds can be absorbed via different routes including the gastrointestinal tract, respiratory tract, and through skin and mucous membranes.
3.1.1 Gastrointestinal Tract
The gastrointestinal tract is the major route of fluoride uptake, except for animals raised in polluted or industrial environments where fluoride compounds are present in high concentration as vapors, fumes, or dust. In animals, fluoride is absorbed mostly in the stomach (in simple-stomach animals), rumen, abomasum (in ruminants), and upper intestine by a passive process, although its excretion into the mucosa of the intestine is by active transport (Parkins 1971). The rate of active transport of fluoride in intestinal mucosa decreases with the increasing age of the animal (USEPA 1980). Permeation of fluoride through the gastric mucosa is pH dependent; the higher acidity of stomach contents increases F absorption. In the stomach under acidic conditions, fluoride ions can reversibly combine with hydrogen ion to form hydrogen fluoride (HF) or hydrofluoric acid, which is an uncharged molecule and can readily pass through biological membranes. Hydrofluoric acid has pKa 3.45. Hence, any fluoride present at a pH below 3.45 will exist mainly in the undissociated form as HF. At pH above 3.45, as in blood plasma, tissue fluid, and the like, fluoride exists mainly in ionized form. In ruminants, only about 50 % of ingested fluoride is absorbed in the rumen, because the rumen pH is around 5–6, which is not suitable for fluoride absorption (Weinstein and Davison 2004).
The rate and extent of F absorption in the gastrointestinal tract is also regulated by several other factors, the most important among them being the chemical nature and solubility of fluoride compounds. In general, inorganic F compounds are more soluble and hence rapidly and extensively absorbed from the gastrointestinal tract. Sodium fluoride has the highest bioavailability, whereas calcium fluoride, magnesium fluoride, and aluminum fluoride have poor bioavailability. Bioavailability of fluoride from bone and fish meal is nearly 50–60 %. Other factors modulating F absorption include species, sex, and age of the animal and presence of dietary components including minerals (divalent cations), fat, protein, and fiber. In human beings, only 50 % of the fluoride from fish protein concentrate is absorbed because most of the fluoride is in the form of highly insoluble fluorapatite. High fat and high cereal products in the diet reduce or delay fluoride absorption. Absorption of fluoride ingested along with milk is slower than that ingested with water (USEPA 1980). Almost all fluoride naturally present in water is absorbed when the gastrointestinal tract is empty. However, the rate and extent of F absorption decreases in the presence of milk or other food items. It was observed that F from sodium fluoride or disodium mono-fluorophosphate was absorbed up to 100 % when ingested along with water in a fasting state. However, a decrease in F absorption by 20–30 % occurs when they are ingested along with milk or baby formula (Whitford 2005). In simple-stomach animals, high protein in the diet favors F absorption by stimulating gastric acidity. High Ca, Mg, Al, and B in the diet reduce fluoride absorption (Cerklewski 1997). The decrease in absorption is due to the binding of fluoride with these cations, which ultimately increases fecal excretion of fluoride.
Fluoride absorption in the gut of herbivorous insects is very low and only about 0.1–9 % of the ingested fluoride is retained. This happens because their gut pH is usually alkaline exceeding 7, and there is an absence of calcified tissues that can act as a sink for fluoride (Weinstein and Davison 2004).
3.1.2 Respiratory Tract
Fluoride released into the air from volcanoes and industrial activities exists both in gaseous and particulate forms. Common gaseous forms of fluoride include HF, silicon tetrafluoride (SiF4), carbon-tetrafluoride (CF4), and hexafluorosilicate (H2SiF6). On the other hand, sodium fluoride (NaF), aluminum fluoride (AlF3), calcium fluorosilicate (CaSiF6), fluorspar (CaF2), and cryolite (Na3AlF6) are common particulate forms of fluoride emitted by industries. Volcanic eruptions are major natural sources of airborne fluoride in many parts of the world. HF is the major gaseous form of fluoride released by volcanoes. The annual global release of HF from volcanic sources ranges from 60 to 6000 kilotonnes, of which approximately 10 % may be introduced directly into the stratosphere (WHO 2002). In addition, other fluorine compounds (such as SiF4, H2SiF6, and F2) are also present in volcanic gases. These gaseous and particulate fluoride compounds, depending upon their solubility and particle size, may be absorbed partially or completely through the respiratory tract (WHO 2002). HF has high water solubility and is rapidly absorbed in the upper respiratory tract. The particulate F compounds are deposited in the nasopharynx, the tracheo-bronchial tree, and the alveoli, and their absorption depends upon their water solubility and particle size. The larger particles may be coughed up and reach into the gastrointestinal tract where they are absorbed or excreted in feces. In a study in rats, controlled exposure of HF (8.7 mg HF/m3 in the air for 2 h/day for 6 months) had no apparent effect on appearance, behavior, and weight gain, but resulted in F accumulation in hair, bone, and teeth (Stolarska et al. 2000). Fluoride absorption from the respiratory tract may have some significance in animals living in areas near industries emitting gaseous fluoride.
The respiratory tract appears to be an important route of fluoride uptake in factory workers, wherein prolonged occupational exposure to even moderately high fluoride concentration in ambient air can cause osteo and dental fluorosis. However, for herbivorous animals living in areas where the air is polluted with particulate or gaseous fluoride compounds, the respiratory tract is not the sole route of fluoride absorption. Most of the airborne F emissions contaminate soil, water, and vegetation a long distance from the source of emission (Radostits et al. 2000). Fodder plants and grasses grown in polluted areas can accumulate a large amount of fluoride. High F concentrations in forage and grasses growing in the vicinity of industrial F emissions are therefore quite common (Swarup and Dwivedi 2002). Thus, fluoride uptake from soil and vegetation can significantly contribute to the total fluoride burden in herbivores living in such areas.
Fluorine gas is extremely irritating and causes nasal and eye irritation (even at low levels), and death due to pulmonary edema (at high levels) after inhalation. Likewise, acute inhalation of HF following facial splashes with hydrofluoric acid can cause bronchiolar ulceration, pulmonary hemorrhage/edema and death. In addition, renal and hepatic damage also occurs in animals after exposure to excess fluorine or HF gas (ATSDR 2003) suggesting substantial absorption of F through the respiratory tract.
3.1.3 Dermal and Other Routes
Dermal exposure of fluorine, HF, and hydrofluoric acid can cause serious damage to skin and mucous membranes due to irritation (itching or burning sensation) and burn. The severity of the damage is directly related to the concentration and duration of exposure. In rabbits, a 1-min exposure to 2 % hydrofluoric acid did not produce skin lesions; however, necrotic lesions were observed after a 1–4-h exposure. When hydrofluoric acid is applied directly over the skin, it is absorbed quickly through the epidermis causing extensive damage and increased fluoride concentration in the systemic circulation (ATSDR 2003). Dermal absorption of other fluoride compounds, particularly those occurring in solid state, are perhaps very low. The dermal route of absorption does not appear to play any significant role in chronic fluoride toxicity in vertebrates. Even in invertebrates, such as silkworm larvae that are highly sensitive to fluoride toxicity, the gastrointestinal tract is the major route of F absorption.
Many insects including honeybees, when exposed to a polluted environment, deposit fluoride on the external body surface as dry deposits, rather than in the body tissues (Davies 1989). The effect of this fluoride deposition is not clearly known. The role of fluoride pollution on honeybee depopulation in industrial vicinities is a matter of much debate (Weinstein and Davison 2004).
Dermal and other routes of fluoride absorption have not been investigated widely in aquatic animals, although many aquatic invertebrates and fish are highly sensitive to fluoride. Studies of fluoride accumulation in body tissues and its toxic effects in fish and other invertebrates are numerous, but data on the relative contribution of dermal, gastrointestinal tract, and other routes of fluoride absorption are lacking. In fish, fluoride compounds can also enter through fluoride-permeable gills (Bielawski 1971). The contribution of gills towards total fluoride uptake is also not clear and the gastrointestinal tract is supposed to be the major route of F uptake.
3.2 Distribution and Retention
Irrespective of the route of exposure, absorbed fluoride reaches into blood circulation which acts as a transport medium and carries F to different body parts. Fluoride in blood exists in two forms, organic and inorganic fluorides. The inorganic F is only important from a toxicological point of view, as it is the only active form. Values of total F in blood plasma are of little biological interest because there is no known conversion of organic to inorganic forms (Venkateswarlu 1975). In blood, about 75 % inorganic fluoride remains in plasma, about 5 % bound with plasma proteins, and rest is present in or on erythrocytes. Out of total plasma inorganic fluoride, 15–70 % remains in ionic form (Singer and Armstrong 1964). The ionic form does not diffuse through the cell membranes; the diffusion occurs as HF which is in a nonionic state. In human serum at least 50 % of the total serum F is in nonionic form, but the ionic form may predominate following excess F uptake (Swarup and Dwivedi 2002).
The time lag between ingestion and peak plasma concentration varies with solubility of the fluoride compound, emptiness of the stomach, and the nature of the F carrier. For example, if a given quantity of sodium fluoride is ingested with milk, it will be absorbed slowly and the time taken to reach peak plasma concentration will be longer than when ingested with water. In human beings, it takes about 30–60 min to reach peak plasma fluoride concentration after ingestion of sodium fluoride with water (Carlson et al. 1960). From plasma, F migrates across cell membranes of nearly all soft tissues which have a steady-state tissue-to-plasma concentration ratio ranging from 0.5 to 0.9. Exceptions to this are brain and fat which have a considerably lower ratio and kidneys which have a higher ratio because fluoride is concentrated in the renal tubular fluid (Whitford et al. 1976).
The peak plasma F concentration starts declining due to two main reasons: uptake by calcified tissue and excretion in urine. Fluoride sequestration by calcified tissues from the plasma pool occurs through exchange for other anions, such as hydroxyl, citrate, and carbonate ions. In general, the clearance of fluoride from plasma by the skeleton is inversely related to the stage of skeletal development. Skeletal uptake, however, can be positive or negative depending on the level of fluoride intake, hormonal status, and other factors (Whitford 1994a). Plasma F concentration is not regulated by homeostasis and F metabolism is modulated by several factors including chronic and acute acid-base disturbances, hematocrit, altitude, physical activity, circadian rhythm and hormones, nutritional status, diet, and genetic determinants (Buzalaf and Whitford 2011).
3.2.1 Transplacental Passage
In human beings, fluoride passes freely through the placenta when fluoride concentration in maternal blood is low; but placenta regulates fluoride transfer and protects the fetus when maternal blood fluoride concentration is high (USEPA 1980; Mohanty et al. 2011). This protective mechanism is perhaps a passive process and the fetus is protected by the bulk of the maternal fluid space, quick clearance, slow placental fluoride diffusion, and ectoptic calcification of the placenta (Erickson and Malmnas 1962).
In cattle, research findings on transplacental fluoride transport are conflicting. One study demonstrated that fluoride does not pass from dam to offspring in an amount sufficient to induce fluorotic changes (Shupe et al. 1963). Similar observations were recorded in Holstein cows given added sodium fluoride in the diet (Shupe et al. 1992). However, in another study when the pregnant cattle were exposed to high fluoride intake, fluoride accumulated in the bone and teeth of the fetus and dental and bony lesions appeared in the newborn calves (Krook and Maylin 1979). In calves born to a fluoride-intoxicated cow, congenital fluorosis is manifested as brown discoloration of enamel, enamel hypoplasia, brown mottling of bone, severe retardation of cartilage cell differentiation, atrophy of osteoblasts, osteopenia, atrophy of bone marrow cells, serous atrophy of bone marrow fat, and severely stunted growth (Maylin et al. 1987).
Transplacental passage of fluoride has not been widely investigated in different animal species. In horses, it is unlikely that enough fluorine passes the placental barrier to induce fluorotic changes in offspring (Shupe and Olson 1971). The effect of variation in placental types on transplacental F transport in different animal species is not clear thus far. Absence of congenital fluorotic lesions in most of the animal species suggests that, despite differences in structure and mineral transport mechanism, all types of placenta provide some protection to the fetus from excess maternal fluoride.
3.2.2 Cerebrospinal Fluid
Fluoride concentration in the CSF in human beings and horses is 50 % or less than that of plasma (Whitford 1994a). Transport of fluorine, as with other halogen and ionic substances in CSF, is an active process. The blood–brain barrier appears to protect the brain from flooding of excess fluoride. Fluoride concentration in the CSF increases marginally only in patients with severe fluoride intoxication or the breakdown of the blood–brain barrier (Hu and Wu 1988).
3.2.3 Skeleton and Other Calcified Tissues
Approximately 99 % of the body F burden is associated with the calcified tissues. Bone, as in the case of lead, acts as a natural sink for fluoride. Bone fluoride levels can be used to quantitate long-term fluoride exposure. Bone is composed of water (45 %), inorganic salts, mainly Ca and P (35 %), and organic matrix or osteoid (20 %) consisting of collagen and noncollagenous glycoproteins and proteoglycans. The inorganic part of the bone comprises mainly mineral hydroxyapatite, and forms crystals that are deposited upon and parallel to the collagen fibers in a regular manner. These crystals are surrounded by a hydration shell that permits free flow of fluoride ions between the extracellular fluid and interior of the crystal (Swarup and Dwivedi 2002).
Fluoride deposition in bone occurs in two phases. In the first phase, fluoride ions diffuse from extracellular fluid into the hydration shell and displace hydroxyl groups in the bone crystal surface to make a mixed fluorohydroxyapatite (Hodge and Smith 1970). This is a rapid and reversible process. Steady-state equilibrium exists between the F concentration in the extracellular fluids and F deposited on bone surface layers (Florkin and Stotz 1971). In the second phase, F is deposited in the denser and deeper parts of the bone. This is a slow process as interlacunar apatite crystals of bone are closely packed and have little fluid. Here F is incorporated deeper into the interlacunar apatite crystal at a rate nearly equal to deposition of phosphorus as bone phosphate. Once F is incorporated deeper into the apatite crystals, it cannot be removed from the bone without resorption of the crystal. This happens during the osteoclastic–osteoblastic cycle of bone remodeling (Hodge and Smith 1970). Fluoride deposition in both surface and deeper parts of bone and teeth is maximum during the growth phase as crystallites are small and have more hydration facilitating F diffusion.
Bone fluoride concentration increases as animals grow older, even in animals on a low fluoride diet (Shupe et al. 1963). Fluoride uptake and concentrations differ among different types of bone and parts of a particular bone (Kay 1975). In the long bones, F concentration is higher at the ends and lower in the shaft; higher in the periosteal and endosteal region and lower in the middle region (Weidmann and Weatherell 1970). Fluoride concentration is higher in bones that have a high cancellous component (ribs, vertebrae, and sternum) than the bones having a more cortical component (compact bones; Marier et al. 1963). This occurs because F deposition depends upon the metabolic activity and vascularization of the bone (USEPA 1980). During chronic toxicity F deposition in the skeleton proceeds rapidly at first and then more slowly until eventually a saturation stage is reached. Beyond this saturation point, flooding of the soft tissues with fluorine occurs causing metabolic breakdown and death of the animal (Underwood and Suttle 1999). In animals, bones can be classified on the basis of F concentration as: 300–400 ppm-normal; <4500 ppm-innocuous F concentration (compact bone); 4500–5500 ppm-marginal osteofluorosis; 5500 ppm (compact bone) or >7000 ppm (cancellous bone) toxicosis; and 15,000–20,000 ppm-saturation (Swarup and Dwivedi 2002).
F is not irreversibly bound to bone; it is released either after cessation of excess F uptake or when the diet cannot sustain mineralization of the skeleton (e.g., during lactation). Fluoride is released from bone in two phases. The first phase is a rapid process that takes weeks and involves anion exchange in the hydration shell. The second phase is slower with an average half-life of 8 years and occurs due to osteoclastic resorption of the bone. F is released slowly from compact bones in comparison to trabecular bones (WHO 1984).
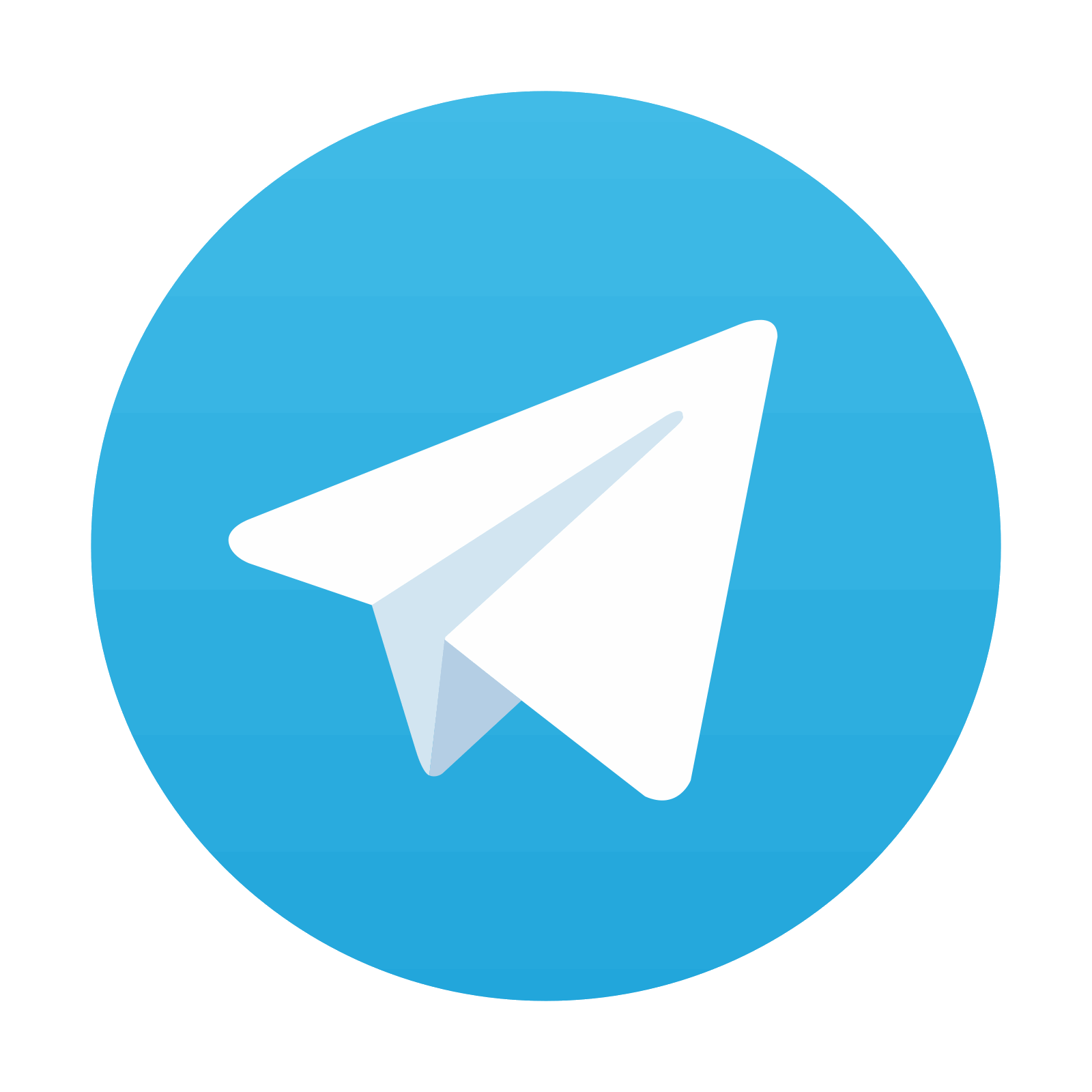
Stay updated, free articles. Join our Telegram channel
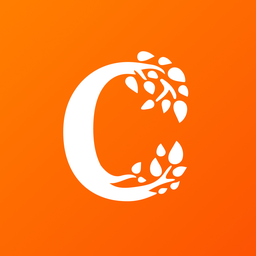
Full access? Get Clinical Tree
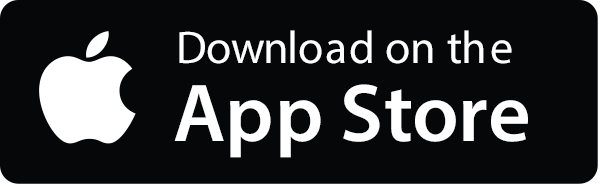
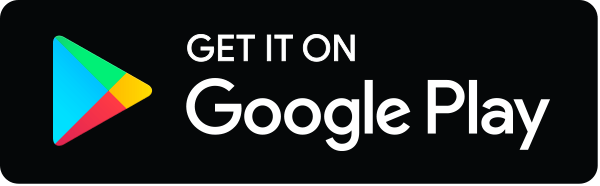