Chapter 15 In response to the requirements of the embryo during the early post‐implantation period, the haematopoietic and cardiovascular systems are the first to develop, supplying oxygen and a vascular system, prerequisites for embryonic growth and development. The production of blood cells occurs in particular anatomical locations as the embryo develops, occurring first in extra‐embryonic haematopoietic sites which are replaced by intra‐embryonic sites. Finally, haematopoietic stem cells (HSCs) move to bone marrow where red blood cell and white blood cell production continues throughout life in a highly regulated manner (Fig 15.1). The blood cells of normal animals have finite life spans and the number of each cell type in the circulation is usually maintained at a relatively constant level by a process of carefully controlled production of mature cells balanced with the rate at which white blood cells are removed from the circulation in response to inflammatory reactions or degenerative tissue changes or by removal of effete cells by macrophages or dendritic cells. In the absence of infectious disease, which can damage both red blood cells and white blood cells and lead to their premature removal, normal levels of cellular blood components are carefully controlled by the removal of ageing cells and their replacement by cells produced either in the bone marrow or by cells arising from the bone marrow and maturing in lymphoid organs or other tissues. Sustained abnormal elevations of blood cell numbers occur in neoplastic changes which affect the bone marrow or lymphoid tissue. In mammals, during embryonic development, the sequential sites at which haematopoiesis occurs include the yolk sac, the aorta‐gonad‐mesonephros region, the placenta, the foetal liver and finally the bone marrow (Fig 15.2). From the haematopoietic stem cells located in bone marrow, multipotent progenitors arise which can differentiate into erythroid, myeloid or lymphoid progenitor cells (Fig 15.3). Stem cells divide to produce more stem cells and also to produce progenitor cells that can respond to environmental influences and differentiate into mature functional blood cells. The HSC is capable of producing all the blood cells and lymphocytes required for optimal functioning of the body. This diversity is achieved through the generation of intermediate progenitor cells whose derivatives are restricted to defined lineages. Figure 15.1 Extra‐embryonic and intra‐embryonic sites of haematopoiesis in mammals. Following migration to the foetal liver, haematopoietic stem cells (HSCs) move to the bone marrow. From these stem cells in the bone marrow, multipotent progenitor cells arise which are the source of myeloid and lymphoid cells, not only during foetal development but also throughout the animal’s life. Figure 15.2 Outline of the tissues and structures, principally of mesodermal origin, associated with the formation of haematopoietic stem cells in mammals. Having reached their final destination, the bone marrow, these stem cells are the source of common myeloid progenitor cells and common lymphoid progenitor cells. Common myeloid progenitor cells are the source of myeloid blood cells and from common lymphoid progenitor cells B lymphocytes, T lymphocytes, natural killer cells and dendritic cells arise. Figure 15.3 Origin of haematopoietic stem cells in mammals, leading to the production of common myeloid progenitor cells and common lymphoid progenitor cells. From these progenitor cells, progressive differentiation of committed cells leads to the production of mature blood cells and platelets. Some unresolved questions relating to the precise origin of basophils and eosinophils require further investigation. As blood cells differentiate, growth factors and microenvironmental factors strongly influence the lineage of individual cell types. HSCs arise from haemogenic endothelium in the aorta. Runx‐1 is a requirement for the conversion of endothelial cells into blood stem cells. Maintenance of HSCs is dependent on the stem cell niche and the production of stem cell factor which binds to the KIT receptor protein and which is produced primarily by perivascular cells with some contribution from endothelial cells. Many tissues in mature animals are formed from cells with a defined life span which are replaced infrequently. In contrast, cells of some tissues such as epithelial cells and red blood cells are subject to constant replacement when the former are sloughed off and the latter are removed from the circulation by the spleen at defined intervals related to membrane alterations associated with ageing. Haematopoiesis, the production of blood cells, is a complex, highly regulated process which ultimately relies on bone marrow stem cells for its continuation. Haematopoietic stem cells are tissue‐specific stem cells which are capable of restoring all mature blood lineages during the lifetime of an individual animal. These self‐renewing stem cells, which derive from the endothelium of the dorsal aorta, retain their multipotency and, thereby, their ability to differentiate into all blood cell types. The ability to develop into various cell types sets haematopoietic stem cells apart from many other types of cells in the developing embryo. Postnatally, haematopoietic stem cells retain their multipotency and can produce progenitor cells capable of differentiating into erythroid, myeloid and lymphoid lineages (Fig 15.3). As the murine embryo has been used extensively to elucidate haematopoiesis in mammals, much of the data referred to in this chapter derive from experimental research carried out on the mouse embryo and foetus. The emergence of haematopoietic stem cells during embryological development is preceded by restricted haematopoietic activity in the yolk sac, characterised by limited self‐renewal on the part of erythromyeloid progenitor cells and the production of primitive erythrocytes, macrophages and megakaryocytes. This first stage of blood cell formation occurs without the participation of established self‐renewing stem cells and with the production of a limited lineage of blood cells. During this phase of haematopoiesis, B lymphocytes and T lymphocytes are not produced. Erythroid‐forming cells and proerythroblasts predominate in the developing foetal liver, succeeded by myeloid and lymphoid progenitor cells at later stages. In contrast, the developing foetal spleen has limited haematopoietic activity. Haematopoiesis in the murine embryo is associated with mesoderm‐derived haematopoietic stem cells in the yolk sac at a gestational age of approximately 7.5 days (Fig 15.4). In the aorta‐gonad‐mesonephros region, evidence of blood cell formation is demonstrable at approximately 10.5 days and placental involvement in haematopoiesis is evident shortly afterwards. Haematopoietic activity is demonstrable in the murine foetal liver by 11.5 days, in the foetal spleen by 14 days and in the bone marrow by 18 days. In the human embryo, primitive haematopoiesis has been demonstrated in the yolk sac at 30 days post‐conception and multipotent haematopoietic stem cells, which emerge directly from the aorta‐gonad‐mesonephros region at four weeks post‐conception, initiate definitive haematopoiesis. The site of haematopoiesis in the human foetus moves from the foetal liver to the bone marrow at 12 weeks post‐conception and, by birth, the bone marrow is the major site of haematopoiesis. In mice, however, haematopoiesis persists in the spleen for several weeks postnatally. Non‐haematopoietic cells in the bone marrow express cell‐surface molecules and secrete extracellular growth factors which influence haematopoietic cell development and, in some instances, may determine the lineage of developing cell populations. Figure 15.4 Timeline of extra‐embryonic and intra‐embryonic haematopoietic activity and the commencement of bone development associated with the murine embryo and foetus. The concept of a cellular microenvironment referred to as the haematopoietic ‘niche’, which strongly influences haematopoietic stem cell behaviour, has been proposed as an explanation for the functioning of these stem cells in the embryo and later in the foetus. The factors which operate in the niche environment include anatomical location, specific cell types, soluble molecules and signalling factors, along with physical influences, including oxygen tension, temperature and shear stress. These factors may promote haematopoietic stem cell self‐renewal and differentiation or limit haematopoiesis as animals age or experience pathological diseases. Although there are many unresolved questions relating to the direct and indirect effect of the niche microenvironment on haematopoietic stem cells, it is evident that signals and cell‐mediated interactions from a range of non‐haematopoietic cell types, including mesenchymal stem cells, multipotent progenitor cells and osteoblasts, exert a major influence on the changes which occur in these blood cell‐forming stem cells. Postnatally, the majority of HSCs are present in osteoblastic and vascular niches in the bone marrow where most haematopoietic activity occurs. Through secreted growth factors, these niches, which contain specialised cells, promote and support HSC growth and development. Endosteal cells, which differentiate into osteoblasts, promote cell‐to‐cell interactions with HSCs through the mediation of neural cadherin. Osteoblasts also express factors which regulate HSC numbers. These factors include angiopoietin, thrombopoietin, Wnt, Notch and osteopontin. Prior to the commencement of bone formation, HSCs which arise from endothelial cells located in the ventral wall of the dorsal aorta are present in sinusoidal or vascular areas before osteoblastic cells are formed. In both mice and humans, the bone marrow is the principal reservoir of HSCs and progenitor cells postnatally. Research in many vertebrate species indicates that HSCs derive from endothelial cells located in the ventral wall of the dorsal aorta. These haemogenic endothelial cells, which are mesodermal in origin and destined to become HSCs, migrate to proliferating tissues in a species‐specific manner and finally to the bone marrow. In the murine embryo, Nodal, Wnt‐3 and bone morphogenetic protein 4 (Bmp‐4) are required for primitive streak formation and mesoderm specification. Haematopoietic stem cells derive from ventro‐posterior mesoderm, specification of which requires Wnt ligands, Bmp and fibroblast growth factors. Immediately preceding HSC emergence, haemogenic endothelium development takes place in the ventral floor of the primitive dorsal aorta which expresses the vascular endothelial growth factor receptor encoded by KDR. In the mouse embryo and in the chick embryo bilateral endothelial aortae with established luminal formation, fuse in the midline before the emergence of HSCs. Accordingly, HSCs do not appear until fusion of the developing aortae occurs, giving rise to a single aorta in the midline. Notch signalling is actively involved in specifying the early trunk vasculature of vertebrates, including the dorsal aorta from which haemogenic epithelium arises. Available evidence indicates that HSCs are specified from haemogenic endothelium in the midline region of the floor of the dorsal aorta. In addition to its vascular patterning, Notch signalling is required for HSC specification. At least one Notch ligand, Jagged 1, has a role in the specification process. Notch 1 is required for definitive haematopoietic development upstream of Runx‐1. It has been postulated that Notch 1‐mediated activation of Runx‐1 operates independently through GATA‐2, as the Runx‐1 promoter lacks Notch‐responsive elements. At a particular stage in their development, HSC precursors must receive a Notch 1‐mediated signal to become HSCs. Thus, Notch ligands, including Jagged 1, either in the endothelium of the dorsal aorta itself or in cells in close proximity, direct the fate of cells destined to become HSCs. Although Runx‐1 expression in the dorsal aorta during HSC specification is one of the earliest markers of cells destined to become HSCs and is required for the transition of endothelial cells into HSCs, it is not required subsequently for the maintenance of HSCs. The bone marrow is the principal microenvironment in which HSCs become established and undergo self‐renewal and differentiation. Postnatally, HSCs migrate from the bone marrow into the blood stream, maintaining the characteristic homeostasis which applies to blood cells. At the interface between bone and the marrow space, osteoblasts interact with HSCs. Cytokines secreted by osteoblasts include granulocyte colony‐stimulating factor (G‐CSF), granulocyte‐macrophage colony‐stimulating factor (GM‐CSF) and interleukin‐6, which support HSC survival and promote differentiation. Other factors secreted by osteoblasts which influence HSC numbers include angiopoietin and thrombopoietin. Co‐culture experiments have demonstrated that osteoblasts are capable of supporting and maintaining HSCs, confirming the vital role of osteoid cells, as part of the niche population in the development and maturation of HSCs. It has been demonstrated experimentally, however, that the endosteal population is heterogeneous and cell types from this population are capable of differentiating into osteoblasts, adipocytes and chondrocytes in vitro and that these subtypes differ in their ability to provide support for HSC development and maturation. Of the endosteal cell types evaluated, chondrocytes were the least effective in promoting HSC development and osteoblasts were the most effective. Many other cell types present in the bone marrow postnatally, notably mesenchymal stem cells, CXCL12‐abundant reticular cells and surface cell antigen 1‐expressing bone‐lining cells are reported to act independently in the regulation of HSCs. A population of perivascular nestin‐expressing mesenchymal stem cells have been shown to influence HSC function. These mesenchymal stem cells express high levels of the chemokine CXCL12 which is known to regulate HSC migration. The dependence of HSCs on the niche cell population is evident from the association between niche cell differentiation and HSC regulation. Accordingly, as stromal cells differentiate, undergo change, express altered functional properties and migrate in response to physiological and other influences, it is likely that the impact of stromal cells on haematopoiesis will be reflected in the changes which occur in the bone marrow microenvironment. HSCs, which are self‐renewing, are the precursors of progenitor cells which become committed to one of the haematopoietic lineages at later stages in development. Evidence for the existence of multipotent stem cells in the bone marrow can be inferred from the ability of bone marrow cells to restore the immune system and associated blood cells of animals subjected to lethal X’irradiation. Multipotent stem cells can be cultured in vitro where they can differentiate into blood cells. The haematopoietic system can be considered as a highly regulated system with HSCs generating uncommitted precursors which later become committed to particular lineages and subsequently undergo differentiation into different blood cell types. Some transcription factors promote particular lineages and, as an example, the transcription factor GATA 1 is required for the differentiation of the erythroid lineage. However, it is the combination of transcription factors and not individual factors which determines the specific pattern of gene expression which leads to differentiation into a particular cell type. In addition to transcription factors, a large number of extracellular growth factors influence cell proliferation and differentiation at defined stages in haematopoiesis. In vitro studies have identified more than 20 factors which can affect cellular activity in a positive or negative manner. Among the numerous growth factors, it is often difficult to identify those factors which exert specific effects on cell differentiation from those which promote lineage development. The activities of three important growth factors, GM‐CSF, macrophage colony‐stimulating factor (M‐CSF) and G‐CSF are well defined. The growth factor GM‐CSF is required for the development of most myeloid cells but in combination with G‐CSF it promotes only granulocyte formation from the common granulocyte‐monocyte progenitor. In combination with M‐CSF, GM‐CSF tends to stimulate differentiation of monocytes from the same progenitor cells. It is reported that, when stimulated by erythropoietin, the common myeloid progenitor cells respond by producing more megakaryocyte‐erythrocyte progenitor cells, resulting in increased erythrocyte production. As multipotential cells arise from stem cells during embryological development, many microenvironmental factors influence the movement of cells to particular sites in the embryo and ultimately into the tissues and organs in which they reside. A group of low molecular weight basic proteins, termed chemokines, have a central role in determining the locations within the embryo and later in the foetus, where particular cell types populate tissues and organs. There are approximately 40 of these basic proteins and, although a few are transmembrane proteins, the majority are secreted proteins. Chemokines possess four conserved cysteine residues and, based on the position of two of the four cysteine residues, most fit into two subgroups: CC subgroup chemokines in which the conserved cysteines are contiguous and CXC subgroup chemokines in which the conserved cysteines are separated by some other amino acid (X). The action of chemokines is mediated by receptors whose polypeptide chains span the membrane seven times. Receptors for chemokines are members of the G‐protein‐linked family and are grouped according to the type of chemokine which they bind. When receptors bind appropriate chemokines, a signal transduction process is initiated which has a marked effect on the activity of cells to which they bind. Chemokines exert important effects on particular cell types both during foetal development and after birth. They help to localise circulating cells to sites of inflammation and also to specific microenvironments in tissues. Chemokines have a diverse range of activities including the ability to exert chemotactic influences and to control adhesion and activation of mature and immature blood cells. Accordingly, they have a major role in regulating leukocyte traffic and in maintaining homeostasis. The chemokine CXCL12, which is expressed by stromal cells and its G‐protein‐coupled receptor (CXCR4) which is expressed on HSCs, are required for proper HSC functioning and migration in the developing foetus. Likewise, the cytokine SCF which is produced by stromal cells and its receptor KIT which is expressed on HSCs, are critical for the optimal functioning of HSCs. Stem cell factor–KIT (SCF‐KIT) signalling is reported to enhance the migration response of foetal HSCs to CXCL12. Additional factors which are reported to be important for HSC migration and establishment at other sites include α4 integrin, N‐cadhedrin and osteopoietin, membrane‐bound adhesion molecules which can activate Wnt signalling. Another factor, expressed by stromal cells, transcription factor pituitary homeobox 2, is required for the maintenance of foetal HSCs. When haematopoiesis in the murine foetus moves from the liver to the bone marrow at a gestational age of approximately 18 days, the circumstances which contribute to this change are attributed to the commencement of bone formation at approximately 12.5 days by osteoblasts and chondrocyte precursor cells. These cells are capable of forming a niche where HSCs can avail of a suitable microenvironment for growth and development. The signals which are reported to be involved in this relocation of haematopoietic activity include CXCL12–CXCR4, SCF–KIT, Tie‐2–angiopoietin, integrin and CD44–E‐cadhedrin adhesion and signalling pathways. These factors also appear to participate in the retention of HSCs within the bone marrow space. In mammals, the sites where HSCs reside include endosteum of bone and vascular niches in bone marrow and in some other tissues. The majority of HSCs in adult mice reside in osteoblastic and vascular niches in bone marrow with smaller numbers present in vascular niches in other tissues. During embryogenesis, in the absence of osteoblastic cells, HSCs arise from progenitor cells in perivascular sites; HSCs in extramedullary tissues such as liver and spleen are present in sinusoidal or vascular areas. Endosteal cells differentiate into osteoblasts which facilitate cell‐to‐cell contact with HSCs. Bone‐resorbing osteoclasts are also present in the endosteum and the competing activities of these two cell types influence HSC development. The maintenance of HSCs throughout life requires conditions which are conducive to the stability of these blood‐forming cells. In the absence of pathological changes affecting the bone marrow or damage to white blood cells or red blood cells by infectious agents or toxic factors, HSCs usually divide infrequently. In mice, it is reported that dormant HSCs divide every 145 days. If stimulated with granulocyte‐colony stimulating factor, dormant HSCs enter a cell cycle before returning to dormancy. Under stressful conditions, HSCs can undergo self‐renewal and subsequently revert to a quiescent state. Within the bone marrow microenvironment, signalling factors involved in the maintenance of quiescence include TIE2 tyrosine kinase receptor on HSCs, which interacts with angiopoietin on osteoblasts together with thrombopoietin and a number of other factors which promote a quiescent state. Experimental evidence supports the ability of HSCs to contribute to multiple cell lineages from which cells such as microglial cells, antigen‐presenting cells and Langerhans cells in the skin can arise. In addition to their role as blood‐forming cells, therefore, HSCs may have the inherent ability to contribute to a range of cells of different lineages, depending on the developmental pathways taken by progenitor cells originating in the bone marrow. Derivatives of HSCs may in some instances be lineage‐restricted and in other circumstances these cells may not be subject to lineage restriction. Stages in the maturation of lymphoid and myeloid cells are shown in Figures 15.5 and 15.6, respectively. A summary of important features of blood cells and associated cells in mammals is presented in Table 15.1. Figure 15.5 Origin, site of maturation and functional activity of lymphoid cells.
Embryological and postnatal features of haematopoiesis
Ontogeny of haematopoiesis
Sites of haematopoiesis in the developing embryo
Cellular activity and other factors in the adult bone marrow which influence HSC development and activity
Acellular factors involved in haematopoiesis
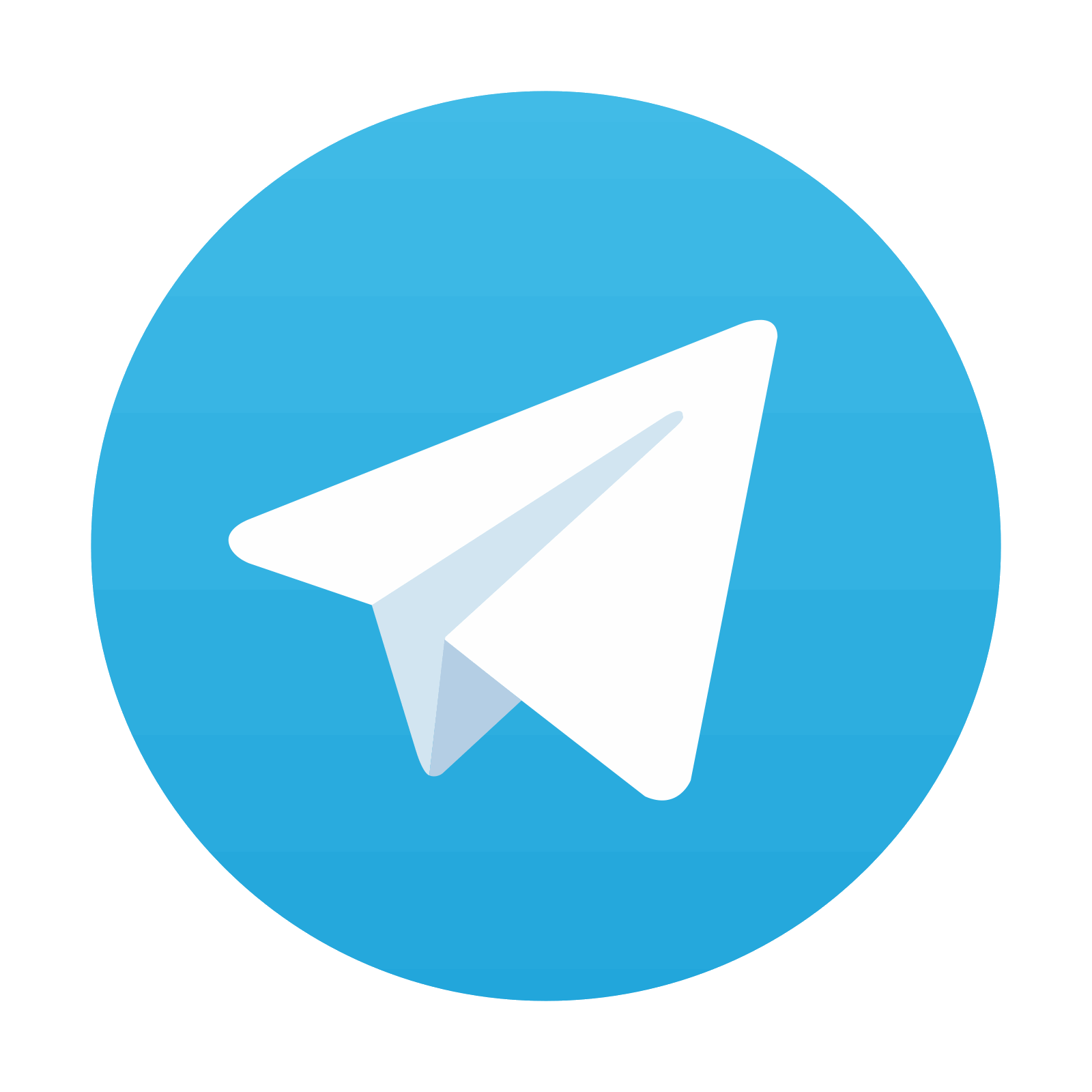
Stay updated, free articles. Join our Telegram channel
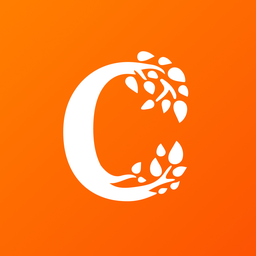
Full access? Get Clinical Tree
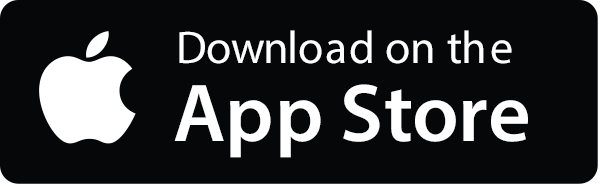
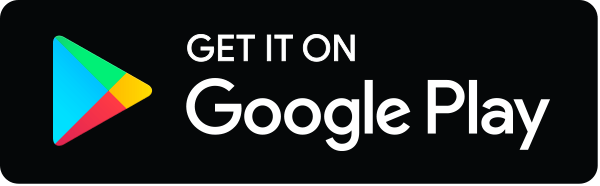