Robert E. Meyer Professor Emeritus, Department of Clinical Sciences, College of Veterinary Medicine, Mississippi State University, Mississippi, USA Anesthesiology and the intentional killing of animals at first glance seem to have diametrically opposed goals. The former is concerned with maintaining life, whereas the latter takes it; indeed, the act of performing euthanasia can be both physically and emotionally difficult for animal workers, caretakers, and veterinarians because of this perceived conflict [1,2]. However, the humane treatment of animals has increasingly become a subject of public debate and discussion, and a conservative and humane approach to the intentional killing of any creature is warranted, justifiable, and expected by society. As veterinarians, we take an oath on entry to the profession “… to use my scientific knowledge and skills for the benefit of society through the protection of animal health and welfare, the prevention and relief of animal suffering, the conservation of livestock resources …” [3]; further, we are reminded that our moral duty is to the welfare of animals under our care [4,5]. Therefore, whether for welfare concerns, food and fiber production, or in response to natural or man‐made disasters, our duty as compassionate veterinarians is to minimize or eliminate animal pain, anxiety, and distress. Viewed in this context, veterinary anesthetists and anesthesiologists are uniquely positioned to contribute meaningfully to matters pertaining to the humane taking of animal life. Determining the humaneness of euthanasia, slaughter, or depopulation methods can be difficult because as humans we can never fully know or understand the subjective experiences of animals during loss of consciousness. This problem has vexed veterinary medicine since 1847 when, after administering the then newly described ether anesthetic to dogs and cats, the eminent British veterinarian Edward Mayhew concluded [6]: The results of these trials are not calculated to inspire any very sanguine hopes. We cannot tell whether the cries emitted are evidence of pain or not; but they are suggestive of agony to the listener, and, without testimony to the contrary, must be regarded as evidence of suffering. The process, therefore, is not calculated to attain the object for which in veterinary practice it would be most generally employed, namely, to relieve the owner from the impression that his animal was subjected to torture. In another light, it is not likely to be of much practical utility. One of the defining characteristics in humans undergoing anesthesia is feeling as if one is having an “out‐of‐body experience,” suggesting a disconnection between one’s sense of self and one’s awareness of time and space [7]. Although we cannot know for certain the subjective experiences of animals, one can speculate that similar feelings of disorientation may contribute to apparent signs of animal distress observed during anesthesia. Fortunately, the development of anesthesia methods for animals continued despite Dr. Mayhew’s initial misgivings. Determining the humaneness of killing methods depends on our ability to assess pain and consciousness within the contexts of method and application. Events such as vocalization, paddling, or convulsions are often interpreted as unequivocal evidence of animal suffering. Although unpleasant to observe, such events have much less effect on animal welfare when they occur after loss of consciousness. The purpose of this chapter is to review the mechanisms and methods used for the intentional taking of animal life, including during the fetal stage, within the contexts of pain perception and consciousness. Recently described novel methods of low atmospheric pressure stunning and foam depopulation for poultry are described, in addition to considerations on agent purity requirements for humane killing. For a more detailed overview of euthanasia, including recommendations and procedures for specific species, readers are strongly encouraged to consult the most recent guidelines issued by the American Veterinary Medical Association (AVMA) [8], the Canadian Council on Animal Care (CCAC) [9], and the World Organisation for Animal Health (WOAH, formerly OIE) [10]. A notable update in the 2020 AVMA Euthanasia Guidelines is the clarification of the difference between sedation and anesthesia. Sedation is defined as “a state of CNS depression in which the animal is awake but calm, and with sufficient stimuli may be aroused.” General anesthesia is defined as “a method used to produce unconsciousness.” Anesthetized animals are not arousable by noxious stimuli. This distinction is important in that several common techniques (e.g., placement of intraosseous cannulas, intraorgan or intracardiac injections, intrathecal lidocaine, and use of saturated potassium (K+) or magnesium (Mg+) solutions) are considered acceptable only when performed on anesthetized or fully unconscious animals. Euthanasia (derived from the Greek eu + thanatos, or “good death”) generally refers to the goal of ending the life of an individual animal in a way that minimizes or eliminates pain and distress. Currently, the term is applied to situations where death is induced in a manner, that is, (1) in accord with an animal’s interests and/or because it is a matter of welfare (humane disposition); and (2) where humane techniques are applied to induce the most rapid, painless, and distress‐free death possible. Hence, for an act of intentional killing to be considered euthanasia, both humane disposition and humane techniques must be considered together [8]. Euthanasia is not a substitute for poor veterinary care and should never be considered as the “easy way out” or as a means to justify questionable management decisions. Rather, euthanasia provides a humane and responsible solution to improve animal welfare by alleviating animal suffering. There is debate as to whether the term euthanasia appropriately describes the killing of some animals at the end of biological experiments and of unwanted shelter animals; extensive discussion regarding these issues can be found in papers by McMillan [11] and Pavlovik et al. [12]. For a more extensive review of the moral and ethical considerations of euthanasia, including the decision processes, the reader is directed to the 2020 AVMA Guidelines on Euthanasia [8]. Within the 2020 AVMA Euthanasia Guidelines, methods are classified as Acceptable, Acceptable with Conditions, and Unacceptable; similar terminology is applied to euthanasia methods throughout this chapter: The following criteria were used in the 2020 AVMA Guidelines on Euthanasia to evaluate methods used to take the life of animals intentionally: Humane slaughter refers to processes and methods employed to intentionally kill animals raised for food, fur, or fiber production [13,14]. The term applies both to individual animals killed on‐farm and to commercial production processes. From a welfare perspective, the term is inclusive of the transport and handling of an animal, the methods employed to induce unconsciousness, the period until unconsciousness is verified, and the time of death when the animal is ready for entry into the food chain. This means that efforts must be made to minimize and, where possible, eliminate distress, pain, suffering, or anxiety associated with the entire process of bringing that animal to slaughter. Although some humane slaughter methods may employ euthanasia techniques, not all humane slaughter methods meet the criteria required to be considered euthanasia. In addition to animal welfare concerns, important considerations with regard to humane slaughter processes include public health and safety, food safety and quality, environmental and economic sustainability, production adequacy and sustainability, occupational health and impact on the labor force, international animal welfare and trade standards, and religious and cultural aspects. In the United States, methods for humane slaughter are federally codified for cattle, calves, horses, mules, sheep, and swine by the 1958 Humane Slaughter (HS) Act and the 1978 Humane Methods of Livestock Slaughter Act (Pub. L. 85‐765; 7 USC 1901 et seq.) (Pub. L. 85‐765, Sec. 1, Aug. 27, 1958, 72 Stat. 862; Pub. L. 85‐765, §2, Aug. 27, 1958, 72 Stat. 862; Pub. L. 95‐445, §5(a), Oct. 10, 1978, 92 Stat. 1069) [15]. Although methods for the humane slaughter of poultry, fish, and rabbits are not specifically included in the 1958 and 1978 Acts, similar considerations should be applied to these species [13,14]. Depopulation is defined as the rapid destruction of large numbers of animals in response to emergencies, such as the control of catastrophic infectious diseases, or exigent situations caused by natural or man‐made disasters [16]. As defined by the AVMA in the 2019 Guidelines, the term applies to methods by which large numbers of animals must be destroyed quickly and efficiently with as much consideration given to the welfare of the animals as practicable, but where the circumstances and tasks facing those performing the depopulation are understood to be extenuating [16]. The AVMA classification of depopulation methods differs from terminology used for euthanasia; methods are classified as Preferred, Permitted in Constrained Circumstances, and Not Recommended. Depopulation is especially problematic from a welfare standpoint owing to several factors, including the sheer number of animals potentially involved, the need for rapid and decisive responses to limit disease spread or economic damage, and the potential for extenuating circumstances limiting availability or deployment of supplies, equipment, and personnel. This combination of factors can lead to consideration of expedient solutions of questionable humaneness. For example, in large confinement production facilities, loss of system ventilation, whether by intentional or accidental means, will eventually result in animal death; a process called “Ventilation Shutdown Plus” has been described for both swine and poultry depopulation [17,18]. Although application of mass depopulation methods may result in the same ultimate outcome, it is our duty as compassionate veterinarians to develop and employ methods that minimize animal suffering under these adverse circumstances. As with humane slaughter methods, depopulation may employ euthanasia techniques, but not all depopulation methods may meet the criteria to be considered euthanasia. Further, application of any recommended killing method must be performed in accord with applicable national, regional, or local laws governing drug acquisition and storage, occupational safety, and methods used for euthanasia and disposal of animals, with special attention to individual species requirements where possible. If drugs have been used, careful consideration must be given to appropriate carcass disposal and steps should be taken to avoid environmental contamination or harm to other animals [8]. Selection of the most appropriate killing method for a particular situation will depend on several factors, including the species and number of animals involved, available means for animal handling and restraint, the skill and proficiency of personnel, and the methods and equipment available. Information in the scientific literature and available from practical experience focuses primarily on domesticated animals, but the same general considerations can be applied to all species. Inevitably, there will be less‐than‐perfect situations in which “acceptable/preferred” methods or “acceptable with conditions/permitted in constrained circumstances” methods may not be possible; judgments on the suitability of the best method or agent under the circumstances will be necessary. It should be noted that any useful and potentially humane killing method can become inhumane through poor technique, improper application, or lack of strict adherence to specified conditions and contingencies. Euthanasia, humane slaughter, and depopulation methods initially produce unconsciousness through three basic mechanisms: (1) direct depression of neurons necessary for life functions; (2) hypoxia; and (3) physical disruption of brain activity. Death follows as the circulatory and respiratory centers fail, or as hypoxia or reduced pH renders intracellular processes non‐functional. However, loss of consciousness occurs at substantially different rates, such that the suitability of any particular agent or method depends on whether an animal experiences pain or distress prior to loss of consciousness. Pain is defined as a conscious perception. Pain is subjective in the sense that individuals can differ in their perceptions of intensity and also in their physical and behavioral responses. The International Association for the Study of Pain (IASP) describes pain as “an unpleasant sensory and emotional experience associated with, or resembling that associated with, actual or potential tissue damage.” This most recent IASP definition also includes contextual notes, several of which are relevant to this discussion: (1) pain is always a personal experience that is influenced to varying degrees by biological, psychological, and social factors; (2) pain and nociception are different phenomena; pain cannot be inferred solely from activity in sensory neurons; and (3) verbal description is only one of several behaviors to express pain; inability to communicate does not negate the possibility that a human or a nonhuman animal experiences pain [19]. Based on mammalian models, the perception of pain requires nerve impulses from peripheral nociceptors to reach an awake, functioning cerebral cortex and associated subcortical brain structures. Impulses from peripheral nociceptors are conducted by primary afferent fibers to either the spinal cord or the brainstem and two general sets of neural networks. Reflex withdrawal and flexion in response to nociceptive input are mediated at the spinal level, while ascending nociceptive pathways carry impulses to the reticular formation, hypothalamus, thalamus, and cerebral cortex (somatosensory cortex and limbic system) for conscious sensory processing and spatial localization. This distinction is important, in that movements observed in response to nociception can be due to spinally mediated reflex activity (unconscious), cerebral cortical and subcortical processing (conscious), or a combination of the two. Consequently, the choice of specific killing agent or method is less critical if it is to be used on an animal that is already anesthetized or rendered unconscious, provided that the animal does not regain consciousness prior to death. Although the perception of pain requires a conscious experience, defining consciousness, and therefore the ability to perceive pain, across many species is difficult. Amnesia, defined as loss of memory function in which old memories cannot be remembered or new memories cannot be formed, is a defining characteristic of anesthesia, in that with sufficient quantities, all anesthetics are capable of producing a state of amnesia where new memories cannot be formed [7]. Unconsciousness, defined as loss of individual awareness, occurs when the brain’s ability to integrate information is blocked or disrupted. Anesthetics produce unconsciousness either by preventing integration (blocking interactions among specialized brain regions) or by reducing information (shrinking the number of activity patterns available to cortical networks) received by the cerebral cortex or equivalent structure or structures. Further, the abrupt loss of consciousness that occurs at a critical concentration of anesthetic implies that the integrated workings of the interconnected neural states underlying consciousness may collapse nonlinearly [20,21]. In humans, onset of anesthetic‐induced unconsciousness has been functionally defined by loss of appropriate response to verbal command; in animals, loss of consciousness is functionally defined by loss of the righting reflex (LORR), also called “loss of position” (LOP) [22–24]. This definition, introduced with the discovery of general anesthesia over 160 years ago, is still useful because it is an easily observable, integrated whole‐animal response that is applicable to a wide variety of species. Although surrogate measures of brain activity such as an electroencephalogram or functional magnetic resonance imaging (fMRI) are often applied in this context, these methods cannot yet provide definitive answers as to onset of either human or animal unconsciousness; limitations of these methods for this purpose are reviewed later in the section evaluating animal distress. The usefulness of LORR/LOP as an easily observable proxy for loss of animal consciousness has been reinforced in studies where a reduction in alpha:delta (α:δ) brain wave ratios was found to coincide with LOP in chickens [25,26]. Vocalization or physical movement observed during application of humane killing methods is often interpreted as unequivocal evidence of consciousness. While purposeful escape behaviors should not be observed during the transition to unconsciousness, human and animal studies confirm that amnesia is produced and conscious awareness is blocked at less than half the anesthetic concentration required to abolish physical movement [23]. Once consciousness is lost, subsequent activities such as seizures, vocalization, reflex struggling, breath holding, and tachypnea can be attributed to the “excitement” phase or anesthesia stage 2 which, by definition, lasts from loss of consciousness to the onset of a regular breathing pattern [27,28]. Hence, vocalization and non‐purposeful movements observed following LORR/LOP are not necessarily signs of conscious perception by an animal. Previously, it was thought that finfish, amphibians, reptiles, and invertebrates lacked the anatomic structures necessary to perceive pain as we understand it in birds and mammals. However, most invertebrates respond to noxious stimuli and many have endogenous opioids [29], and there is increasing taxa‐specific evidence of the efficacy of analgesics to minimize the impact of noxious stimuli on amphibians and reptiles [30,31]. Suggestions that fish responses to pain merely represent simple reflexes [32] have been refuted by studies demonstrating differing forebrain and midbrain electrical activity in response to nociceptor stimulation [33,34]; further, finfish exhibit learning and memory consolidation when taught to avoid noxious stimuli [35]. Based on the above considerations, killing methods that “result in rapid loss of consciousness” and “minimize pain and distress” should be strived for, even in those species where it is difficult to determine that these criteria have been met. Physical methods (e.g., gunshot, captive bolt, cerebral electrocution, blunt force trauma, and maceration) produce instantaneous unconsciousness by destroying, or rendering non‐functional, brain regions responsible for cortical integration; death quickly follows when the midbrain centers controlling respiration and cardiac activity fail. Signs of effective stun and onset of unconsciousness have been reviewed in the context of slaughter. In cattle, sheep, and pigs, signs include immediate collapse (LORR/LOP) and a several‐second period of tetanic spasm, followed by slow hind limb movements of increasing frequency [36–40]. However, there is significant species variability in this response. The corneal reflex will be absent. Signs of effective electrocution are loss of righting reflex, loss of eye blink and moving object tracking, extension of the limbs, opisthotonos, downward rotation of the eyeballs, and tonic (rigid) spasm changing to clonic (paddling) spasm, with eventual muscle flaccidity. The corneal reflex will be absent. Although generalized seizures may be observed following effective application of physical methods, these most often follow loss of consciousness. Physical methods are inexpensive, humane, and painless if performed properly, and leave no drug residues in the carcass. Furthermore, animals presumably experience less fear and anxiety with methods that require little preparatory handling. However, as noted in the section, choice of killing method relative to onset of unconsciousness, physical methods usually require more direct physical proximity with the animals to be euthanized, which can be both offensive and upsetting for the operator. Physical disruption methods are often followed by adjunctive methods such as exsanguination or pithing to ensure death. Decapitation and cervical dislocation as physical methods of humane killing require separate comments. Electrical activity in the brain can persist for up to 30 s following these methods [41–44]; interpretation of the significance of this activity is controversial [45]. As discussed in the section evaluating animal distress, brain electrical activity cannot yet provide definitive answers as to the precise onset of unconsciousness. Other studies [46–50] indicate such activity does not imply the ability to perceive pain and conclude that loss of consciousness develops rapidly. Anesthetics and carbon dioxide (CO2) inhalation initially produce loss of consciousness through direct depression of the cortical neural system; death subsequently occurs due to respiratory or cardiovascular failure associated with agent overdose. Acute hypercapnia (defined as > 5% atmospheric CO2) rapidly reduces intracellular pH, producing unconsciousness and a reversible anesthetic state characterized by reduction of both basal and evoked neural activities [51–54] and inhibits central N‐methyl‐D‐aspartate (NMDA) receptors [55]. In contrast to inert gas killing methods, exposure to CO2 does not rely on the induction of hypoxia to cause unconsciousness and death and can kill over a wide range of concentrations [56]. Hypoxia is commonly achieved by displacing oxygen; this can be achieved through exposure to high concentrations of inert gases, such as nitrogen (N) or argon (Ar). To be effective, O2 levels of < 2% must be achieved and maintained, as re‐establishment of a concentration of O2 of 6% or greater prior to death will allow immediate recovery [56–59]. Carbon monoxide (CO) binds avidly to hemoglobin and produces hypoxemia by blocking uptake of oxygen within red blood cells. Exsanguination is another method of inducing hypoxemia, albeit indirectly, to ensure death in already unconscious or moribund animals. Low atmospheric pressure stunning of poultry (LAPS) represents a refinement in producing hypoxia (see the section low atmospheric pressure stunning). Whether a hypoxia‐based method is classified as stunning or killing depends on the amount of time the animal remains in the modified atmosphere; killing methods eliminate the concern that animals may regain consciousness. Hypoxia‐based methods are not appropriate for those species or stages of development that are tolerant to prolonged periods of hypoxemia. Nitrous oxide (N2O) is not a potent anesthetic in animals. The effective dose for N2O is above 100 vol %; therefore, it cannot be used alone in any species at normal atmospheric pressure without producing hypoxia prior to respiratory or cardiac arrest. In humans, the minimum alveolar concentration (MAC) (defined as the median effective dose preventing purposeful movement; see Chapter 28) for N2O is 104%; its potency in other species is less than half that in humans (i.e., approximately 200%). By comparison, the MAC for isoflurane is approximately 1.4%. Up to 70% N2O can be added to an inhaled fluorocarbon anesthetic‐oxygen vapor to speed the onset of unconsciousness through the “second gas effect.” However, owing to its reduced potency in animals, N2O will only reduce the fluorocarbon anesthetic MAC by 20–30%, or about half that expected in humans [60]. The combination of CO2 with N2O, administered at a displacement rate of 20 and 60% of the chamber volume per minute, produced LORR in C57Bl/6 and CD1 mice 10.3% faster than CO2 alone (N2O–CO2, 96.7 ± 7.9 (mean ± SD) s; CO2, 108.7 ± 9.4 (mean ± SD) s), and may represent a refinement over the use of CO2 alone [61]. A gradual displacement rate between 30 and 70% of the container volume per minute is currently recommended for administration of CO2 for euthanasia [8]. The build‐up, or wash‐in, of gases within enclosed spaces, is an exponential process dependent on chamber volume and gas displacement rate, such that time to loss of consciousness, and eventually death, will be a function of the time constant for that container [62]. The time constant can be calculated as the chamber volume divided by the gas displacement rate [62]. When starting from a gas concentration near zero, one time constant is required to reach a washed‐in gas concentration of 63% such that a gas volume displacement rate of 20%/min represents a time constant of 5 min (1 divided by 0.2). Thus, applying any vapor or gas at a displacement rate of 20% of the container volume per minute will result in an exponential rise to 63% of the wash‐in gas concentration within 5 min for a container of any size [62,63]. The application of inhaled gases into enclosed spaces can be further refined to meet specific inflow rates, delivery points, and optimal gas concentration profiles through use of computational fluid dynamic (CFD) modeling. Gas distribution and temperature within containers can be modeled in three dimensions using CFD software running inside desktop computer‐aided design (CAD) systems. CFD simulation has proved to be a very powerful tool for modeling transient gas concentrations during gas wash‐in and wash‐out. It has further reduced the need for time‐ and labor‐intensive experimental testing of many design alternatives for on‐farm depopulation methods without the need for extensive experimental testing [64]. Loss of consciousness, as LORR/LOP, should always precede loss of muscle movement. However, as with the physical killing methods, some animals may exhibit motor activity or convulsions following loss of consciousness with anesthetics or with hypoxia‐based methods. Indeed, anesthesia, coma, and generalized seizures all represent a loss of consciousness where both arousal and awareness in humans are low or absent [65]. Characteristic signs of effective neural depression are similar to those observed with physical methods or with deep levels of anesthesia, including LORR/LOP, loss of eye blink and corneal reflexes, and muscle flaccidity. Inhaled and hypoxia‐based methods require a number of conditions and contingencies for their proper and appropriate use, such that they are currently classified as “acceptable with conditions” in the 2020 AVMA Guidelines [8]. Agents and methods that prevent movement through muscle paralysis without inhibiting or disrupting the cerebral cortex or equivalent structures (e.g., succinylcholine, strychnine, curare, nicotine, and potassium or magnesium salts) are not acceptable as sole agents for humane killing of vertebrates because they result in conscious perception of distress and pain prior to death. In contrast, magnesium salts are considered to be acceptable as the sole agent for euthanasia in many invertebrates owing to the absence of evidence for cerebral activity in some members of these taxa [66,67]; further, there is evidence that the magnesium ion acts centrally to suppress neural activity of cephalopods [68]. Magnesium chloride is effective on moon jellyfish and sea stars without the adverse side effects noted with KCl and MgSO4 [69,70]. In summary, the cerebral cortex or equivalent structure(s) and associated subcortical structures must be functional for conscious perception of pain to occur. If the cerebral cortex is non‐functional because of neuronal depression, hypoxia, or physical disruption, pain is not experienced. Although distressing to observers, reflex motor activity or physical signs occurring following loss of consciousness, as defined by LORR/LOP, is not perceived by the animal as pain or distress. Given that we are limited to applying humane killing methods based on these three basic mechanisms, efforts should be directed toward education of involved personnel and toward achieving technical proficiency and refinement in the application of currently approved methods [71]. Stress and the resulting responses have been divided into three phases [72]. Eustress results when harmless stimuli initiate adaptive responses that are beneficial to the animal. Neutral stress results when the animal’s response to stimuli causes neither harmful nor beneficial effects to the animal. Distress results when an animal’s response to stimuli interferes with its well‐being and comfort [73]. Distress may be created by the conditions prior to application of stunning or killing methods (e.g., transport conditions, environment, or restraint), or by the conditions under which methods are applied (e.g., gradual gas/vapor displacement or immersion) [74]. Simply placing Sprague–Dawley rats into an unfamiliar exposure chamber containing room air produces arousal, if not distress [75]. Pigs are social animals and prefer not to be isolated from one another; consequently, moving them to the CO2 stunning box in groups, rather than lining them up single file as needed for electric stunning, improves voluntary forward movement, reduces handling stress, and reduces electric prod use [76]. While gradual‐fill inhaled methods may be less stressful than more rapid displacements for certain species in some circumstances [77], this may not be true for all species under all circumstances [8]. Distress may manifest behaviorally (e.g., overt escape behaviors, approach‐avoidance preferences (aversion)), or physiologically (e.g., changes in heart rate, sympathetic nervous system activity, hypothalamic–pituitary axis activity), such that a “one size fits all” approach cannot be easily applied to evaluate killing methods or determine specific species applications. Both sympathetic nervous system (SNS) and hypothalamic–pituitary–adrenal axis (HPAA) activation are well accepted as stress response markers. However, it has been suggested that responses to systemic stressors associated with immediate survival, such as hypoxia and hypercapnia, are likely directly relayed from brainstem nuclei and are not associated with higher‐order CNS processing and conscious experiences [78]. Marked increases in circulating catecholamines, glucagon, insulin, lactate, and free fatty acids have been reported in porcine experimental models where brain death is induced following induction of general anesthesia [79–81]. Forslid and Augustinsson reported that concentrations of norepinephrine and lactate increased 1 min after exposure of pigs to CO2 [82]. Borovsky et al. found an increase in norepinephrine in rats following 30 s of exposure to 100% CO2 [83]. Similarly, Reed et al. exposed rats to 20–25 s of CO2, which was sufficient to render them recumbent, unconscious, and unresponsive, and observed tenfold increases in vasopressin and oxytocin concentrations [84]. Hypothalamic vasopressin‐containing neurons are similarly activated in response to CO2 exposure in both awake and anesthetized rats [85]. In pigs undergoing euthanasia with physical methods resulting in immediate unconsciousness (e.g., captive bolt; two‐point electrocution) or inhaled methods where onset of unconsciousness is delayed (CO2; 70% N2–30% CO2), cortisol levels were observed to be similar, whereas plasma norepinephrine and lactate were similarly increased in all groups [86]; although all gases and mixtures induced open‐mouthed breathing prior to loss of righting reflex, the mean latency to onset of open‐mouth breathing and subsequent loss of righting reflex was substantially longer with 70% N2–30% CO2 than with CO2 alone. The fact that levels of stress hormones observed were similar with both physical and inhaled methods illustrates the difficulty in differentiating conscious from unconscious distress where interpretation is complicated by continued exposure during the period between loss of consciousness and death. Behavioral assessment (e.g., purposeful escape behaviors; open‐mouth breathing) and aversion testing have been used to subjectively evaluate inhaled killing methods in several species; these methods have recently been reviewed [8]. Aversion, defined as a desire to avoid or retreat from a stimulus, is usually determined using approach‐avoidance studies, where the strength of avoidance to a particular situation or condition is determined by the animal’s choice to forego a desirable reward. This quantitative feature makes aversion a powerful tool for behavior studies. However, it is important to note that although aversion is a measure of preference and may imply unpleasantness, aversion itself does not necessarily indicate pain or distress; further, approach‐avoidance tests fail to distinguish adequately between aversion and distress, which is the inability to respond appropriately (either behaviorally or physiologically) to a stressor [74]. In addition, agents identified as being less aversive (e.g., Ar or N2 gas mixtures) can still produce overt and extended signs of behavioral distress (e.g., open‐mouth breathing) prior to loss of consciousness under certain conditions of administration (e.g., gradual displacement application) [86]. Measurements of brain electrical function, such as the electroencephalogram (EEG), bispectral analysis (BIS™), and visual and auditory evoked potentials, have been used to quantify the unconscious state. In humans, the issue is intraoperative awareness under anesthesia; in animals, the problem is procedural humaneness relative to onset of unconsciousness. The problem is even more complex in animals where we cannot question them directly and must infer from their actions and responses [87]. Brain electrical activity is acknowledged to be limited for this purpose, especially when trying to conflate electrical activity with a complex process such as consciousness. EEG is not a direct measure of consciousness – it measures brain electrical activity, and while that activity changes with levels of consciousness, EEG cannot provide definitive answers as to onset of unconsciousness using the current state of the art. Although consciousness must vanish at some level between behavioral unresponsiveness and the induction of a flat EEG (indicating the cessation of the brain’s electrical activity and brain death), current EEG‐based brain function monitors are limited in their ability to indicate directly the presence or absence of unconsciousness, especially around the transition point [20,88]; also, it is not always clear which EEG patterns are indicators of activation by stress or pain [89]. A 2012 editorial in a human anesthesiology journal neatly sums up the issues [90]: The electroencephalogram has been the Holy Grail of anesthetic depth monitoring for more than half a century but has fallen on hard times lately, largely because the focus of the dialog changed from electroencephalogram as a monitor of “depth” to one of intraoperative awareness … consciousness and intraoperative awareness are neurobiologically exceedingly complex phenomena. This makes these states difficult to capture or evaluate with electroencephalography, no matter the parameter or sophistication of the processing algorithm. Recent studies examining the efficacy of the electroencephalogram bispectral index for minimizing the risk of intraoperative awareness confirm as much. Although loss of visual evoked potentials (VEPs) is associated with brain death, visual cortex neurons remain responsive to flash stimulation under desflurane anesthesia [91], and reduction in flash‐induced gamma (γ) oscillations in rat visual cortex is not a unitary correlate of anesthetic‐induced unconsciousness [92]. Large interindividual variations in auditory evoked potentials (AEPs) and BIS analysis make it impossible to discriminate subtle changes in clinical state of consciousness in real time during propofol anesthesia [93]. Similarly, blood oxygen level‐dependent (BOLD) MRI is a multifactorial surrogate for cerebral blood flow or cerebral blood volume, both of which are actual measures of neural activity. Although a BOLD MRI response is generally a good surrogate measure of neuronal activity, it is based on hemodynamic changes, which may not reflect actual neural activity patterns, especially during anesthesia or under pharmacological challenge; rather, observed effects may be directly due to drug effects, or indirectly through changes in autonomic activity, blood pressure, cardiac output, or respiration [94,95]. In contrast, imaging of cerebral blood flow using positron emission tomography (PET) has been used in human volunteers during manipulation of consciousness with anesthetics combined with response to verbal command [21]
8
Euthanasia and Humane Killing
Introduction
Terminology
Euthanasia
Humane slaughter
Depopulation
Pain and consciousness
Mechanisms of action
Evaluating animal distress
Stay updated, free articles. Join our Telegram channel
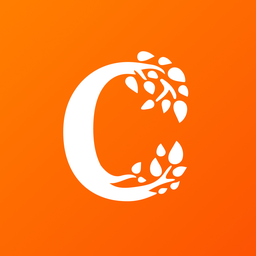
Full access? Get Clinical Tree
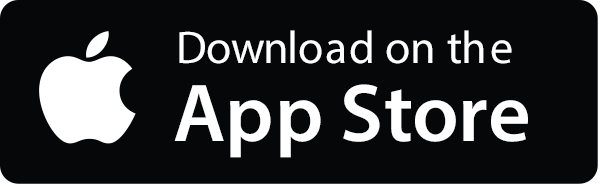
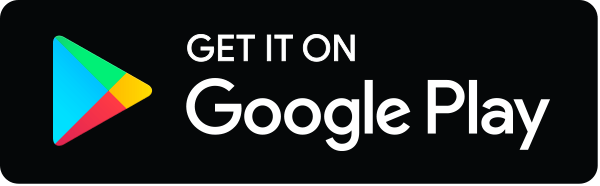