Fig. 1.
Preparations used for electrophysiological recordings. Schematic representation of some of the most common electrophysiological techniques. Top left: Acutely dissociated neuron with two recording electrodes and sample traces of excitatory (top inset) and inhibitory (bottom inset) currents evoked by the application of NMDA and GABA, respectively. Top right: Brain slice showing a patch electrode and recordings of spontaneous currents (lower inset) from a MSSN and another electrode recording evoked currents (upper inset) in the MSSN in response to stimulation of inputs from the cortex. Lower schematic: Single- or multiunit in vivo recordings can be performed in anesthetized or awake behaving animals. The inset shows a sample trace of an in vivo, single-unit extracellular recording.
2.1 Acute Brain Slices
The use of acute brain slices for electrophysiology has become widespread since it was first introduced in the 1960s (4). Brain slices can be obtained from a wide range of animal species and neural regions. Following removal of the brain and isolation of the region of interest, a vibrating microtome is used to obtain a thin slice (100–400 μm thickness) of brain tissue that can be used for electrophysiological experiments after 1–2 h of incubation to allow for neuronal recovery. The extracellular ionic environment can be precisely controlled and various drugs can be applied onto neurons. The local anatomy of the tissue is relatively intact, leaving microcircuits and therefore identification of subsets of local neuronal connections is possible. With the use of brain slices, it is also possible to record from two or more neurons simultaneously or from different parts of the same neuron such as the soma and dendrites. With the development of sophisticated imaging techniques, brain slices have become a versatile preparation for a variety of electrophysiological studies. The main shortcoming using brain slices is that, though the local cytoarchitecture is relatively preserved, there is loss of long-distance synaptic connections.
2.2 Acutely Dissociated Neurons
Neurons can be isolated from most regions of the brain using a combination of mild enzymatic digestion and gentle mechanical trituration (5). This method provides perhaps the most isolated preparation for relatively intact neurons. Also, because these acutely isolated neurons are free from cellular debris and ensheathing glia, the formation of gigaohm seals is facilitated in patch-clamp studies. Neurons obtained from this preparation are free of synaptic inputs and usually shorn of their dendritic processes except for the initial stumps of primary dendrites.
The advantage of using dissociated neurons is the absence of synaptic inputs, so intrinsic and postsynaptic changes in the neuron can be studied in relative isolation. The loss of dendrites reduces space clamp distortion of currents in voltage-clamp recordings but also leads to loss of information from ion channels and receptors located more peripherally on the dendrites.
2.3 Neuronal Cultures
Dissociated neurons and brain slices from many regions of the brain can be grown in culture for electrophysiological analysis (6–8). Since neurons in culture form a monolayer, they can be easily visualized and the extracellular solution in this preparation, as with that in acutely dissociated neurons, can be precisely maintained and quickly changed to record effects of various pharmacological manipulations. As these preparations have synaptic contacts, in addition to ionic currents, synaptic connectivity can be studied. Also, similar to acutely isolated neurons, the bare neuronal membranes facilitate gigaohm seal formation. Neurons from two regions of the brain can be co-cultured and they will form synaptic connections (9). Neuronal cultures are simple yet powerful tools to examine neuronal function; however, there are disadvantages. Though synaptic connections form in these preparations, they do not represent the total of all inputs the neurons receive. Neurons are maintained in an artificial environment in the absence of a majority of their influences from other neurons, hormones, and neurotransmitters and may have compensatory or abnormal properties that are not present in vivo. Finally, cultured preparations typically do not survive for long time periods minimizing the ability to evaluate long-lasting changes.
2.4 In Vivo Recordings
The field of electrophysiology has evolved to the stage where chronic in vivo recordings can be performed in awake animals in a variety of species. Using microwire electrodes, single- or multiunit extracellular recordings can be performed by surgically placing the electrode at desired coordinates inside the brain using a stereotaxic instrument. Action potentials in neurons are detected as small voltage fluctuations (0.1–1 mV). This technique can be coupled with iontophoresis and local drug administration, stimulation (e.g., electrical, chemical, light) of afferents, or antidromic activation of target areas. With the advent of advanced computer techniques, it has become possible to simultaneously record from large samples of single neurons distributed across multiple brain sites in fully awake and behaving animals over long periods of time to integrate changes in neuronal activity with behavioral alterations.
The obvious advantage of in vivo electrophysiology is that neurons can be studied in their natural milieu with their entire complement of inputs, targets, circulating hormones, and modulators. In vivo experiments either in awake or anesthetized preparations can be used to determine the effect of systemically administered drugs or physiological manipulations that might have several targets of action. On the other hand, it is much more difficult to precisely manipulate the local environment surrounding the cell groups under study in in vivo experiments. In awake, behaving animals there are several other variables such as behavioral state, stress, differences in training, and task ability that could affect the recorded activity.
3 An Overview of Electrophysiological Methods
3.1 Sharp Electrode Recordings
Classical electrophysiological techniques involve transmembrane recordings made using a fine-tipped “sharp” electrode inserted carefully inside the neuron. With this technique, a fine-tipped glass capillary filled with KCl or K-acetate is inserted into the cell to obtain transmembrane intracellular recordings. The potential difference between the tip of the electrode and a reference electrode placed in the bath provides the transmembrane potential of the neuron. When a constant or time-varying current is applied to a neuron and the change in membrane potential recorded, it is termed current-clamp recordings. This experimental protocol is more physiological than voltage-clamp recordings as it mimics the changes in membrane potential that a neuron undergoes in vivo in response to applied stimuli. Extracellular recordings can be obtained when the electrode is close to, but outside the neuron. In this configuration, the electrode records relatively large, fast electrical events (e.g., action potentials) from single neurons (single-unit) or groups of neurons (multiunit recordings) as well as slower time course events (field potentials).
Extracellular and intracellular recordings with sharp electrodes are relatively easy to perform. They are therefore the technique of choice for long-term recordings of synaptic plasticity like long-term potentiation (LTP) and long-term depression (LTD). However, due to the high electrical resistance of the pipettes, the recording conditions are not optimal because the cell membrane is typically damaged. Sharp electrode penetration leads to depolarization and sometimes injury discharge coupled with damage to the neuronal membrane which is recorded as a significant leakage resistance across the membrane of the recorded cell (10).
3.2 Patch-Clamp Recordings
In contrast to sharp electrode recordings in which an electrode is inserted through the cell membrane, the patch-clamp technique involves forming a high-resistance seal (gigaseal) by pushing a micropipette with a fire-polished tip against the cell membrane (11). Cells can be studied in current or voltage-clamp modes. In voltage-clamp mode, the membrane potential is held constant (holding potential) or a series of voltage commands, steps, or ramps are applied and the ionic currents through the membrane are recorded. Patch-clamp recording is a versatile method to study voltage- and time-dependent ionic conductances and their modulation by various pharmacological agents.
One of the main difficulties in performing patch-clamp recordings from brain slices is clogging of the patch pipette tip as it passes through the tissue overlying the neuron to be recorded within the slice. This problem can be surmounted by using imaging techniques like infrared (IR) videomicroscopy combined with differential interference contrast (DIC) optics and using a non fire-polished patch pipette to penetrate the slice and “clean” away the debris above the target neuron with a puff from the pipette solution (12, 13). Once the surface of the neuron of interest is clean, a gigaohm seal can be formed.
After formation of the gigaohm seal, currents from single ion channels can be recorded from a patch of the cell membrane in cell attached configuration or from single channels in a membrane patch isolated from the neuron in either inside-out or outside-out configurations. Following gigaohm seal formation, rupturing the cell membrane spanning the micropipette tip enables current recordings from all ion channels in the entire cell membrane and this configuration is termed whole-cell recording. The patch-clamp technique enables recordings from a wide variety of cell types and allows measurements of currents in the picoampere (pA) range. One of the drawbacks of this technique is that the internal milieu of the recorded cell is altered depending on the pipette solution used. Addition of gramicidin or nystatin in the pipette (perforated patch) can circumvent this problem by allowing movement of some small monovalent ions and preserving most of the ionic and second messenger constituents of the cell.
3.3 Electrotonic Potentials and Dye-Coupling
Besides chemical synaptic transmission between neurons, there exists a form of communication between neurons mediated by gap junctions termed electrical coupling. This can be investigated at the same time that intracellular or patch-clamp recordings are performed by including a low molecular weight dye like Lucifer Yellow (LY) in the pipette. LY crosses gap junctions and neurons that are connected by gap junctions appear “dye-coupled” in that more than one neuron is labeled even though the activity of only one neuron was recorded.
3.4 Combining Patch-Clamp and Optical Imaging Techniques
The versatility of the patch-clamp technique enables it to be combined with a variety of imaging techniques. These techniques include imaging of ions, especially Ca2+, in living cells. The cytosolic Ca2+ level and its changes can be visualized using fluorescent, Ca2+ binding dyes, such as Fura-2 delivered to the cell in the patch pipette. Photobleaching and uncaging of compounds in localized regions are also associated techniques for visualization of ions. Fluorescent proteins like green, yellow, and red fluorescent proteins (GFP, YFP, and RFP, respectively) are powerful tools to label neurons. The fluorescent protein can be targeted to specific neurons to identify them in a preparation that permits visualization. The temporal resolution of electrophysiology can be combined with the spatial resolution of imaging techniques to define spatiotemporal characteristics of individual neurons and networks (14). The two techniques can be combined in a wide array of configurations. Electrophysiological recordings of synaptic inputs from the soma of a neuron can be correlated with the location of the input in the dendritic tree by measuring synaptically activated Ca2+ signals in the dendritic arbor. By simultaneous imaging activity in the local network using a fluorescent Ca2+ probe, reverse (presynaptic) or forward (postsynaptic) connectivity of an electrophysiologically recorded neuron can be obtained (14).
3.5 Selective Neuronal Ablation
The ability to selectively inactivate specific neurons or populations of neurons in vitro and in vivo provides a valuable tool to understand specific neuronal functions and the neuronal circuit as a whole. There are various methods for rapidly inducing and reversing modulation of neuronal excitability and neurotransmitter release (15). The allatostatin receptor (AlstR) system is based on the expression of the Drosophila AlstR that is selectively activated by the insect peptide hormone allatostatin. Coupling of allatostatin to AlstR leads to opening of a G protein-coupled inwardly rectifying K+ channel that causes hyperpolarization and suppression of action potential firing (16). A second system to selectively silence neurons is the ivermectin-sensitive chloride channel. This involves co-expression of two subunits (α and β) of a Caenorhabditis elegans glutamate- and ivermectin-gated chloride channel (GluClαβ) that is activated by ivermectin and results in a chloride current that suppresses neuronal activity. For example, in vivo, expression of GluClαβ unilaterally in the striatum and administration of ivermectin leads to perturbation of amphetamine-induced rotational behavior (17). The MIST (Molecular Systems for the Inactivation of Synaptic Transmission) system blocks neurotransmission by interfering with the function of presynaptic proteins like synaptobrevin, synaptophysin, and syntaxin (18). Diphtheria toxin can also be used to remove neurons. For example, when the diphtheria toxin receptor and diphtheria toxin injection ablated selectively dopamine (DA) D2 receptor-containing neurons in the entire striatum hyperlocomotion occurred, whereas more selective ablation in the nucleus accumbens increased amphetamine conditioned place preference (19).
3.6 Optogenetics
Although electrical simulation has been the standard method for activation of neurons and fiber tracts, new techniques combining genetic expression of light-sensitive channels in selected neuronal populations and stimulation with light have been developed. These optogenetic approaches permit rapid stimulation and inhibition of genetically targeted neuronal populations on a millisecond timescale. This is achieved by genetically expressing the microbial opsins Chlamydomonas reinhardtii Channelrhodopsin-2 (ChR2) and Natronomonas pharaonis halorhodopsin (NpHR) in neurons (20, 21). ChR2 is a cation channel that is activated by ∼470 nm blue light and NpHR is a chloride pump activated by ∼580 nm yellow light. By varying the wavelength of light used, ChR2 and NpHR can be activated independent of each other to evoke action potential firing or inhibit neuronal activity, respectively (22). The activity of specific neuronal subtypes can be modulated by targeting these opsin proteins to the neurons of interest. While brain slices with ChR2- and NpHR-expressing neurons can be studied, an important application of optogenetics is the ability to express and study these proteins in intact animals. Fiber optic-based systems have been developed to deliver light to precise ChR2- or NpHR-expressing neuronal targets in superficial and deep brain structures (23, 24).
4 Alterations in Cellular and Network Electrophysiological Properties in Models of Movement Disorders
A significant number of electrophysiological studies have been performed on brain slices obtained from rodent models of HD and PD (25–28) (Table 1). These studies have revealed important changes in passive and active membrane properties, as well as synaptic transmission, as the disease progresses. Of major interest in these studies, specific changes in DA D1 and D2 receptor-expressing medium-sized spiny neurons (MSSNs) of the direct and indirect striatal output pathways, respectively, can now be identified by using mice that express the EGFP gene under the control of the promoter for either DA D1 or D2 receptors. In these mice, D1 and D2 DA receptor neurons can be individually identified and the mice can be crossed to genetic models of HD or PD as well as determining the effects of DA depletion (29, 30).
Table 1
Summary of electrophysiological outcomes in models of HD and PD using different approaches
Preparation | HD | Reference |
---|---|---|
Dissociated neurons and cultures | Decreased NMDAR currents and increased NMDAR Mg2+ sensitivity in cortical pyramidal neurons Increased NMDAR currents and decreased NMDAR Mg2+ sensitivity in striatal MSSNs Increased voltage-activated Ca2+ currents in cortex Decreased voltage-activated Ca2+ currents in MSSNs Decreased K+ channel inward rectification in MSSNs Imbalance of synaptic and extrasynaptic NMDAR signaling leading to toxic extrasynaptic NMDAR stimulation and cell death | (37) (65) (37) (31) (36) (67) |
Brain slices | Alterations in synaptic activity in the cortex – increased spontaneous EPSC frequency and evoked EPSC amplitude; decreased spontaneous IPSC frequency Alterations in synaptic activity in the striatum – decreased spontaneous EPSC frequency and increased IPSC frequency Impaired LTP at CA1 and dentate granule cell synapses in the hippocampus Impaired LTD in hippocampus Aberrant LTD in cortex Abnormal striatal synaptic plasticity-loss of depotentiation in MSSNs and lack of LTP in cholinergic interneurons Elevated extrasynaptic NMDAR activity Differential changes in D1 and D2 MSSN glutamate neurotransmission and dopamine modulation | (41) (58) (59) (41) (61) (69) (70) |
In vivo | Increased firing rate and decreased correlated firing between neurons both in the cortex and in striatum |
Preparation | PD | Reference |
---|---|---|
Brain slices | Increased spontaneous glutamatergic activity Increased GABAergic IPSCs in cortex and decrease in striatum Impaired hippocampal synaptic responses to prolonged trains of repetitive stimulation Decreased glutamate release in CA1 region of hippocampus | (46) (55) (57) |
Deficits in excitatory transmission in the striatum Short-term and long-term synaptic plasticity affected in corticostriatal pathway Increased activity of indirect pathway MSSNs and decreased activity of direct pathway MSSNs Attenuation of collateral connectivity between MSSNs | (2) (56) (33) (51) |
4.1 Passive Membrane Properties
Intracellular and patch-clamp recordings in slices have provided useful information regarding changes in passive membrane properties such as cell capacitance and input resistance in mouse models of HD. These properties correlate with cell surface characteristics exemplified by total surface area as well as the number of conducting channels in the membrane. In striatal MSSNs, there is an increase in input resistance, which probably reflects the progressive loss of conductive channels (31, 32). Another observation is reduced cell capacitance in these neurons, an electrophysiological observation that has been supported by morphological evidence (32). In models of PD in which DA has been depleted chronically, differential alterations occur in passive membrane properties of MSSNs originating the direct or indirect pathways (those expressing D1 or D2 DA receptors, respectively). Neurons of the indirect pathway show no change in cell capacitance or input resistance. However, D1 receptor-expressing MSSNs have a decreased capacitance and a higher input resistance compared to controls indicative of changes in the direct pathway output (33).
4.2 Ionic Currents
Whole-cell recordings in voltage-clamp mode have been used to examine ionic currents mediated by the movement of Na+, K+, Ca2+, and Cl− through neuronal channels. Na+ currents are mediated by movement of the ion through voltage-gated Na+ channels and are critical for action potential generation. Na+ also enters the cell through nonspecific cation channels that are ligand-gated (e.g., α-amino-3-hydroxyl-5-methyl-4-isoxazole-propionate (AMPA) and N-methyl-d-aspartate (NMDA) receptors). In the latter case, though these channels are permeable to both Na+ and K+ (34), binding of the ligand to the receptor channel leads to predominant Na+ entry because the resting membrane potential (RMP) of most neurons is close to the equilibrium potential for K+. Possible sources of Ca2+ currents in neurons are Ca2+ entry into the neuron through voltage-activated Ca2+ channels and NMDA receptor channels. Similarly, there are voltage-activated and ligand-activated K+ channels and also Ca2+-activated K+ channels. Ca2+ also can be mobilized by changing the binding properties of intracellular Ca2+ stores. The anionic Cl− current is predominantly caused by synaptic activation of γ-aminobutyric acid-A (GABAA) receptors leading to membrane hyperpolarization. In very young animals, however, due to a Na+-K+-Cl− cotransporter, the reversal potential for Cl− is relatively depolarized (−35 mV) and GABA release is depolarizing and capable of producing excitation instead of inhibition (35).
Numerous alterations in ionic conductances have been observed in current and voltage-clamp recordings from MSSNs in slices from the R6/2 mouse model of HD leading to a depolarized RMP, a lower rheobase, and an alteration in action potential firing (32). In addition, decreases in voltage-activated Ca2+ currents and K+ channel inward rectification occur (31, 32). In parallel to the decrease in inward and outwardly rectifying K+ currents, MSSNs in R6/2 mice show decreased expression of K+ channel subunit proteins Kir2.1, Kir2.3, and Kv2.1 (36). Attenuation of K+ conductances may contribute to altered electrophysiological properties of MSSNs and their selective vulnerability in the striatum. In contrast to the striatum, voltage-activated Ca2+ currents are increased in cortical pyramidal neurons in symptomatic R6/2 mice, indicative of complex changes that could effectively increase cortical excitability and alter corticostriatal function (37).
4.3 Synaptic Activity
In the absence of external electrical stimuli, neurons display spontaneous membrane currents, which can be either excitatory or inhibitory. These spontaneous excitatory and inhibitory postsynaptic currents (EPSCs or IPSCs, respectively) can be recorded in voltage or current-clamp mode and provide information related to both presynaptic and postsynaptic elements. Even in the absence of presynaptic action potentials, neurotransmitter vesicles are occasionally released into the synapse and they generate miniature (m)EPSCs or mIPSCs. Miniature currents can be recorded by isolating the neuron using tetrodotoxin, a Na+ channel antagonist, which blocks action potentials in neurons. A condition or compound that affects presynaptic neuron firing will affect spontaneous currents but not miniature currents. Changes in presynaptic transmitter release or the function of postsynaptic receptors are reflected by changes in frequency and amplitude of miniature currents. Another method to record evoked EPSCs and IPSCs is by electrically stimulating the inputs to the neuron.
Using mouse models of HD, time- and region-dependent changes in synaptic transmission in the corticostriatal pathway have been demonstrated. In the R6/2 mouse model of HD, dysregulation of glutamatergic inputs occurs early (5–7 weeks) and is manifested by the presence of large amplitude and complex synaptic events in the striatum (38). In parallel, there is a progressive decrease in spontaneous EPSCs and increase in the stimulus intensity required to evoke EPSPs in MSSNs (32, 38). These findings suggested a progressive disconnection of the cortex from the striatum (25). In addition, there is an increase in spontaneous GABAergic IPSCs in the striatum of these mice possibly leading to reduced output of MSSNs to their targets (39, 40). Large amplitude synaptic events in striatum suggested cortical hyperexcitability in R6/2 mice. In agreement, in the cerebral cortex, a progressive increase in frequency of spontaneous EPSCs in pyramidal neurons is observed (41). In contrast, the frequency of cortical spontaneous IPSCs initially increased and subsequently decreased in fully phenotypic mice. This imbalance leads to increased cortical excitability and is manifested by enhanced seizure susceptibility in R6/2 animals (41).
< div class='tao-gold-member'>
Only gold members can continue reading. Log In or Register a > to continue
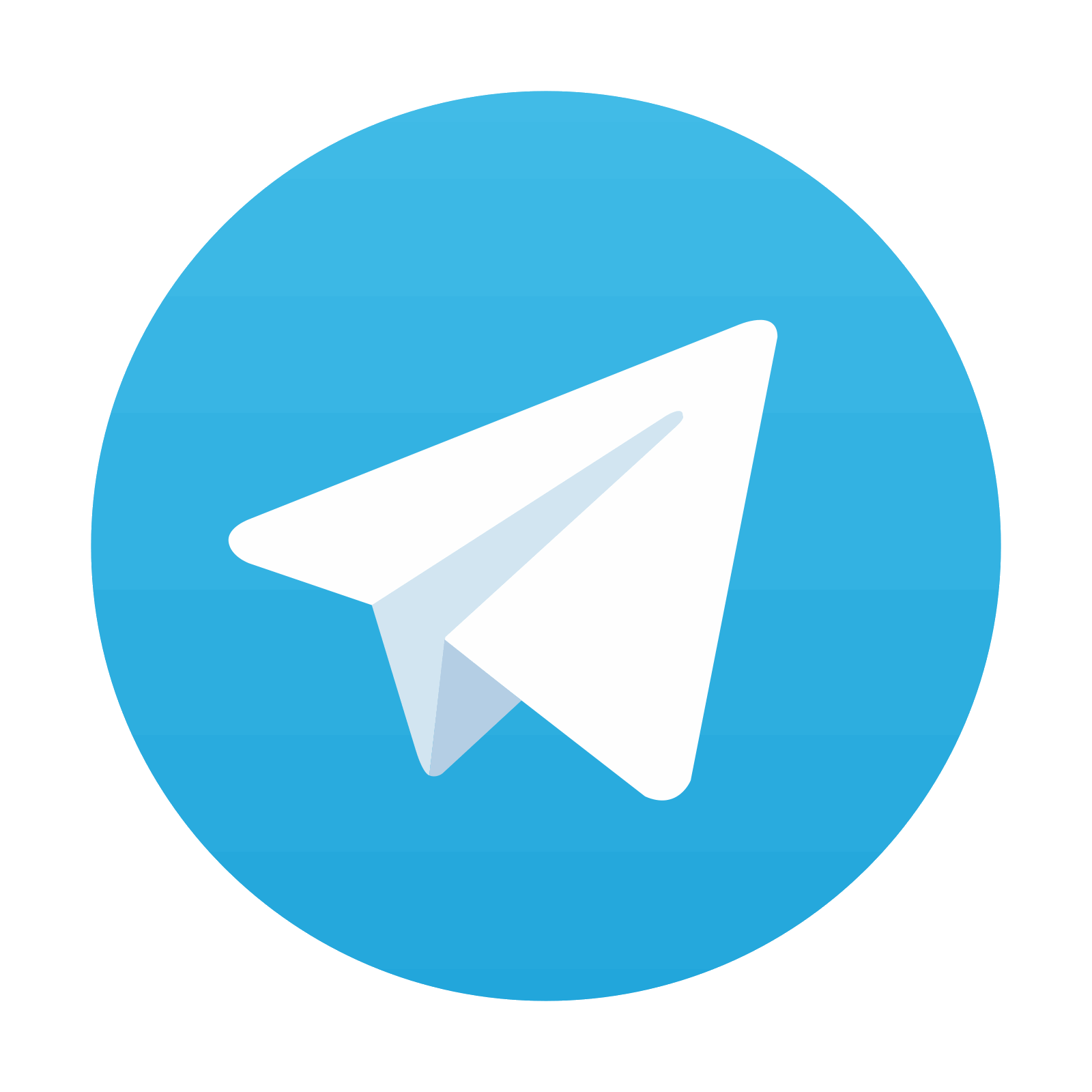
Stay updated, free articles. Join our Telegram channel
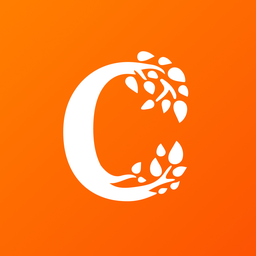
Full access? Get Clinical Tree
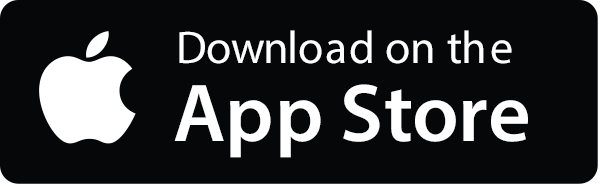
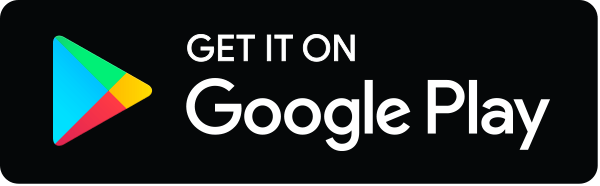