Fig. 15.1
Experimental models of circadian desynchronization. Schematic representation of behavioral rhythms under different environmental conditions as observed in the laboratory. Light-dark cycles different from 24 h (i.e., T-cycles) whose periods (T) are set within the entrainment range are normally compensated by the circadian clock. (a) When T is longer than the circadian period (τ), entrainment occurs by phase-delays (−Δϕ). As predicted by the phase response curve (PRC), light pulses tend to be aligned at the beginning of the subjective night (i.e., locomotor activity onset). (b) An advancing T-cycle is imposed with T-cycles shorter than 24 h, generating phase-advances (+Δϕ) of the clock. Stable entrainment can be challenged by an abrupt shift in the phase of the 24 h LD cycle, i.e., a jet lag simulation. Indeed, either a delay (c) or an advance (d) of the LD cycle will impose a delaying or advancing light-input to the clock, respectively, which will gradually entrain to the new conditions. Finally, chronic jet lag schedules (e, f) or T-cycles outside the entrainment range (g, h) (see Casiraghi et al. 2012), are used in the laboratory to study the circadian system under extreme entraining conditions. While the clock can entrain to gradual changes in the LD cycle, when animals are subjected to frequent phase advances of the LD cycle, relative coordination and/or forced desynchronization of behavioral rhythms can be observed
Table 15.1
Observed effects on health of protocols of circadian disruption and circadian genes mutations in animal models
Model | Observed alterations | References |
---|---|---|
Rats under CJL 8/2 | • Accelerated development of osteosarcoma and hepatocarcinoma tumors • Altered locomotor activity, temperature, and corticosterone rhythms | |
Mice under CJL 8/2 | • Fast development of Lewis lung and lung metastasis | Wu et al. (2012) Hadden et al. (2012) |
• Altered lung mechanics | ||
Mice under CJL 4/1 | • Increased body weight • Altered blood levels of leptin and insulin • Decreased prefrontal cortex neurons complexity and impaired cognitive abilities | Karatsoreos et al. (2011) |
Rats under CJL 6/2 | • Accelerated growth of lung tumors • Disrupted rhythms of NK cells function | Logan et al. (2012a) |
Mice under CJL 6/2 | • Increased body weight and diverse metabolic alterations | Casiraghi et al. (unpublished) |
wt, cry1/2−/−, per1/2−/− mice under CJL ± 8/3 | • High rates of spontaneous and γ-radiation-induced tumors • Hyperplasia of the salivary and preputial gland. • Spontaneous lymphoma development and liver and ovarian cancer | Lee et al. (2010) |
p53−/− mice under CJL ± 8/3 | • Liver and salivary gland hyperplasia • Accelerated development of lymphoma and osteosarcoma • Kidney failure | Lee et al. (2010) |
Hamsters under CJL 6/3 | • Decreased hippocampal neurogenesis • Impaired learning and memory formation | Gibson et al. (2010) |
HIP rats under CJL 6/3 | • Increased beta cell apoptosis and diabetes development | Gale et al. (2011) |
Mice under CJL 6/7 | • Increased lipopolysaccharide induced mortality and cytokines expression | Castañon-Cervantes et al. (2010) |
Aged mice under CJL 6/7 or 6/4 | • Lifespan shortened | Davidson et al. (2006) |
Rats under CJL 6/7 | • Reduced hippocampal neurogenesis | Kott et al. (2012) |
Mice under Chronic LD inversions | • Accelerated growth of carcinoma and sarcoma tumors | Li and Xu (1997) Preuss et al. (2008) |
• Dramatic worsening of dextran sulfate-induced colitis | ||
Rats under LL | • Higher rates of hepatocarcinoma development | van den Heiligenberg et al. (1999) |
Rats chronically exposed to light at night | • Accelerated aging process • Increased spontaneous tumor development | Vinogradova et al. (2010) |
• Faster xenograft tumor growth | Blask et al. (2009) | |
Mice chronically exposed to light at night | • Increased body weight • Impaired glucose tolerance | Fonken et al. (2010) |
Rats forced to be active during light phase | • Disrupted rhythms of plasma glucose, TAG, and corticosterone • Increased weight gain | |
SCN lesion in mice | • Accelerated development of osteosarcoma and adenocarcinoma tumors | Filipski et al. (2003) |
tau mutant hamster | • Cardiac and renal disease | Martino et al. (2008) |
per1 (Brd) mutant mice | • Altered glucocorticoid rhythms | Dallmann et al. (2006) |
bmal−/−, clock mutant mice | • Altered glucose regulation | Rudic et al. (2004) |
mper2 mutant mice | • Accelerated tumorigenesis | Wood et al. (2008) |
• Increased cancer development after γ-irradiation | Fu et al. (2002) | |
Clock mutant mice and mper1/2/3 triple deficient mice | • Symptoms of metabolic syndrome | Turek et al. (2005), Dallmann and Weaver (2010) |
cry1/2−/−, per1/2−/− mice | • Increased spontaneous and radiation-induced tumorigenesis | Lee et al. (2010) |
15.2.1 Exogenous Disruption of Circadian Rhythms
15.2.1.1 Alterations Related to Human Activities
Jet lag
Transmeridian flights produce a mismatch between the circadian timing at destination respect to that of the departure location or, in other words, a transient desynchronization between internal and external time. Depending upon the time zones crossed, travelers might experience an inversion of the LD cycle with respect to their normal sleep schedule. This will result in conflictive light information appearing at the subjective night, forcing the circadian clock to be gradually re-entrained to the new local LD schedule. During the immediate days after arrival, a disruption of the sleep–wake pattern is generated, with decreasing alertness and increasing fatigue during the day, and even gastrointestinal symptoms (Sack 2009). In addition, internal desynchronization between physiological rhythms might persist for several days (Harrington 2010; Sellix et al. 2012). As a general rule, it typically requires one day to adjust for each time zone crossed, but more days to re-entrain are needed when traveling in the Eastward direction. The extent and severity of jet lag can be also worsened by age and affected by a host of personal and experiential factors (Arendt 2009; Waterhouse et al. 2007). Although jet lag is a temporary inconvenient for occasional travelers, it can be a health concern for frequent travelers as airline workers if flight schedules are not planned considering circadian factors, as well as enough time to allow for sleep recovery.
Social Jet lag
Humans exhibit large differences in their temporal preferences (i.e., the “chronotype”), with extreme morning larks and evening owls and most people falling in between these extremes (Roenneberg et al. 2012). However, social schedules impose temporal demands on most individuals without considering their circadian preferred timing. This alteration in the phase-relationship between the activity–rest rhythm (synchronized by social zeitgebers) and the sleep–wake rhythm (synchronized by the circadian clock) is called “social jet lag” (Foster 2012; Wittmann et al. 2006). Population studies indicate that sleep timing in late chronotypes is subjected to the largest differences between work and free days (Wittmann et al. 2006). In addition, social jet lag can be a risk factor for several health troubles, including depression (Levandovski et al. 2011) and obesity (Roenneberg et al. 2012).
Shift work
The International Labour Organization defines working in shifts as “a method of organization of working time in which workers succeed one another at the workplace so that the establishment can operate longer than the hours of work of individual workers” (IARC 2010). Several types of shift work exist and can be described as follows: (a) permanent: people work regularly on one shift only, i.e. morning or afternoon or night; or rotating: people alternate more or less periodically on different shifts; (b) continuous: all days of the week are covered; or discontinuous: interruption on weekends or on Sundays; (c) with or without night work: the working time can be extended to all or part of the night, and the number of nights worked per week/month/year can vary considerably. Moreover, the definition of “period of night work” varies from country to country, i.e., in some countries it ranges from 8, 9, or 10 pm to 5, 6, or 7 am, and in many others from 11 or 12 pm to 5 or 6 am (IARC 2010). Types of shift work that affect normal rest hours will interfere with the circadian and homeostatic regulation of sleep, leading to cardiovascular disease, accidents, and even cancer (Knutsson 2003). It is generally observed that although most shift workers can behaviorally adapt in order to be awake at night and asleep during the day, they manifest reduced alertness and sleep efficiency (Akerstedt and Wright 2009). Furthermore, the majority of shift workers do not display a corresponding shift in core temperature and melatonin rhythms (Folkard 2008).
Nocturnal Lighting
Light pollution during the night is a feature of the industrialized urban environment where the major of human population resides. Light at night might induce acute effects in the human, including alterations in sleep, alertness, and melatonin levels. Recent work indicates that even low levels of illumination at night can reset circadian phase and suppress melatonin release in humans (Gooley et al. 2011; Zeitzer et al. 2000), suggesting that the relatively common exposure to artificial light may in fact affect circadian function. Even the use of low levels of artificial lighting at home and work may alter circadian function. These considerations are of importance since exposure to chronic nocturnal lighting will occur in shift work with nighttime work. However, the use of artificial light at circadian night in humans might compensate photoperiod shortening in higher latitudes, reducing depression symptoms in people suffering seasonal affective disorder (SAD) (Howland 2009).
15.2.1.2 Exogenous Alterations in the Laboratory
Acute and chronic jet lag schedules, constant light conditions and counter-phase forced activity, which may be considered model paradigms for shift- and night-work conditions in humans, as well as rodent circadian mutants, have been employed to study circadian disruption and its consequences on physiology, health, and disease development (see Fig. 15.1).
Simulated Jet Lag
Experimental jet lag is a standard protocol designed for studying circadian re-entrainment dynamics in the laboratory, by advancing or delaying the LD cycle (simulating Eastward or Westward flights, respectively). LD-advancing schedules usually require more days for the re-entrainment of circadian rhythms both in humans (Aschoff et al. 1975) and rodent models (Reddy et al. 2002; Yamazaki et al. 2000). In murine models, simulated jet lag induces transient internal desynchronization at all physiological levels. At the SCN clock, shifts in the LD cycle produce transient desynchrony between the dorso-medial and the ventro-lateral regions, revealing differential capabilities of each region to resynchronize to the new LD cycle (Davidson et al. 2009; Nagano et al. 2003; Nakamura et al. 2005; Sellix et al. 2012). LD-shifts also lead to uncoupling between electrical activity and clock gene expression in the SCN (Reddy et al. 2002; Vansteensel et al. 2003). Outputs downstream from the SCN show a differential re-entrainment rate according to its relationship with the activity–rest cycle; for example, the pineal melatonin peak takes 5 days to re-entrain after an 8 h LD-advance of the LD cycle (Drijfhout et al. 1997). Moreover, shifts in the LD cycle also produce a desynchronization between peripheral oscillators and the SCN, with differential clock gene expression pattern and speed of re-entrainment between organs (Davidson et al. 2009; Kiessling et al. 2010; Yamazaki et al. 2000). Of particular attention is the role of the adrenal circadian clock which regulates the speed of resynchronization after advances of the LD cycle through glucocorticoid secretion. Indeed, chronopharmacological manipulation of glucocorticoid secretion accelerated re-entrainment to the new LD cycle in mice (Kiessling et al. 2010), suggesting a possible therapy for ameliorating the effects of jet lag.
In addition, we have developed a chronic jet lag schedule consisting of a 6 h LD-advance each two days, which generated a stable desynchronization of two activity components in mice (Casiraghi et al. 2012) and exerted severe metabolic alterations (unpublished results). Moreover, animals under similar chronic jet lag protocols showed biphasic corticosterone rhythms (Filipski et al. 2004), attenuated AVP and Pk2 expression in the SCN (Yan 2011), and alterations in PER2 expression rhythms in the SCN, thymus, and liver (Castañon-Cervantes et al. 2010). These persistent alterations due to chronic desynchronization have important consequences in health (see below) and might reflect the risks to which individuals working in frequent rotating shifts are subjected.
Experimental Shift Work
The physiological effects of working schedules involving night shifts have been assessed in an animal model in which rats were forced to stay awake and active for 8 h during their resting phase (i.e., lights-on period) for five consecutive days, alternating with two “weekend” days with no disturbance. Rats under this protocol exhibited a progressive decrease of their nocturnal activity, and a phase shift in food intake and in circulating triacylglycerols rhythm toward the working hours (Salgado-Delgado et al. 2008). In addition, this schedule induced uncoupling between rhythms in hypothalamic nuclei involved in metabolic homeostasis, wakefulness, and arousal (which are shifted by the shift-work protocol), and the SCN, which remained locked with the LD cycle (Salgado-Delgado et al. 2010b). This internal desynchrony might contribute to the metabolic disorders that arises in both animals subjected to this type of protocols and workers under shifting schedules, which are discussed later.
LD Cycles Outside the Range of Entrainment
LD cycles with a T period (i.e., T cycles) can be experimentally set outside the entrainment limits, where the τ − T difference is not normally compensated with a given phase-relationship (Plano et al. 2010; Schwartz et al. 2011). Classical experiments in humans used these T-cycle protocols in order to evidence endogenous circadian regulation (Aschoff and Wever 1981). In rats, imposing a T cycle of 22 h outside the limits of entrainment led to the appearance of two rhythmic components of locomotor activity with different periods, one coincident with the LD period, and the other free running with a period >24 h (Campuzano et al. 1998). Each component was linked with clock gene expression in different regions of the SCN: the light-entrained component was related to the ventro-lateral region and the free running component to the dorso-medial region (de la Iglesia et al. 2004; see Fig. 15.2c). Moreover, under this desynchronization paradigm several physiological variables, such as temperature rhythms, rapid-eye movement sleep, and melatonin rhythms could be uncoupled from the LD scheme (Cambras et al. 2007; Lee et al. 2009; Schwartz et al. 2009).
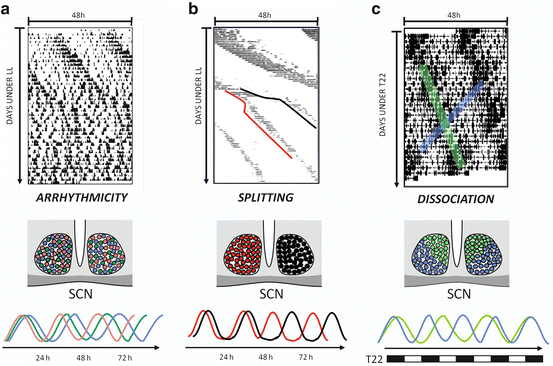
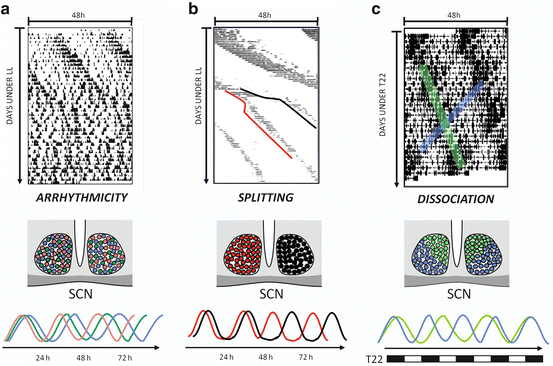
Fig. 15.2
Behavioral models of desynchronization: effects in the SCN. (a) Constant-light (LL) induced behavioral arrhythmicity in the mouse. Individual SCN cells (depicted in different colors in the middle-panel cartoon and in the representation of their activity in the lower panel) express their own rhythmic pattern, with different periods and phases, which results in an arrhtyhmic output such as locomotor activity (after Ohta et al. 2005). (b) Under chronic LL schedules, hamster might exhibit “split” locomotor activity rhythms, with two independent components of different circadian periods. These components represent the activity of each SCN which tend to be active in opposite phase to each other (middle and lower panels) (after De la Iglesia et al. 2000). (c) Rats under a T = 22 cycle might develop a spontaneous dissociation into two locomotor activity components, one following the T cycle and the other one related to the endogenous clock. This reflects the activity of the dorsomedial (depicted in green) or ventrolateral (blue) areas of the SCN (middle and lower panels) (after De la Iglesia et al. 2004)
Abnormal Lighting
Chronic light exposure has been shown to induce internal desynchrony of the activity-rest and core body temperature rhythms (Aschoff 1965). In nocturnal rodents, constant lighting lengthens circadian period, and when prolonged it can cause arrhythmia of behavioral (Chiesa et al. 2010) hormonal (i.e., corticosterone, Waite et al. 2012) and physiological rhythms with desynchronized SCN neurons (Ohta et al. 2005; see Fig. 15.2a). Moreover, in some animals subjected to constant light, activity rhythms become “splitted” into two components separated 12 h from each other, and each of them correlated with left- or right-sided SCN clock gene oscillations (de la Iglesia et al. 2000; Ohta et al. 2005; see Fig. 15.2b). Recent studies have emphasized the effects of nocturnal light pollution by providing rodents with low levels of light at night (typically ~5 lux) under an otherwise normal LD cycle (Bedrosian et al. 2011; Fonken et al. 2010).
In humans, light-pulses at night induced acute melatonin suppression and subjective sleepiness in a dose-dependent response to light exposure duration (Chang et al. 2012).
15.2.2 Endogenous Alterations in Human and Animal Models
Correct entrainment of the circadian clock can be disturbed due to genetic alterations, producing sleep-wake disorders. For instance, in advanced sleep-phase syndrome, specific mutations in the human clock gene Per2 and in Casein kinase Iδ (CKIδ) leads to daytime somnolence and difficulties to sleep and night. These problems arise due to the misalignment between the internal clock and environmental conditions, with persistent 3–4 h advanced sleep onsets and awakening times relative to conventional and desired times (Dauvilliers and Tafti 2008; Reid and Zee 2009; Toh et al. 2001; Xu et al. 2005). The alteration of the activity of CKIε and CKIδ produce changes in the period of the circadian rhythms in mammals (Lowrey et al. 2000; Meng et al. 2008; Ralph and Menaker 1988; Xu et al. 2005) due to the accumulation of PER-CRY dimers (Akashi et al. 2002; Eide et al. 2002; Lee et al. 2001).
Current treatment for this syndrome includes phototherapy and the use of chronobiotics such as melatonin (or its analogs ramelteon and tasimelton) or armodafinil (Hirai et al. 2005; Revell and Eastman 2005; Zisapel 2001). The hamster is an animal model for this syndrome and presents a single autosomal mutation in the enzyme CKIε, which leads to a shorter period in activity (Ralph and Menaker 1988) and endocrine rhythms (Lucas et al. 1999) from 24 to about 20 h. This mutation accelerates the degradation of both nuclear and cytoplasmatic PER proteins (Dey et al. 2005).
Another disorder associated with endogenous factors is the delayed sleep phase syndrome, which has been associated with a polymorphism in PER3 (Archer et al. 2003; Ebisawa et al. 2001; Pereira et al. 2005). In this syndrome the timing of the sleep episode occurs later than desired and is associated with problems to fall asleep and awakening in the mornings (e.g., to meet work or school obligations), and daytime sleepiness, with a particularly higher prevalence among adolescents and young adults (Okawa and Uchiyama 2007). Current treatments for this disorder include melatonin administration and bright light exposure during the morning. In addition, it should be noted that mouse mutants of other clock genes, such as Clock, Cry1, Cry2, Npas2, Dbp, and Bmal1, also have alterations in sleep duration and/or homeostasis (Reviewed in Takahashi et al. 2008), adding examples in which circadian clock genes can clearly affect the circadian and homeostatic control of sleep.
15.3 Health Consequences of Circadian Disruption
Misalignment between circadian oscillators and the 24 h LD cycle promote a wide set of health alterations, in general dominated by disruptions in the sleep–wake pattern. A recent study in humans shows that insufficient sleep at night generates circadian alterations in multiple genes in the blood transcriptome, including those associated with circadian rhythms (e.g., Per1–3, cry, clock, rora), sleep homeostasis (e.g., interleukin-6), oxidative stress (peroxyredoxin 2 and 5), and metabolism (e.g., ghrelin, glucose transporter 2, glucose/fructose transporter 5, cholesterol efflux regulatory protein), thus affecting several processes including chromatin modification, gene expression, macromolecular metabolism, and inflammatory, immune and stress responses (Moller-Levet et al. 2013). Indeed, several of such alterations depend on the deynchrony between the central (SCN) and peripheral clocks, as suggested in Fig. 15.3. Moreover, chronic desynchrony, such as the one observed in shift work schedules, might result in profound health disorders, as represented in Fig. 15.4.
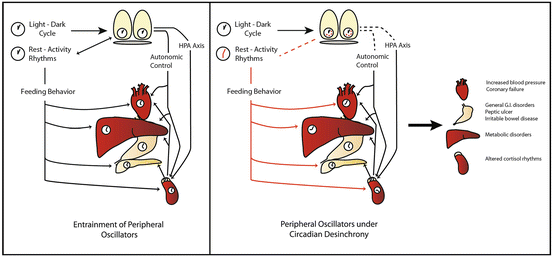
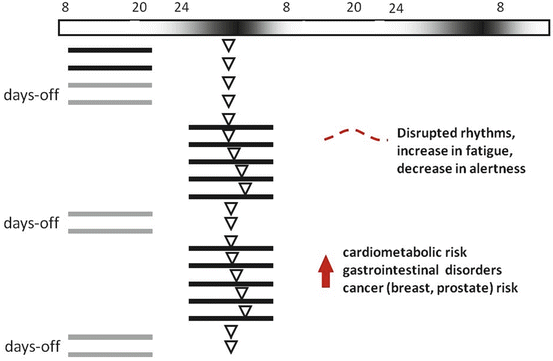
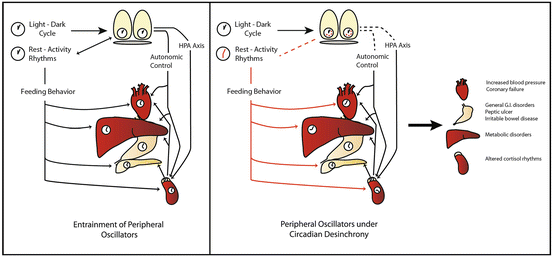
Fig. 15.3
Circadian desynchronization in peripheral organs. Left panel: Schematic representation of circadian entrainment in peripheral tissues. The suprachiasmatic nuclei (SCN) are entrained by photic cues, while peripheral organs receive time cues from the SCN through the autonomous nervous system and the hypothalamo–pituitary–adrenal (HPA) axis. In addition, food availability acts as a strong zeitgeber in several organs. Right panel: Schematic representation of the effects of circadian desynchrony in peripheral organs and its consequence in health status. Under circadian desynchronization, such as shift-work, the activity-rest cycle adopts a changing phase relation with the light–dark cycle. The SCN might remain synchronized by the light–dark cycle; however, exposure to light at night and feedback from downstream outputs might alter circadian timing. On the other hand, feeding behavior sets time cues for peripheral organs that are in conflict with the SCN circadian control, leading to desynchronization within peripheral clocks, and between these and the SCN. This alterations might lead to metabolic, cardiovascular, endocrine, and gastrointestinal malfunctions
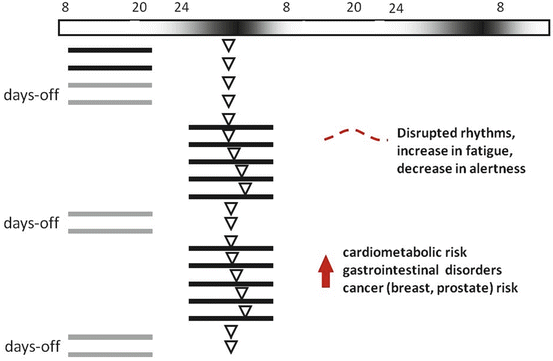
Fig. 15.4
Schematic representation of a typical shift work situation, with nocturnal working hours, and its expected disruptions on circadian rhythms and health. The horizontal top bar represents the relative light intensity perceived by the subjects, exposed to artificial light during the night. Black lines represents activity (grey on “days-off”), while white triangle represents a circadian marker (e.g., melatonin or core body temperature). Acute exposure to nightwork, with subsequent sleep deprivation, incoherent feeding/fasting timing, nocturnal light exposure, and increased sleep drive during the day, will lead to disrupted circadian rhythms in melatonin, cortisol, temperature, activity/rest, sleep/wake, and metabolism. Chronic exposure to abnormal entraining conditions might increase the risk of several health disorders
15.3.1 Metabolic Alterations
Although it has been known for several decades that many metabolic processes, such as glucose and cholesterol metabolism, are regulated by the circadian clock, it is only in the past few years that the severe metabolic consequences of circadian disruption have emerged. The SCN exerts a hierarchical daily control on metabolic rhythms by neurohumoral efferents to other brain regions (mainly several hypothalamic nuclei) and to autonomic centers, which drive hormones (e.g., glucocorticoids) and behavior (e.g., feeding) producing a set of physiological signals that synchronize peripheral tissues (reviewed in Delezie and Challet 2011; Huang et al. 2011). In addition, food-entrainable oscillators exert a secondary daily control on peripheral clocks (reviewed in Delezie and Challet 2011) and fine-tune the balance between energy intake and expenditure. Pancreatic (Marcheva et al. 2010) and thyroid hormones regulating carbohydrate and lipid metabolism (and thus basal metabolic rate) are under circadian control, as well as rate-limiting enzymes of metabolic pathways (Panda et al. 2002). Several molecular clock components are found to be regulators of cell metabolism through the so-called nuclear receptor transcription factors (as Retinoic acid-related orphan receptor α, RORα, reverse viral erythroblastis oncogene products, REV-ERBα, and peroxisome proliferator-activated receptors, PPAR) which sense metabolites such as fat-soluble hormones, vitamins, lipids, oxysterols, bile acids, and xenobiotics. For instance, CLOCK and BMAL1 act as transcription factors of PPAR (α, γ, δ) and estrogen-related receptor (ERR) family members (α, β, γ). Conversely, REV-ERBα and PPARα directly regulate BMAL1 expression, and relevant cellular (nutrient-redox) metabolic sensors are able to interact with clock genes (Sahar and Sassone-Corsi 2012). For instance NADH/NAD + balance regulates CLOCK/BMAL1 and NPAS2/BMAL1 heterodimer activity (Rutter et al. 2001) and, in addition, the NAD + -deacetylase dependent SIRT1 induced by food restriction can directly bind to CLOCK/BMAL1 (Nemoto et al. 2004). This physiological and molecular cross-talk of both clockwork and metabolic networks provides a mechanistic framework to understand how systematic circadian disruption will lead to metabolic alterations (Eckel-Mahan and Sassone-Corsi 2009). For example, circadian Clock mutant mice develop obesity (Turek et al. 2005), and diabetes was found in Clock-Bmal1 double-mutants due to impaired insulin secretion (Marcheva et al. 2010) and gluconeogenesis suppression (Rudic et al. 2004). Moreover, liver-specific deletion of Bmal1 in mice confirmed its strong involvement in hepatic glucose metabolism (Lamia et al. 2008).
Impaired entrainment in the laboratory also generates metabolic alterations, as when exposing mice to T cycles of 20 h, leading to larger body mass gain and increased insulin/glucose ratio (Karatsoreos et al. 2011). In human subjects exposed to controlled 28 h sleep–wake cycles under dim light, misalignment between the free-running central circadian pacemaker and the behavioral sleep–wake and fasting/feeding rhythms, resulted in increased plasma glucose and insulin concentrations, increased blood pressure, and decreased plasma leptin concentrations (Scheer et al. 2009). In addition, constant exposure of mice to bright or dim light led to increased body mass and reduced glucose tolerance as compared to mice housed under a normal LD cycle, despite equivalent levels of caloric intake and total daily activity output (Fonken et al. 2010). There is a well-documented increased risk for metabolic syndrome in humans subjected to shift work (reviewed in Zimberg et al. 2012) perhaps as the result of pathological adaptation to chronically sleeping and eating at abnormal circadian times. Disrupted sleep must be taken as an important factor in glucose metabolism alterations increasing the risk for obesity (Gangwisch 2009; Nedeltcheva et al. 2009; Spiegel et al. 2009) as show in a recent study with humans provided with 5 days with insufficient sleep, showing weight gain due to increased food intake exceeding energy needs (Markwald et al. 2013). On the other hand, a “night-eating” syndrome was detected in women, with a delayed circadian pattern of melatonin and food intake, presenting evening hyperphagia and frequent awakenings accompanied by food intake, while retaining a normal sleep–wake cycle. This alteration in feeding behavior is accompanied by delayed and low-amplitude rhythms in cortisol and several nutrients intake, and altered rhythms of metabolic hormones as insulin, ghrelin, and leptin (Goel et al. 2009). In addition, a failure to manage cholesterol in shift workers could lead to cardiovascular alterations (Ha and Park 2005) such as the ones discussed in the following section.
15.3.2 Cardiovascular Alterations
Autonomic nuclei that regulate the cardiovascular system are directly controlled by neural efferents from SCN and/or by circadian hypothalamic–pituitary factors acting on corticoid stress hormones, as well as by neurohumoral effectors on vascular tone. Cardiovascular risk markers as cortisol, nonlinear dynamic heart rate control, and hemostatic factors are higher during the morning, when adverse cardiovascular events are most likely to occur (reviewed in Morris et al. 2012b; Ruger and Scheer 2009). These circadian acute cardiovascular events are also suggested to be dependent on specific phase-relationship between peripheral oscillators at cardiovascular tissues (Davidson et al. 2005). Epidemiological studies show a consistent association for shift work as a factor for cardiovascular disease (reviewed in Knutsson and Boggild 2000), such as coronary failure (Nakamura et al. 1997; Tenkanen et al. 1997) or increased blood pressure in night workers (Lo et al. 2010; Su et al. 2008). A meta-analysis study of almost 2,000,000 shift workers detected higher risk for coronary events (especially myocardial infarct) in the nocturnal shift (Vyas et al. 2012). It is suggested that cardiovascular risk markers might respond unfavorably to exogenous stressors such as increased nocturnal activity (Morris et al. 2012b), promoting alterations in shift workers with misalignment between the circadian and the activity–rest cycles.
Disruption of circadian regulation in the laboratory increases several risk factors of cardiovascular disease. Chronic LD-shifts increased plasmatic plasminogen activator inhibitor-1 (PAI-1) levels (Oishi and Ohkura 2013), which promotes hypofibrinolysis. The aforementioned study of Scheer et al. (2009) in humans forced to follow a 28 h sleep–wake cycle indicated increased blood pressure during wakefulness. Altered cardiovascular functions are found in the 22 h short-period hamster τ, with a point mutation in Ck1ε circadian regulatory gene, which exhibits abnormal 24 h LD behavioral entrainment and disrupted rhythms. These animals had cardiomyopathy, extensive fibrosis, and severely impaired contractility with shortened life-expectancy (Martino et al. 2008), while under T22 cycles (i.e., their normal endogenous period) behavioral rhythms and cardiac phenotype are normalized. Several vascular dysfunctions were found in clock gene-deficient mice. In Bmal1 knock-out mice, abnormal thrombosis and vascular remodeling responses were found, while Clock mutants showed impeded responses to vascular injury; moreover, in Bmal1, Clock, and Per2 mutant mice, vascular endothelial dysfunction was found (Anea et al. 2009; Viswambharan et al. 2007). Also, blood pressure was altered in Cry 1–2 null mice, showing hypertension due to deregulation of salt homeostasis caused by abnormal high synthesis of adrenal mineralocorticoid aldosterone (Doi et al. 2010).
15.3.3 Gastrointestinal Alterations
The gastrointestinal tract (GT) displays circadian rhythms in several functions and variables, such as motility, maintenance and replacement of the protective barrier, absorption rates, and enzyme secretion rhythms (reviewed in Konturek et al. 2011). Furthermore, rhythmic clock gene expression has been found in peripheral oscillators throughout the GT, which can be shifted, or entrained, by timed meals (Hoogerwerf et al. 2007; Mazzoccoli et al. 2012; Polidarova et al. 2011). The correct phase-relationship between feeding time and the activity–rest cycle led to normal entrainment of the GT, by a myriad of neural and humoral signals related with the interplay between SCN activity, food-entrainable oscillators, and metabolism (Stenvers et al. 2012). There are evidences showing that circadian disruption leads to alterations in gastrointestinal physiology. Increased general symptoms for gastrointestinal disorders were more prevalent among shift workers as compared with day workers (Koller 1983), and aircrew members, especially those on long-distance flights, had more upper-GT symptoms than ground staff (Enck et al. 1995). The consequences on gastrointestinal functions of shifting working schedules might be related to an increase in food consumption during the rest cycle, since time of feed has been shown to be the strongest Zeitgeber in gastrointestinal oscillators (Malloy et al. 2012; Polidarova et al. 2011), resulting in a misalignment between circadian clocks among the body. There is a higher prevalence on peptic ulcer among shift workers (Higashi et al. 1988; Segawa et al. 1987), which might be related to higher incidences in Helicobacter pylori infections in shift workers (Pietroiusti et al. 2006; Zober et al. 1998). Irritable bowel disease prevalence was also higher in nurses working rotating shifts (Nojkov et al. 2010).
Circadian disruption by chronic lighting in rats fed ad libitum abolished rhythmic clock gene expression in the liver and colon, while a restricted feeding schedule restored these rhythms (Polidarova et al. 2011). Similar results were found in SCN-ablated mice, where restricted feeding re-established locomotor, feeding, and stool output rhythms (Malloy et al. 2012). Per1–2 double knockout mice exhibited abolished rhythms in colonic functions, such as motility, intracolonic pressure changes, stool output, and circular muscle contraction (Hoogerwerf 2010).
15.3.4 Immune Alterations
The circadian system exerts a tight control over the immune system and its function, which in turn feedbacks over the circadian clock (reviewed in Cermakian et al. 2013; Scheiermann et al. 2013). Robust rhythms in the number of immune cells, plasmatic levels of proinflammatory cytokines, and immune cell-produced peptide hormones, as well as in major humoral responses, have been described vastly, in humans and animal models (Fortier et al. 2011; Haus and Smolensky 1999; Silver et al. 2012). In addition, circadian disruption has profound effects on the immune system, which has been evidenced both in animal models and in human studies. Female nurses working in a three-shift rotating system showed a progressive decrease in natural killer (NK) cell activity and CD16+ CD56+ cells number (Nagai et al. 2011). Shift working has also been linked to a higher risk for common infections, with the highest risk in workers going through three different shifts (Mohren et al. 2002). Impeding normal LD-entrainment by chronic jet lag under weekly 6 h phase-advances altered the inflammatory response of mice to an experimental challenge with lipopolysaccharide (LPS). After four consecutive shifts, jet lagged mice displayed a fivefold increase in mortality and severe hypothermia due to LPS, as compared to LD-entrained controls. In this chronic jet lag the expression of the cytokines IL-1β, IL-10, IL-12, IL-13, GM-CSF, and TNF-α was significantly increased 24 h after LPS injection, and in vitro macrophage stimulation with LPS produced higher levels of IL-6 (Castañon-Cervantes et al. 2010). Chronic jet lag also impaired NK cells cytolytic activity, as well as altered rhythms in clock gene and cytokine expression (Logan et al. 2012b). Deleterious effects of abnormal entrainment were also reported in a mice model of experimental colitis, where chronic inversions of the LD cycle every 5 days led to increased symptoms as reduced body mass, abnormal intestinal histopathology, and potentiated inflammatory response (Preuss et al. 2008).
Changes in the sleep–wake rhythm, which are frequently concomitant with circadian disruption also have profound effects on the immune system. An altered sleep–wake pattern changed the HPA axis activity increasing cortisol, and consequently affecting the expression of proinflammatory cytokines, such as IL-6 or TNF-α (Vgontzas and Chrousos 2002). Additionally, NK cell activity, antigen uptake, circulating immune complexes, and secondary antibody responses were altered by sleep loss (Krueger et al. 2003), while IL-1β and TNF-α levels increased during sleep deprivation (Majde and Krueger 2005). In addition, sleep deprivation leads to the suppression of immune surveillance, which may permit the establishment and/or growth of malignant clones.
Alterations of clock genes, especially those of the period family, disrupt immune functions. Per3 clock gene polymorphisms have also been associated with circadian disruption and with increased cancer risk together with elevated IL-6 concentrations (Guess et al. 2009). Homozygous Per1 mutant mice exhibited altered cytokine rhythms (e.g., interferon-γ) and cytolytic factors (e.g., perforin and granzyme B) in splenic NK cells (Logan et al. 2013). On the other hand, Per2-deficient mice were more resistant to LPS-induced endotoxic shock than control wild-type mice. Moreover, serum levels of IFN-α and IL-1β were dramatically decreased in Per2−/− mice following an LPS challenge, attributable to defective NK and NKT cell function (Liu et al. 2006). In addition mice carrying hematopoietic Cry1−/− Cry2−/− mutant cells presented a potentiated immune response to LPS administration, with higher levels of circulating proinflammatory cytokines (Narasimamurthy et al. 2012).
Chronodisruption may result in altered immune responses, such as aberrant immune cell trafficking and abnormal cell proliferation cycles, which can lead to cancer (Mormont and Levi 1997). Per2 mutant mice have been shown to present enhanced cancer susceptibility (Fu et al. 2002; Wood et al. 2008), along with disrupted circadian rhythms of NK cell gene expression and function.
15.3.5 Endocrine Alterations
There is an intimate connection between the circadian clock and multiple endocrine axes, which involve several aspects of the daily regulation of hormonal release. A highly specialized organization configures the SCN neural output to both hypothalamic neuroendocrine neurons (i.e., cells containing or releasing corticotrophin-releasing hormone, CRH, tyrotrophin-releasing hormone, TRH, or gonadotrophin-releasing hormone GnRH) and pre-autonomic neurons, as well as to other brain structures (Kalsbeek and Fliers 2013). Such output ensures the coordination between behavioral, endocrine, and metabolic rhythms needed for the circadian adjustment of the organism. For instance, glucose homeostasis is tightly regulated in order to predict the energy needs for the circadian onset of behavioral activity, by increasing hypothalamic CRH and adrenal cortisol release, and by activating hypothalamic orexins at the right time of day (Kalsbeek et al. 2011).
One of the best known examples of endocrine circadian regulation is represented by the synthesis and release of pineal melatonin, which is under the control of sympathetic autonomic efferents commanded by the SCN (Moore 1996). Blood melatonin increases during the night, it is suppressed by light (Zawilska et al. 2009), and its amplitude and duration are proportional to photoperiod duration (Hazlerigg 2012). Indeed, melatonin can be considered a daily and calendar signal which communicates circadian and circannual cues to the body. In recent years, additional evidence suggested an antioxidant role for this hormone, which falls outside the scope of this chapter (Reiter et al. 2010).
It is well known that sleep disruption affects autonomic and endocrine responses. A representative example is the fact that cortisol rhythms are altered in night-working emergency physicians (Machi et al. 2012), shift-working nurses (Korompeli et al. 2009) and in police officers working night or afternoon shifts than day shifts, with maximal differences occurring after 5 days of shift work (Wirth et al. 2011). Moreover, flights involving 7 h of jet lag produced a decrease in cortisol levels that lasted for 9 days after the flight (Pierard et al. 2001). Furthermore, in laboratory conditions, in which subjects were kept in a 28 h forced desynchronization protocol, cortisol was more strongly coupled to the circadian endogenous component than to the environmental “day.” Indeed, an inverted pattern of cortisol secretion was observed when sleep–wake and circadian cycles were misaligned. On the other hand, leptin rhythm followed only the sleep–wake cycle component, with conflict phases of misalignment between the circadian and behavioral component resulting in suppression of circulating leptin levels (Scheer et al. 2009). More information is needed (and on the way) regarding the role of disturbed hormonal cycles on circadian synchronization, both at central and peripheral level.
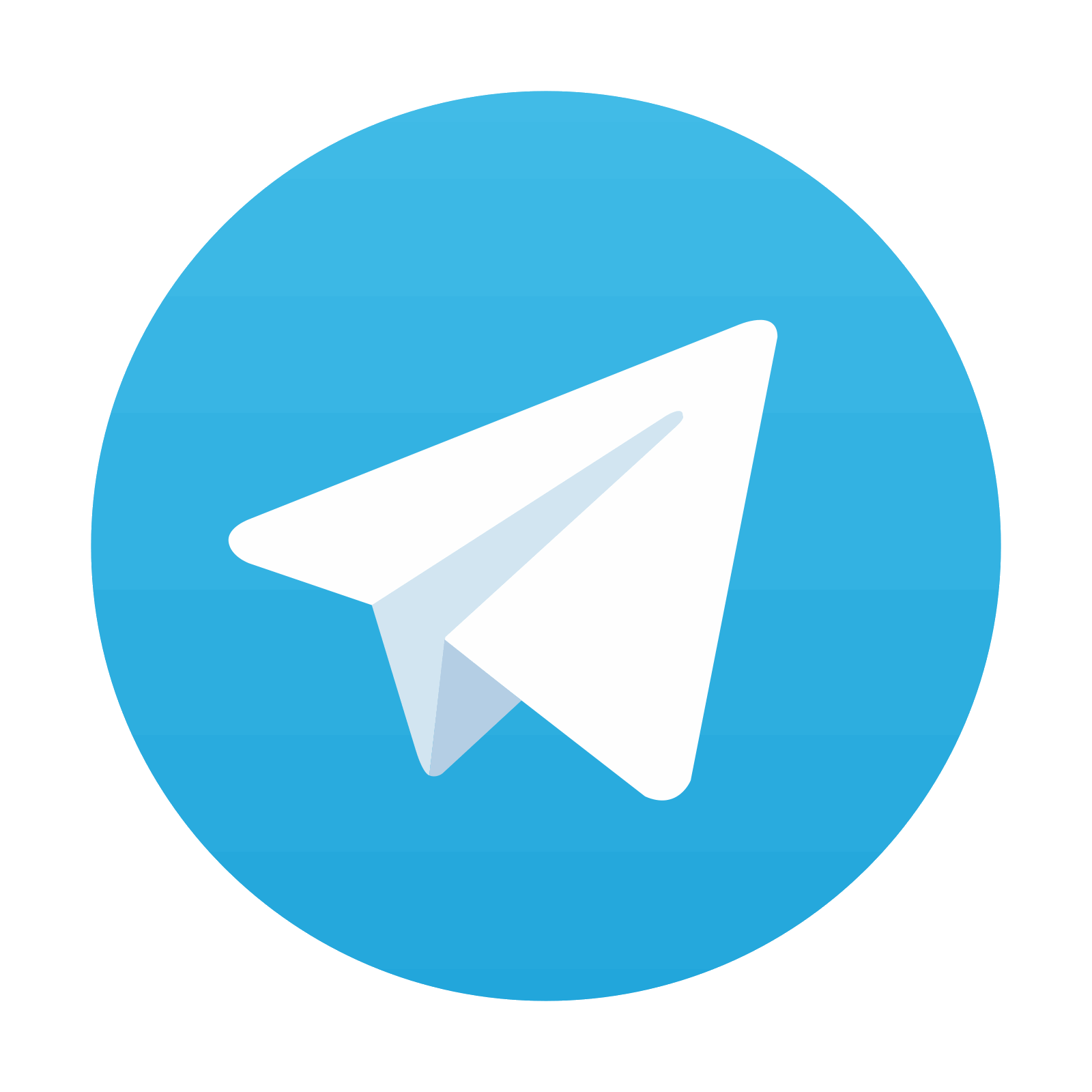
Stay updated, free articles. Join our Telegram channel
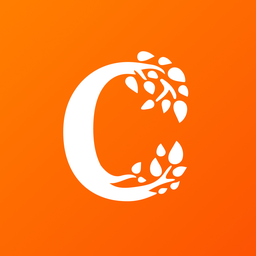
Full access? Get Clinical Tree
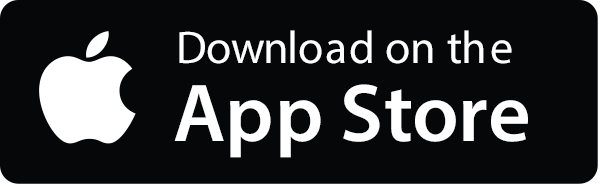
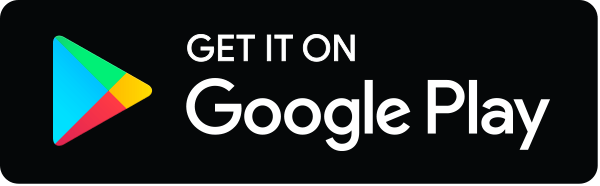