CHAPTER 11 Disorders of the Musculoskeletal System
STRUCTURE
Mammalian skeletal muscle consists of approximately 75% water, 18% to 22% protein, 1% carbohydrate, and 1% mineral, with variable lipid content. Depending on the breed and type of horse, between 44% and 53% of the live weight of a mature 500-kg horse has been estimated to be muscle.1 The myofiber, or individual muscle cell, is the fundamental building block of muscle, and each is a fusiform, multinucleated cell. Combined, myofibers constitute 75% to 90% of the volume of muscle. The myofibers are arranged in parallel along the length of the muscle such that the force of contraction is additive. The rest of the muscle tissue is made up of fibroblasts, capillaries, adipose cells, nerves, and connective tissue fibers. The composition of any muscle varies depending on the muscle surveyed and the overall fitness level, age, and breed of the horse. Muscles begin and end with tendons of various sizes, which attach the muscle to bone. Golgi tendon organs act as end organs of muscle sense and are found in the major tendinous origins and insertions. The nerve and blood vessels that supply an individual muscle typically enter near the midpoint of the muscle belly at a region called the neurovascular hilum. The nerve bundle splits off into individual nerves once it enters the muscle. A single nerve contacts several myofibers such that they contract in unison when stimulated (motor unit). A single nerve innervates each myofiber at a single point known as the motor end plate.
Within each myofiber are parallel arrays of myofibrils, the basic contractile units of muscle. Myofibrils are composed of end-to-end stacks of rodlike structures or contractile units called sarcomeres. Each stack of sarcomeres forms a long filament, and arrays of filaments form the myofibrils. Sarcomeres are composed of the contractile proteins actin, myosin, tropomyosin, and troponin. Troponin is a complex of troponin T, troponin I, and troponin C, whereas myosin is made of heavy and light chains and a globular head that contains the myosin adenosine triphosphatase (ATPase). Figure 11-1 shows an electron micrograph of several myofibrils. The alternating bands of light and dark are notable. The dark A band is composed of the thick myosin filaments and is transected by a darker line called the M line. Each myosin filament is surrounded by, and interdigitates with, six thin filaments composed of actin, tropomyosin, and troponin. Areas where the thin filaments are not overlapping with myosin form the lighter I band. The dark line running across the I band is called the Z line. A sarcomere is defined as the region between each Z line. At the Z lines the protein α-actinin anchors actin. Because of the differing way these structures absorb light, the regular alternation of A bands and I bands in adjacent myofibrils produces the striated appearance characteristic of skeletal muscle fibers as viewed with light microscopy. Mitochondria fit into the small spaces around the myofibrils and produce the adenosine triphosphate (ATP) necessary for the contraction-relaxation cycle. The number, size, and shape of mitochondria vary depending on the fitness of the individual and the muscle fiber type predominant within the muscle sampled.1–3
MUSCLE CONTRACTION
Contraction
Calcium released into the sarcoplasm binds to troponin C, resulting in a conformational change of the tropomyosin molecule and unmasking part of the actin filament responsible for binding to myosin: the G-actin monomer. With binding of myosin to the G-actin monomers, a 90-degree cross-bridge forms. As soon as the cross-bridge forms, myosin ATPase cleaves ATP to form adenosine diphosphate (ADP) and adenosine monophosphate (AMP), resulting in another conformational change converting the 90-degree angle to a 45-degree angle. The contractural force is generated by the movement of the cross-bridge myosin head from a 90-degree to a 45-degree angle with a coincident sliding of the myofilaments relative to one another toward the center of the sarcomere. The hydrolytic products of ATP then detach from the myosin head enabling the cycle to recommence. The addition of new ATP to the myosin molecule results in the rapid dissociation of the actin and myosin filaments.4,5 Sustained contraction occurs with the rapid repetition of this mechanicochemical cycle.5
Relaxation
For the cell to relax and return to baseline polarity, the sarcoplasmic reticulum and the plasmalemma must reaccumulate calcium, thereby lowering the concentration of calcium in the sarcoplasm and the T tubule while sarcolemmal membranes begin to repolarize. Sarcoplasmic calcium concentrations decrease when calcium-magnesium ATPase pumps return calcium to the sarcoplasmic reticulum and sodium-potassium ATPase pumps move sodium extracellularly and potassium intracellularly. Energy-dependent reaccumulation of calcium by the sarcoplasmic reticulum results in the release of calcium from troponin C, restoration of the resting configuration of the troponin-tropomyosin complexes, and ultimately disruption of the linkage between the myosin and actin filaments. Further details on muscle structure and contraction are available.4–7
Source of Energy for Contraction
When the phosphate bond of ATP is cleaved, the resulting by-products include ADP, inorganic phosphate, and energy. At the onset of muscle activity, cleavage of the phosphate bonds of creatine phosphate (or phosphorylcreatine) in the presence of ADP by the enzyme creatine kinase produces creatine and ATP. This anaerobic pathway is quickly supplanted by other pathways to produce energy (Table 11-1).
Glycolysis and glycogenolysis are rapid processes and produce adequate energy for aerobic exercise. When exercise intensity increases and oxygen demand cannot be met, glucose is metabolized anaerobically. Anaerobic glycolysis of glycogen yields 3 moles of ATP and lactic acid as a by-product. The accumulation of lactic acid within the cell lowers the pH of the sarcoplasm, inhibits the function of many intracellular enzymes, and contributes to fatigue.
FIBER TYPE
Differentiation
Under light microscopy using simple staining techniques, skeletal muscle appears to be a homogeneous tissue. However, muscle is composed of many fibers with differing characteristics. Myofibers may be grouped into two categories: type 1 and type 2. Table 11-2 gives a summary of the characteristics of different fiber types. The type 1 and 2 classification is broad, and a spectrum of fibers has characteristics of both categories. The differences between fibers arise from variability in the proteins that comprise the contractile elements. Several different isoforms have been identified for the myosin heavy and light chains, tropomyosin, troponin I, troponin T, and troponin C. The greatest variability is in the isoform of the myosin heavy chain. In addition, the calcium pumping capacity of the sarcoplasmic reticulum may vary from cell to cell.
All types of fibers are found in most skeletal muscles. Type 1 fibers are high in oxidative capacity and therefore rely on glucose, free fatty acids, and a high amount of oxygen to supply energy for contraction. Their sarcoplasmic reticula have a moderately slow reuptake of calcium capacity. Therefore type 1 fibers are best suited to moderate contraction speeds and sustained contractions over long periods. As expected, these fibers predominate in muscles involved in posture. Type 2 fibers have a fast myosin ATPase rate and a rapid capacity to reuptake calcium into the sarcoplasmic reticulum. They are large-diameter fibers compared with type 1 fibers and have a higher glycolytic capacity, making them suited to powerful fast contractions. These fibers predominate in the skeletal muscles involved in locomotion and rapid fine-motor movements such as around the eye. Type 2 fibers that have activities closer to type 1 fibers are called type 2A, whereas those that exhibit high anaerobic capacity are characterized as type 2B. Because of the high oxidative capacity of type 1 fibers, these muscles often appear to have a deeper red color than fibers high in type 2A and 2B fibers.
The varying capacities of muscle fiber types is largely the result of variations in myosin heavy-chain isoforms. These isoforms are identified through antibody and electrophoresis techniques. In mammals, at least nine have been identified. Two are developmental (embryonic and neonatal), two are found in cardiac muscle (alpha and beta), three are common in skeletal muscle (2a, 2b, and 2x), and two are rare with greatest expression in jaw, extraocular, and laryngeal muscles. Beta is also found in skeletal muscle and is an isoform with properties of slow twitch, high oxidative capacity, low glycolytic capacity, and low ATPase activity similar to the histologically identified type 1 myofibers. Type 2a myosin heavy-chain isoforms are typically found in fibers exhibiting fast twitch, high glycolytic, high ATPase activity, and high oxidative capacity and as such resist fatigue. Type 2b and 2x isoforms are found in muscles that are fast twitch, produce strong contractions, have high glycolytic and ATPase activity but have low oxidative capacity and, as such, fatigue faster than type 2a rich myofibers.8–11
Innervation
A neuron and all of its innervated myofibers constitute a motor unit. Each motor neuron may innervate a few or several hundred myofibers, and all of those myofibers exhibit similar contraction characteristics. Each myofiber, however, is innervated only by a branch of one motor neuron. Two types of motor nerve fiber are found, slow and fast. The type and pattern of electrical activity on a muscle cell is related to the type of myosin isoforms expressed: The large phasic motor nerve fibers innervate the type 2 myofibers, whereas the small tonic fibers innervate the type 1 fibers.11
Distribution
The proportions of fiber types present within a muscle vary according to individual traits of the horse, the muscle location, the breed, and the age of the horse.1,8,10,12–15 In some muscles (in particular the middle gluteal muscle, which is sampled most commonly) the distribution also depends on the sampling site or depth because the distribution of fiber types is nonhomogeneous.1,10,15,16 The suggestion has been made, however, that within a specific muscle of an individual, the variation in fiber types is small if samples are taken from the same site or an identical contralateral site under controlled conditions.15,17 This arrangement is why it is generally recommended to biopsy specific muscles to diagnose certain disorders. It provides a relatively homogeneous baseline from which to evaluate a muscle. The exception is when a specific muscle is affected (e.g., exhibits atrophy) wherein it should be biopsied.
GROWTH, TRAINING, AND PERFORMANCE
Fiber type predominance does affect performance. However, with growth and training muscle cells adapt and may change fiber type over time.1,7,18–22 Training results in (1) an increase in the ability of a fiber to use oxygen by increasing the number of mitochondria within the sarcoplasm, (2) a decrease in the use of muscle glycogen and blood glucose with a greater reliance on fat oxidation to supply ATP, and (3) a decrease in the amount of lactate produced per given intensity of exercise. Variable results have been reported on the effects of exercise on fiber size and capillarization. The extent and nature of the changes appear to depend on the duration, intensity, and type of exercise involved, as well as the age of the animal. Over the last decades, much information has been gained in the field of equine exercise physiology, and multiple texts are available on the subject both in print and online that extend beyond the scope of this chapter.23–26
PATHOLOGIC CHANGES
General Response of Muscle to Injury
Muscle responds to injury or damage in a limited number of ways. A growing body of evidence indicates that myopathic conditions share a similar final pathway of muscle fiber degeneration, although the number and type of fibers affected and the degree of damage varies.27 The final common pathway involves failure to sequester calcium ions from the sarcoplasm. Prolonged calcium accumulation within the sarcoplasm leads to activation of cellular enzymes and prolonged activation of myofilaments. This process is visible histologically as hypercontraction. Consequently, mitochondria take up excess calcium in an attempt to prevent cellular damage. Accumulation of calcium within the mitochondria leads to failure of energy production (i.e., ATP) within the cell and eventually failure of all intracellular energy-dependent mechanisms, which leads to failure of membrane pumps and ultimately cell swelling and lysis.27,28
The exact nature of these calcium-activated degenerative pathways is still unknown. A key step may be calcium-induced membrane phospholipid hydrolysis via the activation of phospholipase enzymes, resulting in the production of tissue-damaging metabolites. Other processes, however, involving nonenzymatic lipid peroxidation also may be activated. During exercise the flow of oxygen increases. At the same time an overall depletion of ATP sources occurs. The resultant metabolic stress leads to a greatly increased rate of oxygen free radical production that may exceed the scavenger and antioxidant defense systems of the cell, leading to a loss of cell viability and damage. The increase in free radical activity can lead to a failure of calcium homeostasis and consequent muscle damage, or, alternatively, calcium overloading may lead to an activation of free radical–mediated processes.29 Multiple initiators likely lead to this final common pathway, depending on the type of exercise, fitness level, presence or absence of underlying genetic defects in muscle metabolism, and nutritional status of the horse. Free radical–induced skeletal muscle damage may be especially important if reperfusion follows a period of ischemia.30
Atrophy and Hypertrophy
Atrophy may be defined as a decrease in muscle fiber diameter or cross-sectional area. Atrophy can occur in a variety of circumstances, including denervation, disuse, and cachexia, in many muscle diseases, after circulatory disturbances, and after extensive myolysis. Within 2 or 3 weeks after peripheral denervation, up to two thirds of the original muscle mass may be lost, although this may not always be obvious clinically because of the presence of superficial and intramuscular fat deposits. Histologic changes reflect the affected nerve and the muscle fiber supplied. In disuse (e.g., atrophy resulting from a tenotomy) a preferential atrophy of type 1 fibers is apparent, often with hypertrophy of type 2 fibers. In contrast, with cachexia and malnutrition, type 2 fibers are preferentially affected, especially in the postural muscles. Not all muscles show the same degree of atrophy, even within one disease process. In cachexia, the back and thigh muscles tend to be the first affected, and the loss of muscle is usually symmetric, with a concurrent loss of fat deposits. A localized asymmetric atrophy is associated with paralysis, immobilization, and denervation.31
As indicated previously, hypertrophy may occur through training. A compensatory hypertrophy may also occur in the fibers surrounding an area where fibers have been lost or have decreased greatly in size. Often, large fibers are visible histologically in such conditions, with evidence of incomplete longitudinal division.31
Repair after Denervation
Denervation results in atrophy, as remarked previously, but also leads to abnormal muscle excitability. Muscle becomes more sensitive to circulating acetylcholine. The result is the presence of fine, irregular contractions or fibrillations detectable using electromyography. Fibrillations are not visible clinically and should not be confused with fasciculations that appear grossly as jerky muscle contractions in response to pathologic spinal motor neuron discharges.11 With reinnervation the fibrillations cease. Reinnervation can occur in two ways. Damage without severing the nerve is called axonotmesis. The axon is damaged but the Schwann cell and endoneural fibrous sheaths remain. Axons from the proximal part of the damaged nerve may reestablish connections within the empty residual Schwann cell sheath to the distal portion of the original nerve. Initially, atrophy of scattered muscle fibers is visible, but after reinnervation a proportion of the muscle fibers are restored to their normal size and function. Even if the affected muscle is not fully restored, other surrounding unaffected muscles may be able to compensate so that overall function is maintained, although slight gait abnormalities may remain.
Repair after Injury
Regeneration where a break in the continuity of the muscle fiber has occurred differs considerably from neurologic damage. In such cases, repair involves multiplication of nuclei and formation of new internal structures and organelles followed by fusion and alignment into a new multinuclear myofiber. Regeneration can be swift when parts of the affected fiber remain intact. In this case, regeneration occurs at the healthy end of the severed fiber (continuous or budding regeneration). When damage is more severe, regeneration occurs by fusion of mononuclear myoblasts to form a myotube, which develops in a similar way to fetal fibers (discontinuous or embryonic regeneration). The origin of myoblasts is primarily satellite cells, and their origins and function have been well reviewed.32,33 If the scaffolding, basement membrane, and supporting tissues remain intact, and if the initiating disease process subsides, new fibers tend to orient in a way similar to the original fibers. This type of regeneration usually occurs with segmental necrosis in which necrosis of the whole diameter of the fiber has occurred involving several sarcomeres. Fibroblastic and vascular reactions are minimal in this type of regeneration. Therefore full function of the muscle is restored without residual dysfunction.
DIAGNOSIS OF SKELETAL MUSCLE DISORDERS
Skeletal muscle disorders broadly fall into two categories: acquired or inborn. Acquired myopathies include those of traumatic or infectious origin and ones arising secondarily from endocrine or nutritional disease or electrolyte imbalances (acquired metabolic myopathies).34 Inborn errors of metabolism that manifest as metabolic myopathies originate primarily from defects in muscle energy metabolism. As such, they can be grouped as errors in carbohydrate, lipid, or purine metabolism; as mitochondrial defects; or as primary disorders of ion channels (channelopathies).
Plasma and Serum Enzyme Activities
Increases most commonly occur because of a defect in the integrity of the membrane containing the enzyme-rich sarcoplasm.6,35 The defect results from complete disruption of the cell or a transient change in the permeability of the membrane without cell death. A complete explanation for the pattern of release of intracellular constituents from diseased muscles cannot be given here. Most of the enzymes that are detectable in increased concentrations in the blood with the various muscle disorders are the major soluble (sarcoplasmic) enzymes, although one can also detect the mitochondrial form of AST with severe injury.
Creatine Kinase
Creatine kinase is the enzyme responsible for breaking down creatine phosphate to creatine and phosphate, releasing energy for muscular contraction. This reaction is the sole source of energy in muscle at the initiation of exercise. Thereafter, energy is supplied by oxidation of glucose and free fatty acids as described previously. Because this enzyme is responsible for the breakdown of creatine phosphate, it is often called creatine phosphokinase. This term, however, is inappropriate, and one should use creatine kinase. In the horse, CK is found mainly in skeletal muscle, the myocardium, and the brain.36 Little or no exchange of CK between the cerebrospinal fluid and plasma appears to occur. A significant increase in total plasma CK activity therefore is caused by cardiac or skeletal muscle damage. CK (molecular weight, 80,000 daltons [Da]) does not enter the bloodstream directly after its release from the muscle cell but transits through the lymph via the interstitial fluid. The total quantity of circulating CK in the horse under normal conditions is estimated to be equivalent to the quantity of CK in approximately 1 g of muscle; a threefold to fivefold increase in plasma CK activity corresponds to the apparent myolysis of approximately 20 g of muscle.37 However, as described previously, making assumptions as to the amount of muscle damaged based on serum CK activity is difficult because muscle cells appear to be able to release significant quantities of CK without necessarily being lysed.
In human beings, two monomers of CK are known and are designated M and B. The enzyme is dimeric, and three possible primary forms exist: MM, MB, and BB. In simplified terms, MM is found mainly in skeletal muscle, BB in the brain and epithelial tissues, and MB in the myocardium. In the horse, some confusion over CK isoenzymes exists, with workers reporting different electrophoretic bands and tissue activities, perhaps because of the different techniques used.38–41 In one study the heart and skeletal muscle were found to contain predominantly the MM dimer; the brain, pancreas, and kidney, mainly the BB dimer; and the intestine, the MB and BB dimers.39 This work suggests that in the horse, CK isoenzymes on their own could not be used to differentiate between skeletal and cardiac muscle damage. This problem is alleviated largely by the current availability of tests for cardiac troponin I. Unfortunately, commercial tests for skeletal troponin I are not available. In the absence of clinical cardiac disease, elevations in CK primarily can be attributed to skeletal muscle. The plasma half-life of CK in the horse is short (108 minutes, 123 ± 28 min with a plasma clearance of 0.36 ± 0.1 ml/kg/min)37,42 in contrast to reports of 12 hours in human beings.43
Aspartate Aminotransferase
AST is found mainly in skeletal muscle, liver, and heart, although lower activities are present in several other tissues. Therefore AST is not tissue specific.36,44,45 Two isoenzymes have been identified by electrophoresis: MAST (found exclusively in the mitochondria) and CAST (originating from the cytoplasm or sarcoplasm). The ratio of cytosolic to mitochondrial enzyme in horse serum is significantly greater than that found in human beings and many other mammals.46 In the horse, the ratio of these two forms varies between tissues, and no tissue specificity is apparent for either isoenzyme. The plasma half-life of AST in the horse is 7 to 10 days,42 far longer than the 11.8 hours in human beings.43
Lactate Dehydrogenase
Lactate dehydrogenase is a tetrapeptide that occurs in five different isozymic forms produced by combinations of two kinds of subunits (H [heart] and M [muscle]) in groups of four to produce the functional tetrameric molecule. The five isoenzymes are labeled as LDH1 to LDH5, or H4, MH3, M2H2, M3H, and M4, respectively. Similar to AST, LDH is found in most tissues and is therefore not organ specific. However, tissues contain various amounts of the LDH isoenzymes, and the isoenzyme profile obtained by electrophoretic separation has been used to identify specific tissue damage.47 For the most part, LDH5 (plus some LDH4) is found in the locomotor muscles, the liver contains mainly LDH3 (with some LDH4 and LDH5), the heart contains mainly LDH1 (with LDH2 and LDH3), and all types have been found in certain nonlocomotor muscles.38,41 Training has been shown to increase the percentage of LDH1 to LDH4 and decrease that of LDH5 in skeletal muscle.48 The practitioner should use nonhemolyzed samples for LDH determinations because red blood cells contain large amounts of LDH. In general, though, assays for CK and AST are most commonly used to evaluate skeletal muscle.
Urinalysis: Myoglobinuria
Myoglobin (molecular weight 16,500 Da) is essential for the transport of oxygen into and within muscle cells. Most mammalian muscles contain approximately 1 mg myoglobin per gram of fresh tissue, and the suggestion has been made that acute destruction of at least 200 g of muscle must occur before serum myoglobin levels rise sufficiently for detection in the urine.49 Serum myoglobin has a short half-life, and therefore measuring serum myoglobin is of limited diagnostic value. In human beings, myoglobinuria occurs in a variety of conditions, including myocardial infarction, crush and burn injuries, malignant hyperthermia, idiopathic and exertional rhabdomyolysis, and certain genetic metabolic abnormalities.6,49–52 In the horse, myoglobinuria has been seen in equine exercise or trauma-induced rhabdomyolysis,53,54 white muscle disease in foals,55 and postanesthetic myositis.56
Pigmenturia or dark urine may be caused by increased myoglobin, hemoglobin, or whole blood in the urine. Low levels of myoglobin, hemoglobin, or whole blood may occur in the urine and can be detected by urine dipstick or laboratory test before visible changes in the color of urine. Therefore considerable amounts of myoglobin, hemoglobin, or whole blood must be present for visible pigmenturia to occur. Many causes of hemolysis in the horse can result in hemoglobinuria, including oxidative damage to erythrocytes,57 neonatal isoerythrolysis, hepatic disease, and renal disease.59
Unfortunately, stored urine, concentrated urine, and urine containing myoglobin, hemoglobin, or other porphyrins can appear similar in color57,59,60 (Figure 11-2). Therefore myoglobinuria cannot be distinguished by color alone. The orthotoluidine-impregnated strips commonly used by veterinary clinicians (urine dipstick tests such as BM-Test-8, Boehringer Ingelheim Pharmaceuticals, Ridgefield, Conn.) are insensitive to the presence of myoglobin and hemoglobin.54,61 The differential salting out of hemoglobin with ammonium sulfate gives false results in human beings49,50,59,62 and the horse but is used commonly in many laboratories.49,50,59,61,62 Visual inspection of the serum or plasma may indicate the cause of the pigmenturia. The low affinity of myoglobin for haptoglobin means that myoglobin is excreted at plasma concentrations around 0.2 g/L, whereas hemoglobin only appears in the urine at plasma concentrations greater than 1.0 g/L. At this concentration a pink discoloration of the plasma occurs, indicating that hemoglobin is present.9,49,50,63
On electrophoretic separation on cellulose acetate, myoglobin migrates as a β2-globulin and hemoglobin as an α2-globulin. This method can distinguish the two proteins in urine containing high concentrations (>125 μg/ml) of either protein, provided no other proteins, apart from albumin, are present. Immunoassays are the most sensitive and specific tests and can be used for detecting small amounts of myoglobin in blood and urine.61,64,65 Sequelae of acute tubular necrosis and acute renal failure are associated with myoglobinuria in human beings66 and horses.44,63 Renal disease associated with myoglobinemia in the horse seems to be associated most with horses that are highly stressed (causing decreased renal blood flow), dehydrated, or have lactic acidosis associated with rhabdomyolysis. Therefore horses suffering from “capture” myopathy (e.g., cast in a stall or trailer accident and struggling for a prolonged period) and endurance horses are most at risk.
Muscle Biopsy
The practitioner can obtain muscle biopsy samples by surgical excision under general or local anesthesia63 or by percutaneous needle biopsy from the standing horse.9,14,67 Because of the variation in fiber composition in muscles, which muscle is chosen, as well as the position and depth of sampling, is important. Good specimen preparation for frozen sections is vital to prevent artifacts such as ice crystals.6,9,68 For laboratories that prefer examination of frozen sections, specimens are typically shipped chilled overnight so that the laboratory may freeze the sample to minimize artifacts. Other laboratories prefer formalin-fixed specimens. The practitioner should discuss sample procurement, preparation, and shipment procedures with the laboratory before biopsy.
Muscle biopsy allows the morphologic, biochemical, and physiologic properties of the myofibers to be examined with the animal still alive and causes little morbidity. Typical sites used for percutaneous biopsy with a 6-mm modified Bergstrom needle in cases of generalized muscle disease or for research purposes include the semimembranosus, the biceps femoris, and most commonly the middle gluteal muscle. Although convenient, the necessity to have a modified Bergstrom needle means that most practitioners opt for open biopsy of the semimembranosus muscle over percutaneous middle gluteal muscle biopsy. Instructions on how to obtain a muscle biopsy are available online.69
Histochemical staining of frozen muscle sections aids in the detection of glycolytic enzyme deficiencies and storage of various atypical metabolic compounds.6,70–72 Pathologic changes that can be found in muscle include presence of degenerate or regenerate myofibers, abnormal cytoplasmic inclusions, accumulations of polysaccharide, changes in fiber volume, number, shape, malformation, degeneration or proliferation of organelles, and disruption of the basic architecture of the fiber. Aggregates of inflammatory cells, reactive changes in vessel walls, occlusion of blood vessels, and increased amounts of fibrous tissue are other possible pathologic findings. In some conditions, such as tetanus or botulism, no significant changes may be seen. Further information on the types of changes that occur is available.6,31
Immunocytochemical studies have been used to investigate neuromuscular disorders in human beings and to diagnose autoimmune streptococcal-associated myositis in the horse.73,74 Specialized staining techniques help to localize specific enzymes and intracellular and extracellular muscle components such as the various collagen and myosin types, complement, and fibronectin. Electron microscopic studies enable investigation into the ultrastructure of muscle fibers.
Electromyography
Needle EMG is the study of the electrical activity of muscles. EMG may be useful in horses with unusual gait or muscle changes and can be used to discriminate between neurogenic and myogenic causes and more specifically to identify areas or regions of an abnormality.75 A recording needle electrode is placed into the muscle, and the electrical activity is amplified, recorded on an oscilloscope, and projected audibly into a loudspeaker. The electrical status of muscle membranes depends on the integrity of the whole motor unit. Thus an EMG evaluates the function of the ventral motor horn cell, its axon, axon terminals, and neuromuscular junctions, as well as the muscle fibers it innervates.
Fasciculations, visible muscle twitching, are caused by the spontaneous contraction of some or all of the constituent fibers of a motor unit. They can be local or generalized and occur primarily with any cause of muscular weakness (myasthenia), especially in neurogenic disorders such as tetanus and certain debilitating and metabolic disorders. In addition, one can assess the electric activity induced by electric stimulation of nerves or associated with voluntary or induced muscle contraction. Further information on EMG is available.76
Thermography
Abnormalities have been found in exercise-exacerbated focal thoracolumbar gait abnormalities, as well as disuse atrophy.77 The technique has been useful in documenting hindlimb muscle strain as a cause of lameness in horses.
Scintigraphy
Bone-seeking radiopharmaceuticals have been used to detect and localize skeletal muscle involvement, especially in poor performance cases, but are of limited routine value in the field. Accumulation in damaged muscle may be related to the deposition of calcium in damaged fibers, binding by tissue hormones or enzyme receptors, tagging to denatured proteins, or altered capillary permeability. Uptake of labeled phosphates appears to occur only when muscle damage is ongoing and does not occur in areas of repair. Three main types of muscle uptake were identified in one study of horses with skeletal muscle damage: a diffuse, severe, and generalized uptake; bilateral symmetric uptake involving muscle groups that perform synergistic functions; and asymmetric radioisotope uptake in one or more muscle groups on one side of the animal.78 The reasons for the various patterns have not been elucidated fully. Scintigraphy can be used as part of a series of tests to identify atypical or difficult-to-pinpoint lamenesses in horses that may have primary muscle lesions among other problems.79,80
Exercise Tests
Certain physiologic changes can result in a transient alteration in cell membrane permeability. Hypoxia, catecholamines, hypoglycemia, changes in pH, and altered ionic concentrations have been reported as causing such a change in membrane permeability.45,81,82 Many of these changes are believed to act by decreasing the amount of ATP available for the maintenance of cell integrity, which becomes especially important during exercise.83
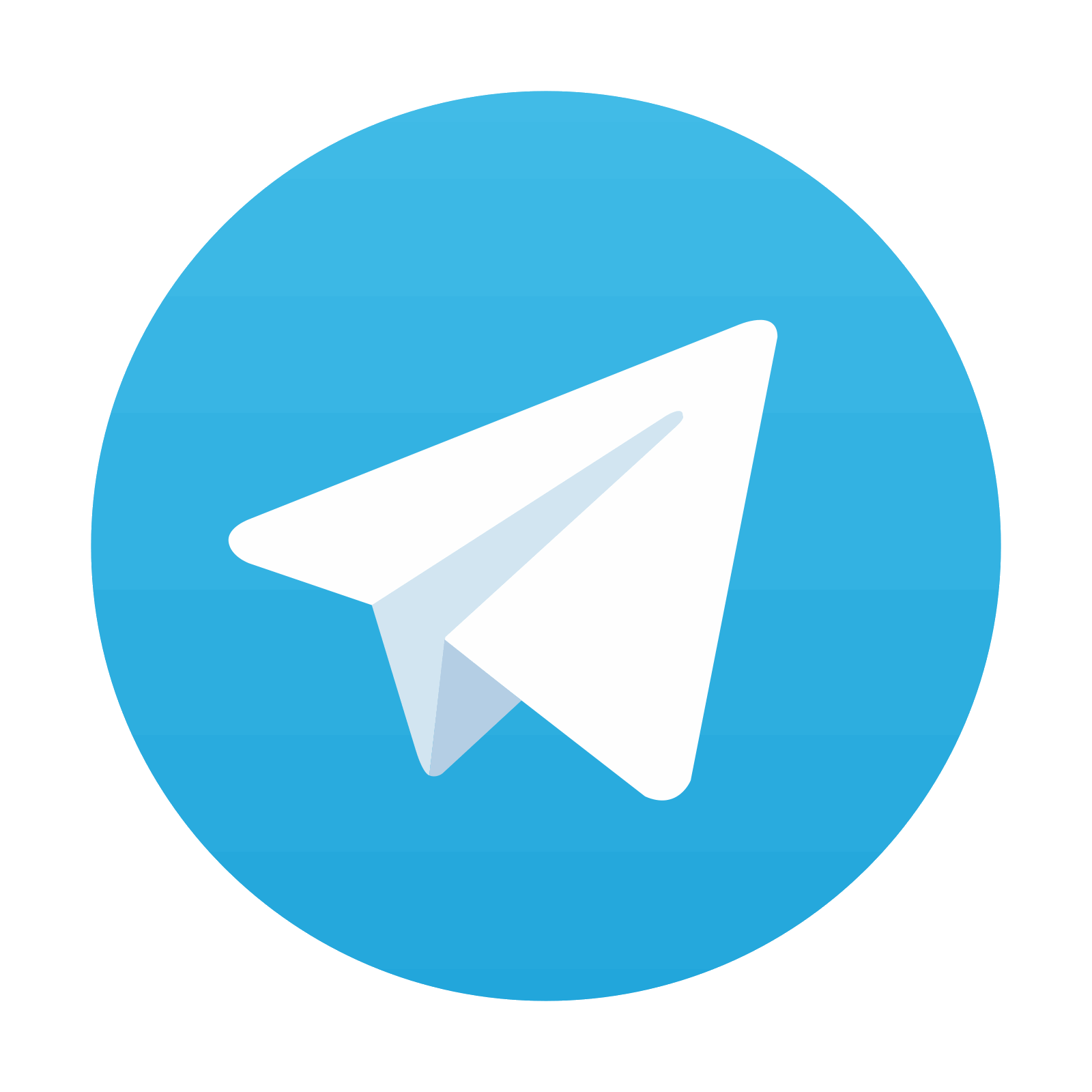
Stay updated, free articles. Join our Telegram channel
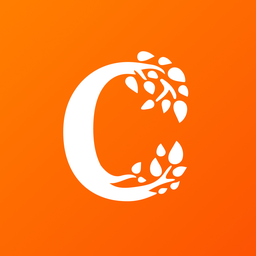
Full access? Get Clinical Tree
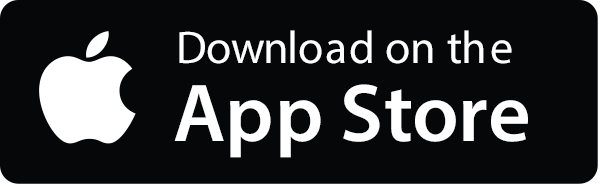
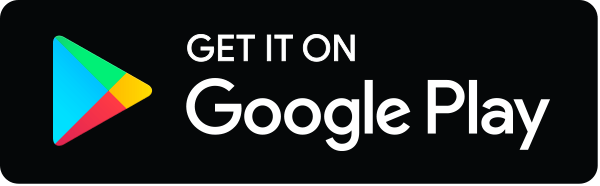