The Spine’s Role in Normal Gait
The requirements that determine the anatomy of the spine are diverse and complex. The spine must support the head, support the abdominal contents, allow for propulsion while coordinating limb movement, and be flexible to a limited extent, while all the time protecting an extremely delicate structure, the spinal cord (Figure 16.1).
Figure 16.1 Cross section through the spine showing the relationship between the vertebra, intervertebral disk, nerve roots, and spinal cord.
Illustration by Marcia Schlehr.

Most veterinary descriptions of the spine are about mechanical failures of the spine that result in spinal cord injury. The canine athlete is so dependent upon the spine working properly that the structural functions assigned the vertebrae and the associated soft tissue cannot be ignored. Although there is a large body of literature about the workings of the canine spine, the focus has been almost exclusively toward applications for spinal conditions in humans.
This chapter concentrates on the functions of the spine specifically. Spinal cord conditions are discussed in general terms. Detailed descriptions of spinal pathology affecting the spinal cord and spinal cord diseases are readily available in numerous veterinary publications (Birchard, 2006; Dewey, 2008; Jaggy, 2010).
Interactions of the Head, Thoracic Limbs, and Pelvic Limbs
The Head
The head, in upright, bipedal primates is a rather large heavy structure whose weight is carried by a stack of relatively thin vertebrae. It is interesting to note that in bipeds, in which the head is not suspended cranial to the body, the thoracic dorsal spines are small. In the dog, however, the head is cantilevered away from the body and needs to be suspended by structures over the forelimbs, which thus carry more weight than the pelvic limbs. Numerous spinal components are necessary to support this load efficiently. The nuchal ligament is a thick band of elastic fibers that help support the head with minimal expenditure of energy. The nuchal ligament extends cranial to a large spinous process of the second cervical vertebra and attaches caudally to the most dorsal portions of the dorsal spines of the first few thoracic vertebrae (Figure 16.2). The fibers of the nuchal ligament actually can be followed caudally into the ligaments that attach to the dorsal prominence of each vertebral body’s dorsal spine. This ligamentous continuation of the nuchal ligament is called the supraspinous ligament. The thoracic spines are attached to each other by way of the interspinous ligaments. They allow the force of the weight of the head to dissipate through the dog’s body but still maintain a level of flexibility. These nonmuscular components form a very efficient system where muscular energy is conserved. The cervical vertebrae are large and proportional to the weight of the head. The forces required to suspend the head are complemented by absorption of compressive forces placed upon the cervical intervertebral disks.
Figure 16.2 Photograph of the cervical spine demonstrating the variations in conformation (A) and the large dorsal thoracic spine needed to support the head (B).
Illustration by Marcia Schlehr.

The first and second cervical vertebrae have rather unique conformations that serve two significant purposes. As the nuchal ligament is attached to the second cervical vertebra’s large and wide dorsal spine, the forces required to keep the head in various positions require these forces to be dissipated over a large surface. Fan-shaped muscles, predominately the splenius, attach to the nuchal crest of the caudal skull and, like the nuchal ligament, arise from the cranial thoracic vertebrae’s dorsal spines. This conformation also acts as a cantilever allowing the head to rotate and be supported by the compressive forces placed upon the atlanto-occipital joint (Figure 16.3).
Figure 16.3 A. Photograph of the base of the skull showing the large occipital condyles that attach the skull to the cervical spine and the broad nuchal crest that is an important muscular attachment. B. The first (atlas) and second (axis) cervical vertebrae disconnected to demonstrate how C2 fits into and joins C1. C. An end-on view of the most rostral cervical spine demonstrating the C1–C2 articulation and the large atlanto-occipital joint.

The joints and articular facets of the C3–C7 cervical vertebrae are quite large and play a significant role in controlling fine movement of the head and neck in three planes. Their role in support of the neck is unknown, but they may play a minor role, as their removal does not significantly affect the biomechanics of the neck (Crisco et al., 1990).
The head initiates all purposeful movement not only by determining the proposed activity through mentation, but also by determining the position that the body occupies in space through the vestibular apparatus within the skull. “Where the head goes, the body must follow” is a common phrase among rehabilitation therapists. It is understood that head position and movement initiates the next body movement and allows for appropriate body mechanics. Visual and olfactory input as well as reflex modification are important but are not critical for the vestibular system to perform its duties, but may be critical for developing training routines. Better understanding of how the neural feedback loops initiate, maintain, and protect propulsion and, in this case, spinal-related structures, is critical to attain peak performance.
The Thoracic Limbs
The thoracic vertebrae, as previously stated, play a major role in maintaining the head’s normal positions in space. The most cranial thoracic vertebrae with their large dorsal spines form the anchor that allows the head to be suspended at some distance from the body (Figure 16.4). These cranial thoracic vertebrae are supported directly by the thoracic limbs. The weight of this entire unit is supported by the forelimbs, and it is often noted that this represents 60% of the body weight of the animal. Considering the tremendous variation in conformation that exists from breed to breed and from individual to individual, this would have to be a very rough estimate.
Figure 16.4 Lateral view of the cervical and rostral thoracic vertebrae demonstrating the functional anatomy described.

The thoracic limbs do not have bony or ligamentous attachments to the spine. The extrinsic forelimb muscular apparatus attaches the forelimb to the thoracic body wall, allowing for freedom of movement that permits extreme changes in direction while protecting the spinal column’s rigidity throughout the area of the rib cage.
The Pelvic Limbs
The pelvic limbs are responsible for carrying the weight of the caudal portion of the body. The pelvic limbs interact with the body in a completely different manner from the thoracic limbs (Figure 16.5). The coxofemoral joint transmits forces to the caudal spine through the sacroiliac joint. Although the boxlike structure formed by the pelvis has less flexibility than the muscular attachment of the forelimb to the body wall, there is a significant rotational advantage afforded by the range of motion (ROM) of the ball and socket joints. This also allows the pelvic limbs to move far forward under the body producing significant forward propulsion. When turning, a great deal of the actual body movement has been committed by the trajectory of the head, and the actions of the forelimbs and thoracic cavity. The limited flexibility of the spine allows for fluid movement once propulsion is initiated.
Figure 16.5 Ventrodorsal radiograph of the pelvis and femurs showing the box-like nature of the pelvis and the ball and socket joints of the femurs.

The tail, like the head, is cantilevered from the body. The apparatus necessary to carry this weight is not extensive, but the tail has a role in balance and fine movement that has been extremely well documented in the cat (Walker et al., 1998). Although not as well studied, the canine tail also serves to help with balance during locomotion (Wada et al., 1993).
Conformational Considerations
Each vertebra interlocks with the adjacent vertebra cranial and caudal to it. Except for the first and second cervical vertebrae (Figure 16.3B), the components of each individual vertebra are similar, but each varies as the morphology of that site is determined by its functional requirements (Figure 16.6). The first few thoracic vertebrae have the tallest dorsal spines, as they are the scaffold that carries the weight of the head. These forces are transmitted through the vertebral joint, muscle, and ligamentous structures. The lowest point of the thoracolumbar spine is usually at the point at which the tenth and eleventh thoracic dorsal spines meet. At this site, the dorsal spines acutely reverse direction from a caudal sweep (T10) to a dorsal sweep (T11). The anticlinal vertebra is the one where this change occurs and is usually but not always T11. A fibrous ring, the fibrous annulus, which is composed of strong cross fibers that firmly attach one vertebra to another, interconnects the vertebral bodies. The central portion of this ring is filled with a gelatinous substance, the nucleus pulposus, a remnant of the phylogenetically primitive notochord. The nucleus pulposus absorbs forces created between the vertebral endplates and dynamically transfers those forces to the surrounding fibrous ring in response to dorsoventral and lateral movements of the spine. We do not usually appreciate the dynamic nature of these structures as they are often viewed through still-motion studies such as radiography, computed tomography (CT), and magnetic resonance imaging (MRI). With motion, the gel becomes compressed at the side of narrowing of the disk space and expanded at the side that widens as the spine routinely and regularly changes from one angle to another through all gaits. Faster gaits will also involve greater changes in dorsoventral movements as the pelvis is alternately tilted to gain more distance with each stride. This spinal flexibility is dramatically demonstrated in the canter and gallop gaits.
Figure 16.6 From left to right, a photograph of a cervical, thoracic, and lumbar vertebra showing the tremendous variation in conformation in the same animal.

The interspinous ligaments stabilize the dorsal spines of the vertebrae. The articular surfaces, which are extensions of the dorsal lamina of each vertebra, form joints with the vertebra both cranial and caudal. The cranial articular surface is ventral to the caudal articular surface of the vertebra cranial to it (Figure 16.7). All of these intervertebral articulations allow for a significant but limited motion that permits the spine to bend in all planes. The sacral vertebrae are fused and have the least flexibility in any plane. The thoracic wall and rib attachments limit the mobility of the thoracic vertebrae (Figure 16.8). The chest wall, which consists of the interconnecting vertebrae, ribs, and associated soft tissue, forms a stable element that serves to anchor the more flexible and mobile cervical and lumbar regions of the spine. The increased mobility at the cervicothoracic, thoracolumbar, and lumbosacral junctions in comparison to other spinal segments is quite significant. The forces produced at these junctures are most likely responsible for a higher degree of degeneration at these sites.
Figure 16.7 Photograph of several lumbar vertebrae showing how they articulate and the appearance of the joint surfaces when disarticulated.

Figure 16.8 Lateral radiograph of the thoracic spine showing the ribs and chest wall that give it its rigidity.

The atlanto-occipital and atlanto-axial joints are unique and allow for tremendous freedom of movement of the skull. This is critical so that the extremely important special senses are able to harness information from the widest possible area. Head mobility is extremely important, as the mouth is the dog’s main means of defense. The occipital condyles are extremely large and allow for considerable dorsoventral ROM of the head. The odontoid process, bound to the floor of the atlas, and the joints formed by the articular surface of the atlas and axis permit considerable rotation of the head in relation to the neck (Figure 16.3)
The muscles that act upon the spine are too extensive and numerous to enumerate here. They are classified as intrinsic muscles that help with the stability of the spine, such as the multifidus musculature, and extrinsic muscles that help in more complex motions within the axial skeleton or in the appendicular skeleton such as the iliopsoas muscle. One overriding factor that must be kept in mind is that all of these activities occur while the spinal cord remains protected.
Very little attention has been paid to the actual conformational variation that occurs among breeds and between individuals within a specific breed (Figure 16.9). Small dogs have large cervical spinal canals with what seems to be relatively thinner dorsal lamina for their size. The relative sizes of the spinal canal from L4 to the sacral vertebrae can vary tremendously. Sacralization of the last lumbar vertebra and lumbralization of the first sacral vertebra are very common. The tremendous variation evident in spines of our canine population makes it difficult to provide generalizations about function. Breeding for desirable conformations and developing training regimens for canine athletes should take into account these variations.
Figure 16.9 A. Lateral radiograph of a normal lumbar spine. B. Normal lumbar spinal column and sacrum.

Forces That Affect the Spine
It is quite obvious that the spine can rotate on one axis and move in all planes and in more than one plane at the same time at different points along its length. Although these motions are critical for day-to-day activities, very little information is available about the actual forces involved and the limits necessary to protect spinal structures, including the spinal cord, from damage.
A biomechanical evaluation of L3–L4 in canine cadavers (Figure 16.10) where most extrinsic soft tissue structures had been removed from the vertebrae was performed (Smith & Walter, 1988). Excision of the supraspinous and interspinous ligaments yielded a decrease in stiffness in flexion, an increase in the ROM of the interspace, and a decrease in the ultimate flexion bending strength by 62%. Panjabi et al. studied the in vivo affects of transecting the supraspinous and interspinous ligaments in the cervical spine at C4–C5 (Panjabi et al., 1988). In the cervical spine, this injury caused a decreased ROM. It is, however, difficult to compare these two studies as one involved cadavers with most soft tissues removed and the other examined live dogs.
Figure 16.10 Lumbar vertebrae 3 and 4 demonstrating the actual joints of the vertebral experiments described.

There are numerous publications examining the forces placed upon the articular facets and intervertebral disks of the canine lumbar spine. These studies were intended to study the effects that these forces might have upon the human spine, and many conclusions were found to be applicable to both species.
Wood et al. found that while walking, the dog’s L2–L3 vertebrae became 2–3 degrees more kyphotic compared to the standing position, and the average excursion between opposing facets was 3.4 ± 1.3 mm (Wood et al., 1992). Although it is well recognized that these motions and their limits protect the spine from excessive flexion and torsion, and help to stabilize the spine, the actual forces involved have not received much attention. Butterman et al. were the first to measure actual loads on the facet joint in vivo. They determined the load on the articular joint surface of the L3 cranial articular facet (Buttermann et al., 1992). Using strain gauges, Butterman et al. measured the forces of the right L3 articular surface of five mixed-breed dogs in various positions and performing various activities. They verified their findings using two in vitro studies. A newton (N) is an internationally accepted unit of force that represents the force necessary to provide a one-kilogram mass with an acceleration of 1 m/s.
Their results demonstrate the relative forces placed upon the facets under varying conditions. Remember, only the right L3 cranial facet is being measured.
To put these numbers in perspective, the force measured from a human prosthetic hip while walking is 800–2200 N. Comparing the force per unit area of the human coxofemoral joint, the measurement would convert to 1–6 MPa (megapascals). Since the facet joint is considerably smaller, the forces over the L3 articular surface convert to a maximum of 2 MPa (megapascals). A megapascal is 106 N/M2. It is worth noting that although the joint forces on the cartilaginous structures in humans and dogs are in the same range per unit area, the cartilage in the dog is considerably thinner. Although we do not usually consider joint function in terms of newtons and megapascals, these relationships provide starting points from which to consider improving treatment and training outcomes and considerations for the causes of spinal degeneration.
In the Buttermann et al. (1992) study, there was considerable variability between individuals. The authors believe this was due to differences in anatomy, muscle mass and distribution, motion patterns, and individual animals’ motivation to perform the tasks. The variations between individual dogs, which affected the results of that study, are often reflected in the training of dogs to complete tasks and to successfully compete. These individual variations require our attention if we are going to train the successful athlete or competitor.
Several other interesting points were noted. The force on the joint surface remained virtually constant regardless of the speed with which the subject was walking. The results were similar to the findings of in vitro studies. The caudal portion of the joint surface was rarely loaded unless there was extreme extension of the spine or the intervertebral disk had been compromised.
Breit followed up on this study by comparing the facet geometry of the canine thoracolumbar spine using three distinct groups of dogs (Breit, 2002). Breit divided his 140 dogs into three groups: large, chondrodystrophic, and small breeds. His findings increase our awareness of the variations between breeds and individuals. Torsional strain between vertebrae is determined by the transverse distance between the articular surfaces (Figure 16.11). In small breed and chondrodystrophic dogs, the transverse distance was very consistent when adjustments were made for size. In these cases, the facets consisted of a lateral and a ventral component that made the joint surfaces rather flat. In all large breeds, the transverse distance was considerably less, adjusted for size, and these joints were completely different in conformation. All of the large breeds had a caudal facet component that made their articular joints virtually ball and socket joints. This design would be able to handle the much greater forces that their increased mass would absorb. Interestingly, none of the small breeds had caudal facet components but some chondrodystrophic breeds did. The increased stability of this conformation might be a desirable trait that could be heritable.
Figure 16.11 Third lumbar vertebra. Arrow indicates the transverse distance between joint surfaces, which plays a major role in many of the force measurements referenced.

Lastly, there are several studies using the dog as a model that evaluate the mechanical properties of the intervertebral disk. Most of these have been in vitro studies and most have examined the mid-lumbar vertebrae. Unfortunately, routine intervertebral disk failure leading to spinal cord compression is not nearly as common in the mid-lumbar spine as in the thoracolumbar region.
Zimmerman et al. determined mechanical properties of the canine intervertebral disk at two sites, L2–L3 and L5–L6. Compressive stiffness varied at each site requiring 717.8 N/mm at the L2–L3 interspace and 949.0 N/mm at the L5–L6 interspace (Zimmerman et al., 1992). Torsional stiffness also varied in each case. At the L2–L3 interspace, it was 1.04 Nm/degree and at L5–L6 it was 1.72 Nm/degree. Axial or compressive stress on the intervertebral disk at L2–L3 was 14.03 MPa and at L5–L6 it was 16.30 MPa. Adjusting for size, these forces are similar to what is seen in people and the differences reflect the fact that the caudal lumbar spine plays a greater role in spinal stabilization. Interestingly the torsional stress measured in the dog at both sites was considerably higher than in people at 30.8 MPa for L2–L3 and 26.17 MPa for L5–L6.
Unfortunately, results of studies using the dog spine as a model for understanding and treating human spinal conditions have not been incorporated into specific requirements for our canine athletes. They have dramatically illuminated the differences in breeds and among individual dogs within breeds, however.
The sum of these studies clearly demonstrates that specifics about spinal conformation can be measured and through genetics, specific training, and attention to detailed anatomy, better functioning spines are possible.
Congenital Predispositions
Malformations
Spinal anomalies are usually considered incidental findings. Additional ribs and absence of ribs as well as additional lumbar vertebrae are probably the most common vertebral anomalies. Occasionally, a lack of a transverse spine is noted and on occasion, the anticlinal vertebra is not T11 but one of the neighboring vertebrae.
Spina Bifida
Spina bifida is an uncommon malformation of the spine that is frequently an incidental finding. Spina bifida is an interruption of or incomplete fusion of the dorsal spinal arches. This can occur at several vertebral sites but is most often recognized at the level of the sacrum and sometimes involves L7. Spina bifida is most common in the screw-tailed breeds: Boston Terriers, French Bulldogs, English Bulldogs, and others. This malformation is sometimes associated with incomplete formation of the neural tube causing dysplasia of the spinal cord and protrusions of the meninges alone and occasionally the spinal cord. Dogs in which the sacral segments of the spinal cord are malformed are often presented for a lack of urinary and/or fecal control.
Caudal Occipital Malformation Syndrome
Caudal occipital malformation syndrome (COMS) has been described (Churcher & Child, 2000; Rusbridge et al., 2000; Rusbridge & Knowler, 2003). In this condition, there is crowding of the cerebellum within the caudal fossa of the skull. This malformation often leads to a fluid-filled tubular structure, a syrinx, forming within the cervical spinal cord, and in many cases hydrocephalus is seen (Figure 16.12). Flow dynamics and the Venturi effect are suspected of being responsible for the syrinx formation. These fluid-filled tubes can occur when the dogs are very young or develop as the dog ages. Affected dogs often present with neck pain and tetraparesis frequently affecting the forelimbs to a greater extent than the pelvic limbs. A peculiar obsessive scratching of the neck area is a common occurrence. This disease is more prevalent in certain breeds such as the Cavalier King Charles Spaniel and Brussels Griffon and in toy breeds, although occurrence in other breeds has been described.
Figure 16.12 Lateral MRI of a Cavalier King Charles Spaniel with caudal occipital malformation syndrome (COMS). Note the syrinx in the center of the spinal cord (arrow). The white color denotes fluid, as this is a T2 image.

Atlanto-Axial Instability
The second cervical vertebra, C2, is held in place by several ligamentous structures. The bony projection, the odontoid process or dens, of C2 attaches to the ventral floor of C1 and allows for rotation of the head within limits in each direction. Dogs that have atlanto-axial subluxations usually have a hypoplastic dens or aplasia of the dens. This condition occurs most commonly in toy and miniature breeds, and in most cases, clinical signs occur when the dogs are young. The spine of C2 is displaced caudally at an angle leading one to conclude that the nuchal ligament is what is giving the C2 subluxation its characteristic radiographic appearance (Figure 16.13).
Figure 16.13 Lateral radiograph of an atlanto-axial subluxation. Notice the tilt (arrow) of C2 away from C1 and the odontoid process (dens) riding up into the spinal canal.

Hemi or Block Vertebra
Hemi or block vertebrae are usually incidental findings seen on radiography involving the mid-thoracic vertebrae in the screw-tailed breeds (French Bulldogs, English Bulldogs, Pugs, etc.). These vertebrae are malformed and can have quite an anomalous conformation (Figure 16.14). They can be wedge shaped or fused and often cause an extreme angle to the spine. The vertebrae are foreshortened and cause crowding of the ribs. The spinal alignments often are severe, but the dogs usually do not manifest clinical signs of spinal cord dysfunction.
Figure 16.14 Lateral thoracic radiograph demonstrating hemi or blocked vertebral abnormalities in this French Bulldog. These vertebral changes were incidental findings. Note the shortening and malformation of the vertebral bodies leading to shortening of the dorsal intercostal spaces as well as malformations of the dorsal spines and lamina (oval). These are all common, usually incidental, findings.

Congenital Stenosis
Within the spinal column, there are two abrupt transitions of spinal mobility. These areas probably have the greatest forces placed upon them. Advanced imaging of these areas in affected individuals seems to reveal a smaller spinal canal than is normal for the diameter of the spinal cord and associated nerve roots (Fourie & Kirberger, 1999; Drost et al., 2002; da Costa et al., 2006). These changes are present from a very early age. At the level of C6–T1, many large and giant breed dogs seem to have very little space around their spinal cords. A similar loss of spinal canal area is seen in many breeds of dogs at the level of L5–S1. If the spinal canal is pathologically narrowed in younger animals, clinical signs usually occur before 1 year of age. These relatively stenotic spinal canals predispose affected animals to spinal cord compression. In large breeds that have stenosis in the caudal cervical spinal canal, abnormal gaits and postural deficits can be seen in dogs as young as 12 weeks of age. Although several names describe caudal cervical spinal cord impingement, stenosis of the spinal canal underlies these pathologic conditions.
On rare occasion, dogs present at less than 1 year of age with severe signs from lumbosacral nerve root entrapment. More typically, these dogs develop nerve root entrapment at various adult ages due to encroachment caused by degeneration and proliferations of the surrounding ligamentous and sometimes bony structures that further compromise an already stenotic spinal canal.
Many of these conditions are highlighted by dynamic advanced imaging (Figure 16.15). These conditions are often overlooked when only static representations such as radiographs are evaluated (Jones & Inzana, 2000).
Figure 16.15 A. Transverse CT with contrast of the C6 vertebra. Note the large amount of space surrounding the spinal cord (normal). B. Transverse CT with contrast of the C6 vertebra in a different dog. Note that there is no room around the spinal cord, and the spinal cord appears flattened (asterisk). Both figures are at approximately the same level of the spine and both dogs’ heads were positioned similarly. The dog in part B had significant clinical signs.

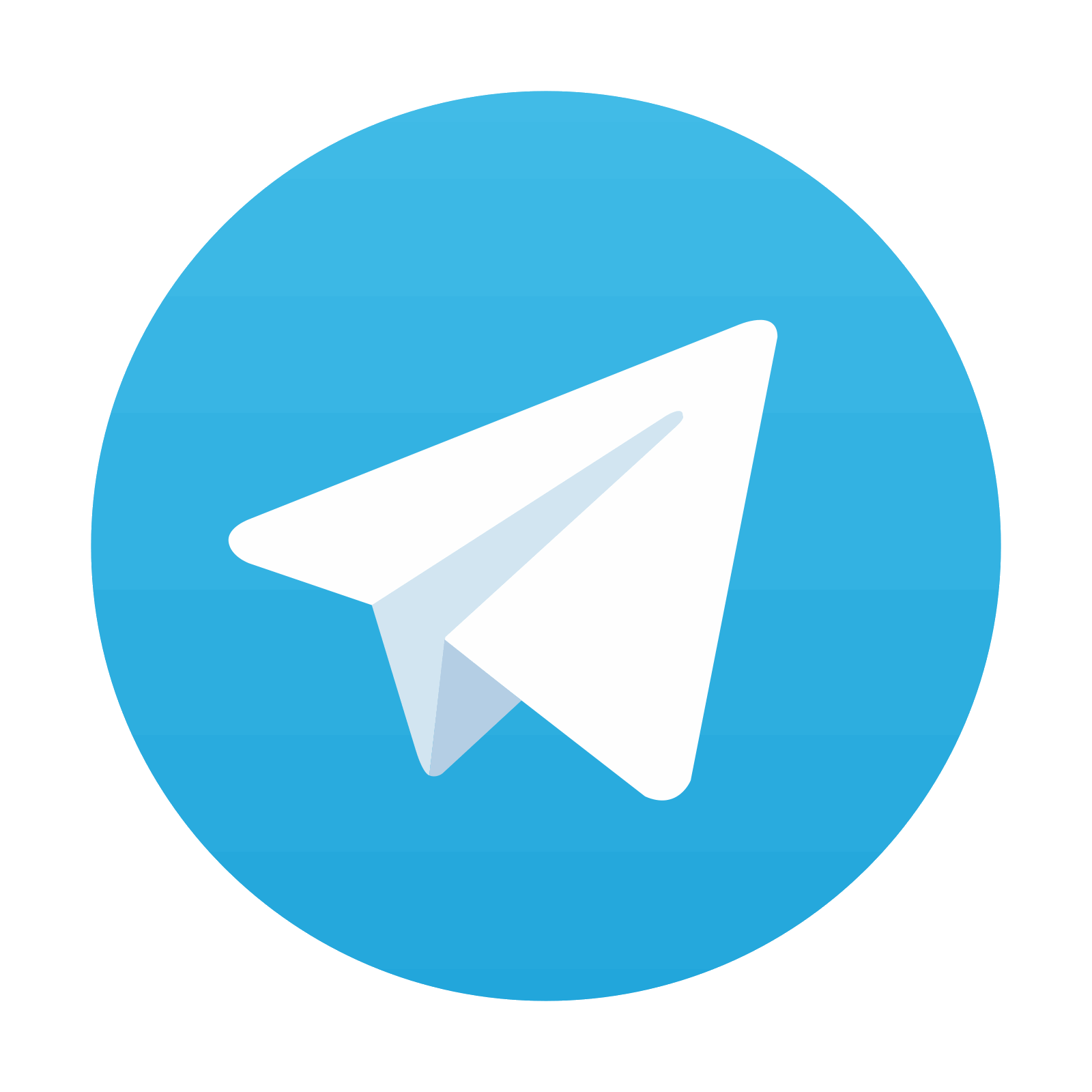
Stay updated, free articles. Join our Telegram channel
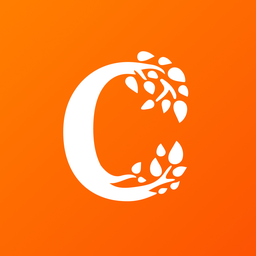
Full access? Get Clinical Tree
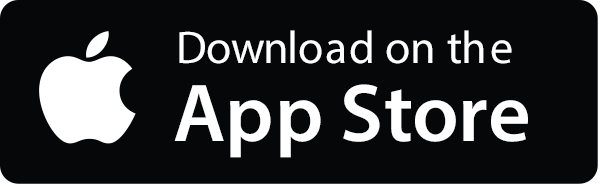
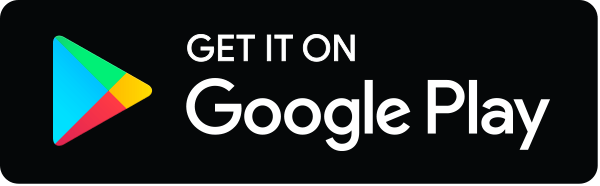