Chapter 15 Drugs Acting on Blood or Blood-Forming Organs
Drugs Stimulating Medullary Poeisis
Bone Marrow Regulation
The bone marrow contains nonhematopoietic (osteoblasts, of pluripotential stromal origin) and hematopoietic (osteoclasts, of macrophage/monocyte origin) stem cells as well as nonosteogenic cells such as platelets and lymphocytes. Interaction is critical to hematopoiesis, with both nonhematopoietic and nonosteogenic cells contributing to regulation of hematopoietic cells and medullary poiesis. Multiple factors control the commitment, proliferation, and differentiation of bone cell precursors. These include but are not limited to cytokines, growth factors, systemic hormones, and transcriptional regulators. Cell–cell and cell–matrix interactions maintain contact between osteoblastic cells and osteoclast marrow precursors and between the stroma and hematopoietic cells. Among the interacting signals are adhesion molecules, including but not limited to integrins, selectins, and cadherins. Osteoclastogenesis is stimulated by interleukin (IL)-1 and tumor necrosis factor (TNF). The latter is released from marrow mononuclear cell lines in response to NFκB ligand (RANKL), IL-6, IL-11, macrophage colony-stimulating factor (M-CSF) and granulocyte-macrophage colony-stimulating factor (GM-CSF) released from the stroma. Megakaryocytes appear to play a major role in the regulation of hematopoiesis through expression of a number of mediators such as RANKL, n-methyl d aspartate-type glutamate receptors, calcium-sensing receptors, osteonectin, and osteocalcin. Another example is thrombopoietin, which simultaneously regulates megakaryopoeisis and inhibits osteoclastogenesis.1
Red Blood Cell Formation
Hematopoiesis
Erythropoietin (EPO) is the most important regulator of the proliferation of committed erythroid cells (see the discussion of erythropoiesis-stimulating agents [ESAs]) (Figure 15-1).2 The kidney is the major site of EPO production, where it is released in response to anemia or hypoxia. Among the more important regulators of the myeloid series are granulocyte colony-stimulating factor (G-CSF) and granulocyte/macrophage colony-stimulating factor (GM-CSF). Several vitamins are needed for red and white blood cell formation (hematopoiesis and granulopoiesis, respectively).2,3
Drugs Affecting Red Blood Cell Formation
Pharmacologic therapy (Table 15-1) for anemia is oriented toward (1) providing components needed for red blood cell (RBC) production (e.g., proteins, vitamin B12, and folic acid), including hemoglobin synthesis (iron and other minerals); and (2) stimulating bone marrow formation of RBCs.
Components Needed for Red Blood Cell Production
Vitamin B12
Vitamin B12 (cyanocobalamin), the “maturation factor,” is essential for DNA synthesis, and its deficiency inhibits nuclear maturation and division. Because cells that rapidly multiply are affected first, reduced RBC proliferation and a “maturation arrest” in the bone marrow are among the first indications of a vitamin B12 deficiency. The erythroblast cannot continue to divide, becomes very large, and is referred to as a megaloblast (megaloblastic anemia). The mature erythrocyte is also large and is referred to as a macrocyte. Although its oxygen-carrying capacity is adequate, the enlarged cell is very fragile because of its large size, and it has a reduced life span.2,3
Vitamin B12 is a porphyrin-like compound with a ring containing a centrally located cobalt.2 It can be acquired from both the diet and microbes in the gastrointestinal tract. Most microbial production is in the large intestine, however, where B12 is not readily absorbed because it is dependent upon receptor mediated uptake. Dietary deficiency of B12 is unlikely and usually results from poor absorption (including pancreatic enzyme deficiency) from the gastrointestinal tract. Absorption of B12 is complicated (Figure 15-2) and depends on several factors.2 Gastric acid and pancreatic enzymes are needed to release B12 from dietary and salivary binding proteins.2 To avoid being digested, B12 is protected by binding to intrinsic factor (excreted by the exocrine pancreas in cats4) and R protein, which are secreted by the parietal cells. The bound B12 complex is carried to the ileum, where B12 is adsorbed to highly specific receptor sites on the brush border. Vitamin B12 enters the cell by pinocytosis and then enters the blood, where it is bound with transcobalamin, the plasma carrier. Excessive vitamin B12 is stored in large quantities in the liver and is slowly released as needed. Vitamin B12 is excreted into the bile but undergoes enterohepatic cycling. Interference with absorption by the ileum will result in continuous depletion of B12. Many months of defective vitamin B12 absorption are necessary, however, before vitamin B12 deficiency occurs. The anemia resulting from B12 deficiency is also referred to as pernicious anemia. Exclusive uptake of cobalamine in the ileum as led to its detection in serum as a tool for diagnosing disease of the ileum.4 Deficiency has been described (in cats) by increased serum methylmalonic acid (MMA) coupled with serum cobalamine at or below 100 ng/mL (the lowest detectable concentration). However, the description of clinical and biochemical signs associated with deficiency focused on the gastrointestinal tract and did not address tests indicative of anemia.
Vitamin B12 is available as a parenteral preparation in the pure form of cyanocobalamin or the more highly protein bound hydroxocobalamin. Hydroxocobalamin may provide more sustained effects than cyanocobalamin when given by injection.2 Vitamin B12 is also available for oral administration in the pure form or in combination with other vitamins and minerals. Methylcobalamin is another congener of vitamin B12 and represents one of the two intracellular active forms of the vitamin (the other being deoxyadenosylcobalamin).2 Foods high in vitamin B12 include selected microbial sources and animal (meat) products. There are no significant toxicities associated with therapy. Indications for B12 therapy are limited to situations of B12 malabsorption such as ileectomy, gastrectomy, malabsorption syndromes, or chronic administration of cimetidine or other antisecretory drugs because an acid environment is necessary for release of B12 from the diet and for intrinsic factor activity. In one study4 response to cobalamin treatment (250 μg subcutaneously once weekly in cats (n = 19) with gastrointestinal disease was prospectively studied, with a focus on indicators of gastrointestinal health. Indicators of cobalamin deficiency (serum MMA, cysteine) improved and were correlated with weight gain and folic acid use, however, improvements in indices of red blood cell health were not addressed.
Folic Acid
Folic acid (pteroylglutamic acid) is a cofactor needed for DNA synthesis because it promotes the formation of a nucleotide necessary for DNA formation. Folic acid is also necessary for RNA synthesis, and it serves as a methyl donor for the formation of vitamin B12.2 Folic acid is acquired from the diet, although it can also be formed by microbes. Dietary sources include yeast, liver, kidney, and green vegetables. Folic acid is also stored in the liver but not as avidly as is vitamin B12. It undergoes enterohepatic circulation but is destroyed daily by catabolic processes. Daily requirements are high, and serum levels will fall rapidly several days after dietary deficiency. Gastrointestinal absorption of folic acid is not as complex as that of vitamin B12, although it requires protein digestion and the presence of dihydrofolate reductase in the small intestine. Jejunal pathology can result in folate deficiency. The degree of folic acid binding to plasma proteins is not well understood.
Folic acid is available as both a parenteral and an oral (pure or combined product) form. The minimum daily requirement in humans is 50 μg/day, but this can increase to 100 to 200 or more in patients with high cell turnover rates (e.g., hemolytic anemia).2 In humans the most popular form of folic acid supplementation is as part of a multivitamin preparation containing 400 to 500 μg of pteroylglutamic acid. In high disease states, 1 to 2 1-mg tablets are consumed. This dose may be the source of the recommended dose for cats and dogs. Compared with commercially available products, the dose recommended for small animals seems excessive, and it is not clear that such a high dose is necessary. There are, however, no apparent significant toxicities associated with therapy.
Indications for therapy are inadequate intake as a result of the administration of several drugs (methotrexate, potentiated sulfonamide antibiotics, some anticonvulsants such as phenytoin), liver diseases, malabsorption, or other chronic debilitating diseases. Folinic acid (leucovorin) is a formyl derivative of tetrahydrofolic acid and as such does not require the action of dihydrofolate reductase in order to act as a folate by contributing a carbon moiety. In humans, administration of folinic acid increases serum folate activity owing to 5-methyltetrahydrofolate. Folinic acid apparently serves as a substrate for inhibitors of dihydrofolate reductase such as methotrexate, or diaminopyrimidines such as trimethoprim or ormetoprim, and as such, can resolve some deficiencies associated with folic acid deficiency. However, it will not replace deficient folic acid or any of its derivatives prior to the tetrahydrofolate step in folic acid use.2 As such, leucovorin can be used clinically to circumvent the actions of dihydrofolate reductase (e.g., methotrexate) and replace materials in the folic acid pathway beyond tetrahydrofolate, but can not prevent folic acid deficiency. Leucovoran also inhibits thymidylate synthtase and as such, facilitates anticancer efficacy of 5-fluorouracil.
Hemoglobin Synthesis: Iron
Iron is a component of hemoglobin, myoglobin, and other substances, such as those found in cytochrome and electron transport systems.2,3 Approximately 65% of total body iron is present in hemoglobin, 4% as myoglobin, and 1% in cytochromes and electron transport systems. The remaining 15% to 30% is stored as either ferritin, the soluble form of iron stores, or hemosiderin, the insoluble stores. Oral absorption of iron is slow, complicated, and not well understood (Figure 15-3). It is available in the diet in either a heme form, which makes up a small percentage of the total but readily absorbed form, or in a nonheme (ferric oxide) form. The nonheme form represents the largest dietary fraction, but its absorption is profoundly affected by dietary factors. Nonheme iron must be converted to the ferrous form for absorption to occur; conversion depends on an acidic environment. Absorption of iron is increased by hydrochloric acid and decreased in situations that decrease acid production in the stomach (e.g., chronic use of antisecretory drugs).
Preparations
Iron is available in both oral and parenteral preparations. Oral preparations are prepared as ferrous (bivalent) or ferric (trivalent) salts. Ferrous salts tend to be the treatment of choice for oral supplementation and are dosed according to their iron content.2 Examples of bivalent ferrous salts include sulfate (20% iron), gluconate (12%), and fumarate (33%). The ferrous bivalent salts are more soluble in the gastric environment and are absorbed three times faster than the trivalent salts. The efficacy of polysaccharide oral iron products approximates that of ferrous products. Slow-release iron products have not been well studied. These products may be continued for several months; toxicities and side effects are dose related.
Parenteral preparations are indicated if oral preparations cannot be tolerated or are not feasible. Iron dextran administered by intramuscular injection generally is preferred. Parenteral administration results in a more rapid accumulation of iron stores, which may take months (in humans) with oral therapy. Other indications for parenteral therapy include diseases of the gastrointestinal tract that preclude iron absorption or that will exacerbate another disease (e.g., inflammatory bowel disease) or intolerance of oral supplementation.2 Much of the iron given intramuscularly remains at the site of injection for several months. The remaining iron enters the plasma but must first be phagocytized by reticuloendothelial cells for processing. This may take several months, and evaluation of total body iron may be difficult until all of the iron is processed.2
In human patients iron injection is preceded by a test dose (approximately 0.5 mL). It is given only in a large muscle mass and is associated with long-term discomfort, local skin discoloration, and a perceived risk of malignant change at the site of injection. Selected iron dextran preparations also can be administered intravenously. Dosing is based on a conversion of body weight (0.66 × weight in kilograms) and on the patient’s hemoglobin level compared with the desired hemoglobin level (14.8 g/dL).2 After an initial 0.5-mL test dose, the calculated dose is given in 2-mL increments each day until completed. Side effects associated with intravenous administration include malaise, fever, arthralgias, urticaria, and generalized lymphadenopathy.2
Drug interactions
Several drugs, including tetracyclines and antacids, and several foods bind to and precipitate iron when given orally. Absorption is enhanced in the presence of ascorbic acid because it reduces ferric iron to its ferrous state and prevents formation of insoluble and unabsorbable iron compounds.5
Clinical use
Indications for iron therapy are limited to treatment or prevention of iron deficiency such as occurs with blood loss or successful therapy with anabolic steroids or recombinant EPO in a patient suffering from chronic anemia. The efficacy of “shotgun” products is questionable. As with any hematinic preparation, provision of these compounds will be ineffective if the nutritional status of the animal is poor. Shotgun preparations are products that contain a combination of hematinic agents such as vitamin B12, folic acid, pyridoxine, riboflavin, nicotinic acid, pantothenic acid, thiamine, biotin, ascorbic acid, and vitamin E. The need for inclusion of all of these products is not clear. An exception might be made for ascorbic acid, which enhances oral absorption of iron, and pyridoxine, which is useful in human patients with selected anemias. One human patient with pure red cell aplasia has responded to riboflavin. Occasionally, these products might be considered dangerous because some additives may be sufficiently high to mask clinical signs of other nutrient deficiencies that ultimately may become life threatening.2
Bone Marrow Stimulation
Erythropoiesis-Stimulating Agents
EPO, an endogenous glycosylated protein hormone (MW 30,000 daltons), is the most important regulator of committed erythroid progenitor proliferation.2 Extensive glycosylation is responsible for the activity of the molecule.6 The kidney (peritubular cell) is the primary source of endogenous EPO, although the liver produces a small amount in some species. Insufficient oxygen delivery to tissues is the primary stimulus for promotion of the transcription and thus production and secretion of EPO2 (see Figure 15-1). Hypoxia or anemia will increase synthesis by a hundredfold or more. However, renal disease, damage to the bone marrow, or deficiencies of iron or other essential vitamins can impair synthesis. Inflammatory cytokines also impair secretion (as well as iron delivery). Prostaglandins appear to increase and nonsteroidal anti-inflammatory drugs appear to decrease EPO production.
Mechanism of Action
Once released from the renal cell, EPO travels to the bone marrow, where it binds to receptors on the surface of committed erythroid progenitors. The influence of EPO (including ESAs) on RBC production occurs at several steps. EPO binding initiates changes in intracellular phosphorylation.2 Additionally, EPO stimulates proliferation and differentiation of erythroid precursors, including BFU–erythroid CFUs: erythroid, erythroblasts, and reticulocytes.2 Recombinant human EPO also stimulates the release of reticulocytes from the bone marrow into the blood, where they subsequently mature.7–11
Preparations
Human EPO has been isolated and cloned and can be synthesized in large quantities with recombinant techniques using a mammalian (hamster cell line). ESAs include two recombinant EPO (rEPO) products. Epoetin is a recombinant human product (rhEPO), available in two products that differ in their glycosylated carbohydrate patterns: alpha (Epogen, Eprex) and beta (Procrit). A second ESA is darbepoetin, a recombinant hyperglycosylated analog of the human protein, often referred to as a second-generation EPO. Hyperglycosylation increases stability. Darbepoetin is dosed in μg/kg, whereas epoetin is dosed as units/kg for epoetin: a dose of 6.25 to 12.5 μg/kg of darbepoeitin is equivalent in humans to a dose of 2500 to 5000 units/kg of rhEPO (0.0025 μg darbepoetin to 1 unit epoetin).12 Albumin is included as a vehicle in both epoetin alpha and darbepoetin. However, because of the concern regarding potential transmission of disease carried by albumin, alternative vehicles such as polysorbate 80 have been used to formulate epoetin beta.13 Products are intended for intravenous or subcutaneous administration. Both canine (rcEPO)14 and feline (rfEPO)15 recombinant products have been developed but are not yet commercially available. Canine EPO is more closely homologous to feline EPO compared with rhEPO and will be preferred in cats should rcEPO approval precede the feline product.
Disposition
The disposition of ESAs has not been well characterized in either humans or animals. Glycosylation (addition of carbohydrates) prolongs elimination compared with the endogenous proteins. In humans the elimination half-life of rh-EPO is about 6 to 9 hours after intravenous administration, with a very small volume of distribution (0.055 L/kg). As with most endocrine products, plasma half-life may not necessarily reflect biological half-life.9,11 Glycosylation of darbepoetin is greater than that of epoetin, leading to an accordingly longer (threefold) biological half-life in humans. Dosing subsequently can be reduced to every other day (3 times weekly).2
Adverse Effects
Antibody formation
Use of ESAs is associated with immunogenicity, and relevant side effects should be anticipated when administering foreign protein products intended to mimic endogenous protein products.16 Pure red cell aplasia (PRCA) is a recognized, albeit rare, sequela of rEPO in humans.17 In 1998 a formulation was approved internationally that varied from the U.S. product by vehicle, storage, and handling. The use of the product was associated with an increased incidence of PRCA, leading to the assumption that the differences increased the immunogenicity of the product.18 The increased frequency of disease facilitated its characterization.Clinically manifested as a rapid onset of severe anemia, refractoriness to rhEPO therapy, and a low reticulocyte count, PRCA is associated with high concentration of EPO-neutralizing antibodies, probably of IgG origin.6,19 Diagnosis is confirmed by the presences of EPO neutralizing antibodies in circulation, absence of bone marrow red cells and normal to elevated transferrin saturation. Testing no longer appears to be available for either eryropoietin or antibodies for dogs or cats. In one study, greater than 95% of human cases afflicted with chronic renal disease received the drug subcutaneously for a mean of nine months before the onset of PRCA. Antibodies resolved in 80% of patients when the drug was discontinued but only in the presence of immunosuppressive therapy.13,17,19 A lower incidence of PRCA was found in patients with cancer chemotherapy–related anemia, probably because anticancer therapy led to immunosuppression.13 The incidence of PRCA has been reduced by methods intended to reduce immunogenicity. These included changes in processing that contributed to immunogenicity (such as freeze drying), changes in packaging (replacement of rubber stoppers with Teflon, removal of silicone lubricant in prefilled syringes), and a shift from subcutaneous to intravenous administration.13 Darbepoetin may be associated with less immunogenicity compared with rhEPO, as is shown by response in one human patient to darbepoetin despite development of rhEPO-induced PRCA.20
Because of the foreign nature of EPO, adverse effects reported in humans also should occur with rhEPO administration in animals. Local and systemic allergic reactions include cellulitis, fever, arthralgia, and mucocutaneous ulcerations, which occurred in 12% of animals treated in a pilot study.21–23 Signs resolve with drug withdrawal but may reappear when treatment is resumed. Because antibodies directed toward the foreign rhEPO may stimulate production of antibodies targeted toward endogenous EPO, RBCs, hematocrit, and hemoglobin may progressively decrease starting as early as 2 weeks of therapy.21–23 Antibodies are indicated by a bone marrow myeloid-to-erythroid cell ratio of less than 8. Discontinuation of rhEPO will result in resolution of antibody formation and, to some degree, the anemia (i.e., that caused by antibodies), but drug therapy cannot be reinstituted.22 The incidence of antibody formation is high, developing in 63% to 100% of healthy dogs receiving EPO.24 Red cell aplasia was associated with anti-Epogen antibodies in two out of three dogs treated longer than 90 days and seven out of eight cats treated for 180 days or more. For the cats, 70% were refractory to further EPO therapy, requiring transfusion therapy until anti-epoetin antibody concentrations decreased (2 to 4 months after rhEPO was discontinued. Because both exogenous and native EPO may be impaired, aplastic anemia resulting from bone marrow failure may ultimately occur. Immunosuppressive therapy directed toward antibody formation, although apparently successful in humans, has not been addressed in veterinary medicine. Antibodies generally require 2 to 12 months after therapy is discontinued for eradication. Species specificity of rEPO should minimize the advent of anti-EPO antibodies as has been demonstrated for both the dog24,25 and cat.15 Randolph and coworkers15 have demonstrated that the feline recombinant product not only does not induce antibodies but also will re-establish erythropoiesis in most cases of rhEPO-induced red cell aplasia. Unfortunately, the same does not appear to be true for the canine recombinant preparation25 in dogs, suggesting that even species-specific origin of rEPO may be associated with antibodies.
Miscellaneous adverse effects
Other adverse effects that may require monitoring in patients receiving EPO include systemic hypertension, iron deficiency, hyperkalemia, and polycythemia.8 Flulike symptoms occur in some human patients receiving the drug intravenously. Hypertension occurs in many human patients with renal disease as the hematocrit normalizes. Patients who begin the use of rhEPO when in a state of hypertension are likely to experience a further increase in blood pressure. The mechanism of hypertension is not known but may include increased blood viscosity or peripheral vasoconstriction. Blood pressure increases within as little as 2 weeks of therapy and tends to stabilize by month 4. Increased blood pressure has been reported in 40% to 50% of dogs or cats treated with ESAs.26 It may be necesary to adjust drug therapy for hypertension (e.g., increase amlodipine dose).27 Polycythemia is another likely sequela of ESAs if response to therapy is not monitored on the basis of packed cell volume (PCV).27 Other miscellaneous adverse effects of rhEPO in dogs and cats include seizures (one in six dogs, two of eleven cats26), particularly in animals with moderate to severe azotemia and as a terminal clinical sign.27 Depleted iron stores should be anticipated in patients with iron deficiency (discussed later).22 Pain on subcutaneous injection (a route being used less frequently in humans in order to minimize PRCA) varies with products, with the alpha (e.g., Eprex) being more painful in humans than the beta (Procrit). In an animal model involving renal ablation, rhEPO actually hastened the progression of chronic renal disease. However, the clinical relevance of this finding has not been documented.
Indications and Clinical Use
The primary indication for ESAs in humans is anemia of chronic renal disease (CRD).7,8,21,23,28 Most human patients receiving renal dialysis also receive ESAs. Indications in animals are likely to be similar to those in humans, particularly for renal disease, because clinical signs of weakness, somnolence, depression, and poor appetite associated with CRD in cats (and presumably dogs) is due to anemia. Decisions must be made regarding the product (epoetin, alpha or beta, versus darbepoetin), route (intravenous versus subcutaneous), and dosing regimen (dose and interval). Subcutaneous administration of epoetin—which can cause pain on administration—has been associated with better response in humans, although it appears to be more immunogenic. In contrast, darbepoetin appears to be equally efficacious regardless of the route.29 Preference among the ESA products is not yet clear; evaluation of darbopoetin currently is ongoing.30 Advantages of darbopoetin may include faster response time, particularly with front end loading, decreased dosing intervals, and decreased risk of immunogenicity. The average dose of darbepoetin used in human patients with CRD or cancer ranged from 3.5 to 5 μg/kg 1 to 3 times per week; preliminary studies indicate that administration as infrequent as once every 3 weeks may be sufficient.
Because it has been available longer, more information is available regarding rhEPO use in both humans and animals. In humans with CRD, rhEPO normalizes the hematocrit, hemoglobin concentration, and RBCs after administration of 15 to 300 U/kg rhEPO 3 times weekly. Some human patients have required 500 U/kg. A stair-step approach has been used for nonresponding CRD in human patients in that the dosage continues to be increased until the desired response is achieved.8 When the desired response is achieved (a hematocrit of 30% to 40%), a smaller maintenance dose (25 to 100 U/kg three times a week) is given to maintain the hematocrit above 30%.8 Normalizing the PCV in patients with underlying renal disease may be associated with a greater risk of cardiovascular side effects.31 Because of convenience and cost, in humans the maintenance dose generally has been given subcutaneously, whereas the induction dose is often given intravenously. However, intravenous administration increasingly is being used for maintenance dosing to minimize the risk of immunogenicity (in human patients).18 The intravenous route in humans has the added advantage of convenience in those individuals with chronic access ports (i.e., human patients undergoing dialysis), a situation that is less likely in animals.
Erythropoetin therapy is indicated in dogs or cats with a PCV of 20% to 25% or less owing to renal disease (see Table 15-1). To date, of the commercially available products, reports (whether scientific or anecdotal) of use in animals are limited to alpha EPO. Recombinant human EPO has been used in small animals with chronic anemia.21,22,23 When administered in uremic animals with CRD, the hematocrit of most patients normalizes within 3 to 4 weeks of therapy, and the clinical well-being of patients improves. Response to therapy is indicated by reticulocytosis, and an increase in hematocrit of 0.5% to 1% each day. Hypokalemia associated with uremia in cats should also resolve, in part because of improved appetite.22 White blood cells and platelets do not seem to be affected. Caution is indicated for patients that are hypertensive before rhEPO therapy. Informed consent should be obtained before use because of the high incidence of antibody formation. Initial treatment should begin at 100 U/kg (or, if a slower increase is acceptable, 50 to 75 U/kg) subcutaneously 3 times a week until the target hematocrit (originally 37% to 45% in dogs or 30% to 40% in cats; more recently 30% to 35% for dogs and 25% to 30% in cats) has been reached.27 Generally, response requires approximately 4 weeks. The PCV should be measured twice weekly for the first several months of therapy. The dose is decreased to a maintenance dose of 50 to 100 U/kg and the frequency of administration to twice weekly once the target PCV has been achieved. The dose should be titrated to maintain the PCV in the mid-target range. If the PCV does not increase sufficiently, the dose of rhEPO can be increased in 25- to 50-U/kg increments. The maintenance dose will vary for each patient. A maximum dose has not been established in animals, although weekly doses of 300 to 1050 U/kg have been reported.22
Among the more common causes of ESA therapeutic failure in humans is iron deficiency. Iron therapy generally is begun along with rhEPO. Iron therapy is not easily monitored. In humans serum ferritin and transferrin iron saturation are the most common tests used.32 An increasing percentage of hypochromic red blood cells and decreasing content of hemoglobin in reticulocytes may also reveal iron deficiency but will not reveal iron overload. Iron gluconate and iron sucrose are considered the safest of the intravenous iron medications.32 However, because intravenous iron dextran therapy is more expensive and is associated with anaphylactic therapy, oral iron therapy is preferred.2,8 Further, the body can control iron content with oral but not intravenous administration. Differences in the gastrointestinal absorption of iron may alter response to rhEPO therapy. Addition of 200 mg of oral iron, however, appears to successfully maintain adequate iron stores in human patients with CRD receiving rhEPO. Oral iron supplementation in dogs is recommended at 100 to 300 mg of ferrous sulfate daily (20- to 60-mg elemental iron) and for cats 50 to 100 mg (10 to 20 elemental).27 Iron dextran (10 to 20 mg/kg for dogs and 50 mg total dose for cats) is recommended by intramuscular administration (deep) for animals that cannot tolerate oral supplementation. Note that oral absorption of iron may not occur until serum ferritin is below 30 to 50 ng/mL (human patients). Erythropoiesis improves, however, even in human patients whose transferrin saturation is above that diagnostic of iron deficiency (16%).33
Anemias other than that associated with CRD also may be associated with decreased levels of endogenous EPO in animals and thus might respond to supplementation. However, the potential application of these indications in veterinary medicine must be balanced by the lack of scientific support and the adversities that are more likely in animals compared with humans if rh-EPO is used (e.g., antibody production). These have been reviewed by Langston and coworkers27 and others.2,34 An enzyme-linked immunosorbent assay (ELISA) kit developed to detect canine EPO may be helpful in identifying the need for supplementation although access at the time of publication is not known.35
The most common nonrenal use of ESAs in humans is anticipation of autologous blood transfusion; use of rhEPO increased the number of autologous units collected, and pretreatment may decrease the need for allogenic transfusion therapy. Although the need is less clear, a similar approach should be of benefit in animals; experimentally, pretreatment with iron and rhEPO in dogs resulted in higher hematocrit levels after transfusion than those in pretreatment with iron and saline.27 Selected (nonmyeloid) cancer anemias associated with chemotherapy may respond to EPO supplementation, including multiple myeloma and myelodysplastic diseases (the latter defined on the basis of cytopenia, dysplastic hematopoietic precursor cells and less than 30% blasts in the bone marrow).2,27,34 Anemia associated with cancer may reflect an inadequate EPO response; in humans the ratio of measured to predicted EPO (the latter based on population studies) of less than 0.9 is interpreted as an indication of potential response. Response may be related to dose: One retrospective study of multiple myeloma in humans reported a higher overall survival in patients who received a high dose (greater than 60,000 U/week) rather than a lower routine dose.36 The use of ESAs in human cancer patients has been reviewed,38 and guidelines for such use have been published.38,39
A combination of rhEPO and immunosuppressive therapy apparently was not successful in cats with pure red cell aplasia (not associated with previous rhEPO therapy) but may have prolonged survival rates of dogs with myelofibrosis.27 An interesting and potentially relevant application of ESA is for patients in the intensive care unit. In human patients in the intensive care unit, blood loss, including that associated with repetitive phlebotomy for diagnostic testing, can be significant. The veterinary patient—particularly the smaller one—may be similarly predisposed. Relative EPO deficiencies in these patients further predispose to anemias, as is indicated by transferrin saturations of less than 20% in the majority of patients. The use of rhEPO decreases the number of transfusions needed in these patients. The use of exogenous EPO in viral immunosuppressive diseases is tempting but controversial and might best be based on demonstration of endogenous EPO deficiency. Whereas endogenous EPO appears to be low and thus exogenous therapy is of benefit in human patients with HIV-associated anemia, EPO concentrations are increased in feline patients with feline leukemia virus.27 However, the PCV increased after 2 weeks of rhEPO in nonanemic cats positive for feline immunodeficiency virus; antibodies did not appear with this short-course therapy. Other uses of rhEPO in dogs have been characterized by variable success. The use of rHEPO in racing animals is likely to be associated with the production of antibodies and therefore is discouraged not only for ethical and legal reasons but also for health reasons. Human growth factors (granulopoietin and EPO) were associated with bone marrow recovery in a dog with ehrlichiosis.40 However, therapy took several months before response in peripheral counts were realized. The use of EPO in patients with pancytopenia associated with parvovirus is controversial, particularly in light of one case of fulminant infection in a human patient with immunodeficiency who was subsequently treated with rhEPO.41 Evaluating response of drug-induced bone marrow depression to rHEPO is complicated by the impact of discontinuing the inciting drug.
Therapeutic Failure
The most likely reason for a patient not to respond to rhEPO is inappropriate therapy. If the anemia is not associated with CRD, rhEPO therapy may be ineffective if endogenous rhEPO concentrations are maximally increased. Failure in a patient with low endogenous rhEPO may occur for several reasons. The dosage may be insufficient. Failure of an anemic animal to respond to rhEPO may indicate the development of antibodies directed toward rhEPO, as previously discussed.22 Animals that develop antibodies should recover, with antibodies potentially resolving within 3 to 4 weeks in dogs. Recovery may depend in part on the severity of renal disease, with recovery less likely in those animals with severe renal disease. In humans pure red cell aplasia as a result of antibody formation may be treated with a low dose (3.3 mg/kg) of cyclosporine.6,42 Differences in vehicles, stabilizers, and handling were identified as possible contributors to autoantibodies in human patients with renal disease who were receiving recombinant EPO; changes in response to these problems has dramatically reduced the incidence of antibody formation.16 Response to ESAs is likely to require iron supplementation.22 Chronic inflammatory disease decreases response to rhEPO, as do myelophthisic diseases that replace bone marrow with fibrous tissue. Alternatively, anemia may persist because patient nutrition is not sufficient to support increased EPO.
Granulopoietin and Related Products
At least six growth factors influence granulopoiesis.2 Formation of both granulocytes and macrophages from multipotential stem cells is stimulated by stem cell factor, a glycoprotein produced by bone marrow stromal cells; IL-1 and IL-6, inflammatory cytokines that mediate many of the systemic manifestations of acute inflammation; and IL-3. GM-CSF, along with G-CSF, is produced by a variety of tissues in response to cytokines such as IL-1, TNF, and endotoxin.2,5 Supplementation with exogenous factors stimulates the proliferation of various cell types, with the type affected depending on the factor or combination of factors. IL-3 increases both platelets and granulocytes, and GM-CSF administered by itself stimulates granulocyte and macrophage proliferation. When given in combination with IL-3, however, GM-CSF stimulates thrombopoiesis, and when combined with EPO and IL-3, erythropoiesis is stimulated as well.43
As with EPO, recombinant products have been developed for therapeutic stimulation of granulopoiesis. Both recombinant canine (rcGSF) and recombinant feline (rfGSF) G-CSF have been cloned, but neither is commercially available at this time. Feline GSF has much closer homology with rcGSF than with rhGSF and thus is the preferred product. Despite their lack of availability, the factors are increasingly being studied for treatment of neutrophil disorders in dogs and cats.5,44
In normal dogs G-CSF (5 μg/kg per day) will increase neutrophil counts over fourfold within 24 hours, reaching a maximum of approximately 72,000/mL by 19 days, with counts returning to normal within 5 days after discontinuation of therapy.44 In dogs afflicted with cyclic neutropenia, rcGSF (2.5 μg/kg every 12 hours) prevents neutropenia and associated clinical signs, although cycling of neutrophils is not prevented. Chemotherapy-induced neutropenia (induced by mitoxantrone) was minimized in dogs receiving rcGSF for 20 days. Studies in normal cats receiving rcCSF reveal an approximately threefold increase in neutrophil count that persists until the drug is discontinued. No adverse effects occur.44 Cohn et al.45 demonstrated that plasma G-CSF concentrations increased just after the onset of neutropenia in puppies (n = 8) experimentally exposed (oronasal) to parvovirus. Neutrophil counts rebounded within 2 to 3 days, and G-CSF concentrations decreased before neutropenia resolved, questioning the benefits of exogenous therapy. However, recombinant human G-CSF did correct canine cyclic hematopoiesis in one study.46
Other compounds have been studied for their effect on granulopoiesis. An extract of Serratia marcescens activates interferon- α and interferon-γ, as well as IL-1, IL-6, and GM-CSF, and induces myeloproliferation either directly or through release of other cytokines.44
Vincristine
Vincristine has demonstrated efficacy in the treatment of thrombocytopenia. Its mechanism is not clear, but several have been proposed. These include decreased upregulation of glycoprotein receptors on platelets, thereby reducing interaction with von Willebrand factor (vWF) and platetet aggregation, or reduction in immunglobulin G (IgG) autoantibodies that damage endothelial cells or suppress von Willebrand factor–cleaving protease activity.47 Other mechanisms suggested in the veterinary literature have included impaired macrophage phagocytosis of platelets and effects at the bone marrow (increased megakaryocyte fragmentation and stimulation of thrombopoiesis).48 For acute episodes of thrombocytopenia purpura in humans, vincristine treatment has been considered a salvage treatment (1.4 mg/m2 followed by 1 mg on days 4 and 7).49 However, its success in these patients has led to more frequent use as first-choice therapy. A retrospective study in humans found patient outcome (survival) to be better when vincristine therapy was administered initially as opposed to 3 days into treatment.50 The authors subsequently encourage the early, initial use of vincristine as part of combination therapy, including traditional modalities. Dogs with severe immune-mediated thrombocytopenia (IMT; platelet count less than 15,000/μL) appear to be respond similarly. In a prospective study, Rozanski and coworkers48 found that platetet counts in dogs with IMT increased more rapidly in patients that received prednisone (1.5 to 2 mg/kg/day) and vincristine (0.02 mg/kg) as opposed to prednisone alone. Further, animals refractory to prednisone alone for 7 days responded rapidly when vincristine was added to therapy. However, all dogs also were treated with doxycycline (10 mg/kg/day) for 7 days. Because IMT in dogs is characterized by increased antiplatelet antibodies on platelet surface, response to vincristine might be expected in this syndrome more than other causes of thrombocytopenia. Because vincristine is a relatively safe drug, initial therapy with vincristine should be considered in dogs with moderate to severe IMT.
Treatment of Disorders of Hemostastis
Anabolic or Androgenic Steroids
Chemistry
Anabolic steroids are synthetic compounds structurally related to testosterone. They have protein-anabolic activity similar to that of testosterone but ideally minimal androgenic effects (i.e., minimal masculinization) (Table 15-2).51,52 Testosterone is the sole endogenous androgen in most mammals. Its androgenic effects and rapid elimination have led to the manufacture of synthetic compounds. Chemical manipulations that have produced clinically useful anabolic steroids include (Figure 15-4) (1) alkylation (addition of a methyl [CH3] group) at the 17β position (e.g., methyl-testosterone, stanozolol), which impairs hepatic metabolism, thus prolonging elimination half-life; (2) esterification of the 17β hydroxyl group (e.g., Deca-Durabolin) such that absorption from parenteral sites is prolonged; and (3) modification of the steroidal ring structure. The sequelae of changes in the steroidal structure vary with the modification and include prolonged absorption or elimination.
Mechanism of Action
The mechanism of hematinic action of anabolic steroids on the cellular level is typical of the steroidal compounds.52–55 Anabolic steroids enter the RBCs, where they enhance glycolysis and the formation of steroidal 17-keto metabolites. These metabolites are delivered to tissues, including the bone marrow, where they interact with a cytosolic receptor in the appropriate cell and are transferred to the cell nucleus. In the nucleus they induce the formation of RNA and synthesis of an effector protein that brings about the pharmacologic effect.
The sequelae of steroid-induced nuclear transcription are manifold.54,55 The proposed sequelae on RBC formation include (1) stimulation of EPO production by way of EPO-stimulating factor, (2) differentiation of stem cells into EPO-stimulating factor–sensitive cells (e.g., hemocytoblasts), and (3) direct stimulation of erythroid-progenitor cells. Anabolic steroids also increase intracellular concentrations of 2,3-bisphosphoglycerate in erythrocytes; oxygen release into tissues is subsequently increased.
Efficacy of the anabolic steroids on the RBC mass depends on the presence of supportive materials.54,55 This includes adequate concentrations of androgen dehydrogenase in the RBC, adequate EPO concentrations, and sufficient bone marrow cellularity. Thus the effectiveness of anabolic steroids in treating anemia may be limited depending on the cause (i.e., renal disease accompanied by low EPO levels). Administration of high doses of these steroids may cause a negative feedback inhibition.
Pharmacologic Effects
The difference in the pharmacologic effects of these drugs among the various tissues and, specifically, whether an androgenic versus an anabolic effect will predominate cannot be attributed to differences in target tissue receptor structure because there appears to be only one androgen receptor type. Differences in response may be concentration dependent (e.g., reproductive tissues requiring higher concentrations than nonreproductive tissues), with drugs differing in their binding to the androgen receptor. However, mibolerone has little anabolic effect despite tight receptor binding and methylation; in contrast, boldenone, which is not methylated, has marked anabolic activity, attributed to a double bond at positions 1 and 2 (see Figure 15-4).56
The effect of several anabolic steroids in renal EPO was studied in the perfused canine kidney exposed to 4 hours of hypoxia.57 Activities important to secretion of EPO included a double bond at position 4 and 5 and the absence of a methyl group at position 10 (see Figure 15-4). Alternatively, differences in response may reflect conversion of the drug by target tissues to an active metabolite that subsequently causes the pharmacologic effect.54,55 In the presence of continued administration, anabolic steroids initiate and maintain a positive nitrogen balance, although the response is short-lived in intact males.52,54,55 The anabolic effects of stanozolol have been studied in dogs. Based on urine excretion of urea, retention of intravenously administered radiolabeled amino acid was increased in sled dogs (n = 10) receiving stanozolol either 2 mg/dog orally twice daily (increased from a baseline of 30% to posttreatment retention of 50%), or 25 mg weekly for 4 weeks (increased from 27% to 67%). The authors58 concluded that such an effect might be beneficial with acute or chronic conditions characterized by protein loss. Anabolic steroids antagonize glucocorticoid-induced protein catabolism by competitively inhibiting glucocorticoid binding to glucocorticoid receptors. Although anabolic steroids appear to vary in their ability to antagonize the effects of glucocorticoids, protein anabolism may be induced in patients experiencing glucocorticoid-induced protein catabolism. Anabolic steroids also reduce urinary excretion of nitrogen, sodium, potassium, chloride, and calcium.
As part of their anabolic activity, these compounds increase the circulating RBC mass (and possibly granulocytic mass). Red blood cell indices, hemoglobin, and hematocrit increase in various types of anemia. White blood cell mass may increase in cases of pancytopenia, although white blood cell response takes longer than the RBC response. Response by thrombocytes is slower and less predictable.52,54,55
Pharmacokinetics
The absorption and disposition of anabolic steroids depend on the type of preparation, the presence of specific receptors, and the species to which it is administered.52,54,55 Most anabolic steroids depend on hepatic metabolism for elimination, with those metabolites that are anaologs of the parent compound accompanied by metabolic activity.56 The risk of abuse in athletes (including the racing animal) has led to metabolic profiling of some steroids. Among the steroids, Beagle hepatic microsomal metabolism of steroids yielded hydroxylated and oxidized metabolites testosterone, methyltestosterone, mibolerone, and boldenone, with testosterone a metabolite of boldenone and androstenedione a major metabolite of testosterone (see Figure 15-4).
Preparations
Anabolic steroids have been divided into two categories depending on the presence or absence of an alkyl (CH3) group at the 17-carbon position, although the relevance of this division is probably less important than the impact of the individual drugs (see Figure 15-4).54,55 Oral and parenteral preparations are available, with the alkylated products being better absorbed orally. Oil-based parenteral preparations are intended for slow release. Examples of alkylated anabolic steroids include methyltestosterone, (oral), oxymetholone (oral), stanozolol (oral and parenteral), methandrostenolone, ethylestrenol, and norethandrolone (see Table 15-1). Examples of nonalkylated anabolic steroids include testosterone cypionate or enanthate, oxymetholone, and nandrolone in its decanoate form (parenteral).
Toxicity Versus Efficacy
A review of risks in human athletes includes cardiovascular adversities, increased risk of cancer, behavioral disorders, and increased risk of tendon damage.59 Hepatotoxicity is the most common serious adverse effect associated with androgenic or anabolic steroids.54,55 Toxicity ranges from mild increases in clinical laboratory tests to hepatocellular carcinoma (in humans). Drugs alkylated at the 17-carbon position are more likely to cause hepatotoxicity (see Figure 15-4). Although the mechanism is not known, the drugs or their metabolites may be carcinogenic or may increase the metabolism of other drugs to carcinogenic or hepatotoxic compounds. Cholestatic liver damage occurs early and can be significant but is apparently reversible if the drug is discontinued before irreversible hepatic lesions develop. It is not clear if the hepatotoxic effects that occur in humans are likely in dogs or cats.
Masculinization is a major undesirable (or desirable) side effect of anabolic steroids in humans.52,54,55,59 Virilization can occur but is seldom objectionable in dogs or cats. In dogs and cats, anabolic steroids increase libido in males, interfere with the female reproductive cycle, and cause masculinization of fetuses if administered during pregnancy. Other undesirable consequences of anabolic steroid therapy in dogs include hyperplasia of the perineal glands and stimulation of androgen-dependent tumors, such as anal carcinomas and prostatic carcinomas. In humans edema resulting from water retention occurs.52,54,55
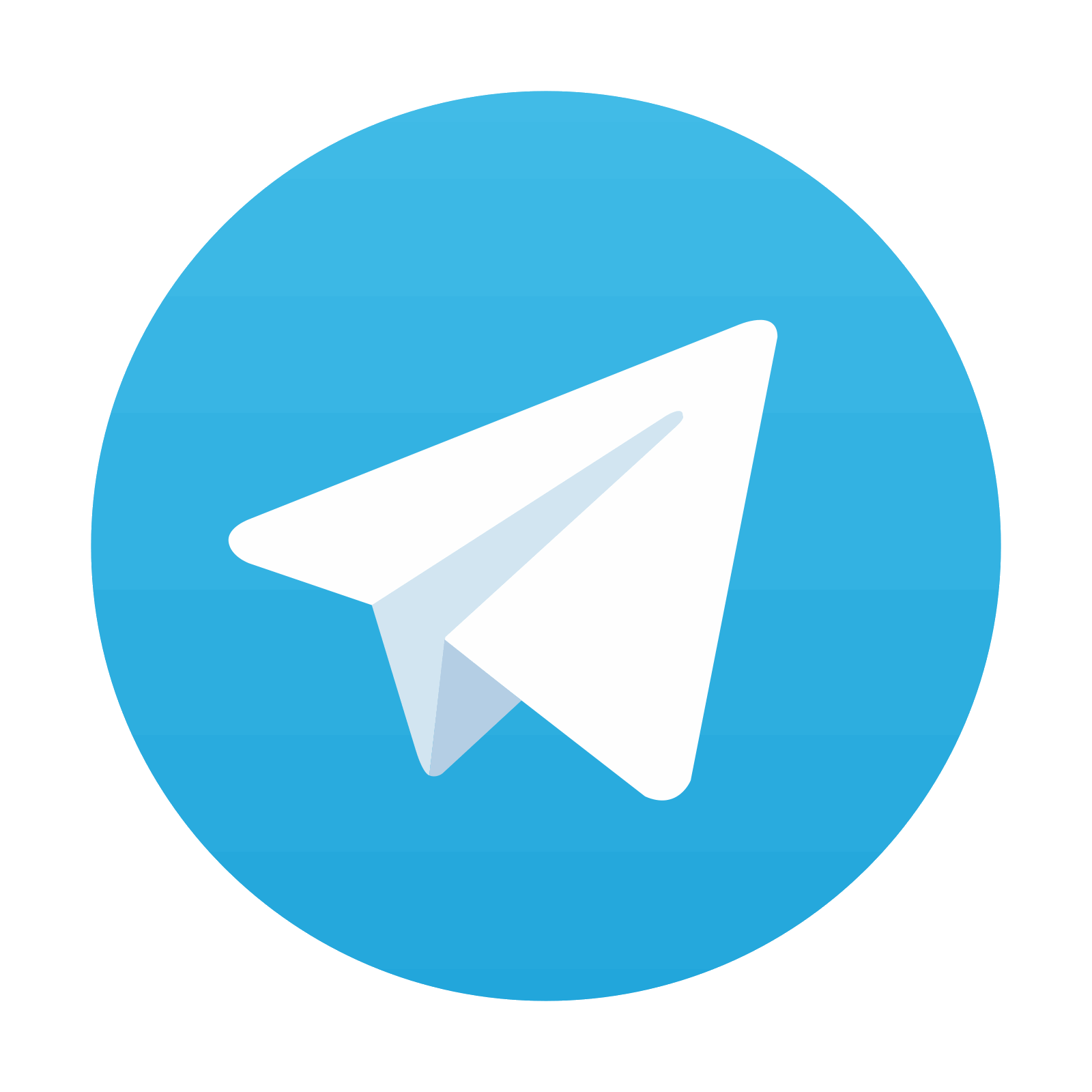
Stay updated, free articles. Join our Telegram channel
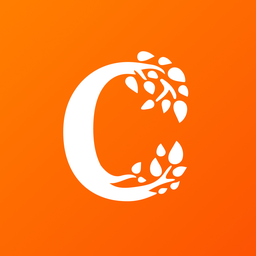
Full access? Get Clinical Tree
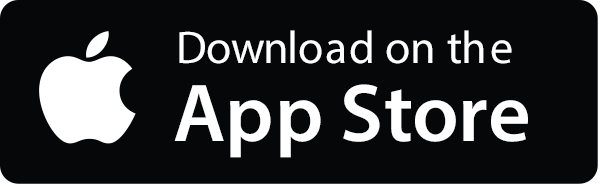
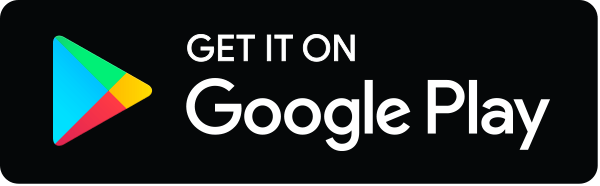