3 Determinants of Sperm Morphology Abdullah Kaya,1* Sema Birler,2 Lefric Enwall3 and Erdogan Memili4 Spermatozoa, or sperm cells, develop within the walls of the seminiferous tubules by a complex and time-consuming process known as spermatogenesis. During this process, primordial diploid ‘typical’ cells are transformed into unique, morphologically and functionally specific haploid cells. Following this process, and after release into the lumen of the seminiferous tubules and then passage into the epididymides, the spermatozoa undergo further maturational changes, e.g. acquiring the ability to be motile, but still undergo further physiological and biochemical changes in the female reproductive tract to acquire their final ability to fertilize ova. The unique morphological features of spermatozoa are critical for cell functionality. External influences, e.g. genetics, reactive oxygen species, temperature, hormonal modifications, external chemicals and DNA modifications, may negatively affect the production of normal spermatozoa. These factors are discussed in this chapter, but before doing so, a review of spermatozoa morphology is presented in order to provide an understanding of the significance of these external factors. Classical studies using light and electron microscopy have provided a basic understanding of the structural and functional features of mammalian spermatozoa. Also, many sperm abnormalities have been documented as associated with male infertility and sterility in most of the species studied. Although the size and shape of spermatozoa are different among different species, the main morphometric structures are similar (Sullivan, 1978). Mammalian spermatozoa consist of two major functionally dependent parts: the head and the tail. While the sperm head contains the materials necessary for fertilization and paternal DNA, the tail comprises the apparatus necessary for sperm energy production and motility (Sullivan, 1978; Roberts, 1986; Barth and Oko, 1989; Garner and Hafez, 2000). The sperm head is oval and flattened in shape and it is divided into two segments: the anterior acrosome and the posterior post-acrosomal region. The junction of the anterior and posterior regions is known as the nuclear ring. Underlying the anterior acrosome and continuing to the base of the head is the nucleus (Fig. 3.1). The largest component of the sperm head is the nucleus, which contains highly condensed DNA, the result of the involvement of sperm-specific proteins, the protamines (Ward and Coffey, 1991; Hazzouri et al., 2000). This condensed DNA state characterizes the cell as being non-dividing and transcriptionally inactive (Balhorn, 1982; Hazzouri et al., 2000; Johnson et al., 2011). Further, the compacted sperm DNA occupies a very small volume compared with the DNA in the mitotic chromosome (Ward and Coffey, 1991). The nucleus is surrounded by a special cytoskeletal complex of perinuclear theca called the post-acrosomal sheet (Fawcett 1975; Sullivan, 1978; Olson et al., 2002). The acrosome is a unique sperm organelle that develops from the Golgi apparatus during early stages of spermatogenesis. The acrosome is composed of an inner and outer membrane and a protease-filled matrix. In mammals, the acrosome overlays in a cap-like manner about 60% of the apical region of the sperm nucleus and head. It exhibits species-specific differences in shape, size and precise location on the sperm head (Fawcett, 1975; Mann and Lutwak-Mann, 1981). The acrosomal membrane that just underlies the plasma membrane is known as the outer acrosomal membrane, while that overlying the nucleus is called the inner acrosomal membrane. (Fawcett, 1975; Yanagimachi, 1994; Wassarman, 1999; Eddy, 2006). The posterior region of the acrosome is known as the nuclear ring or the equatorial segment. Because the acrosome develops during spermatogenesis from the Golgi apparatus, it has been referred to as a specialized lysosome. The posterior region of the sperm head (the post-acrosomal region) undergoes changes during post-testicular maturation in the epididymides. These maturational changes play a significant role in sperm-specific binding and fusion with the oolemma (plasma membrane of the oocyte) after sperm have penetrated through the corona radiata and zona pellucida following the acrosome reaction (Sullivan, 1978; Barth and Oko, 1989; Yanagimachi, 1994; Ellis et al., 2002 Olson et al., 2002). Enzymes that have been reported to be present in the acrosome include: hyaluronidase, proacrosin, acrosin, esterases, neuraminidase, acid phosphatase, phospholipases, aryl phosphatase, β-N-acetylglucosaminidase, arylamidase, collagenase and corona penetrating enzyme (CPE) (Allison and Hartree, 1970; Zaneveld and Williams, 1970; Mann and Lutwak-Mann, 1981; Barth and Oko, 1989; Eddy, 2006). The specific functions of some of these enzymes are well documented, while those of others are not. Hyaluronidase is released from the acrosome and plays a role in either the dispersal or the digestion of the cumulus oophorus extracellular matrix of the oocyte. CPE, which is also associated with the outer acrosomal membrane, plays a role in penetrating through the corona radiata of the oocyte. Acrosin is present in the acrosome as proacrosin; it is associated with the inner acrosomal membrane and is converted into acrosin. Acrosin is a trypsin-like enzyme that functions to allow penetration of spermatozoa through the zona pellucida during fertilization. Neuraminidase also evidently enhances passage of spermatozoa through the zona pellucida and the plasma membrane of the oocyte (Allison and Hartree, 1970; Zaneveld and Williams, 1970; Mann and Lutwak-Mann, 1981; see Gadella, 2013, and Chapter 4 for in-depth discussions on the physiology and biochemistry of sperm–oocyte interactions). The sperm tail consists of four segments: the neck (connecting piece), the midpiece, the principal piece and the endpiece. Each of these four segments is surrounded by a common cell membrane (Fawcett, 1975; Sullivan, 1978; Barth and Oko, 1989; Garner and Hafez, 2000; Olson et al., 2002; Eddy 2006). The primary structural parts of the mammalian sperm tail are the axoneme, the mitochondrial sheath, the outer dense fibres and the fibrous sheath. The centrally located axoneme is composed of nine evenly spaced microtubule doublets and a central pair of singlet microtubules. These extend throughout the length of the tail. The outer dense fibres have a very remarkable cytoskeletal structure, consisting of nine fibres that surround the axoneme and extend through the neck, the midpiece and principal piece of the mammalian sperm tail (Sullivan, 1978; Roberts 1986; Haidl et al., 1991; Mortimer, 1997; Garner and Hafez, 2000; Olson et al., 2002). Haidl et al. (1991) also reported that defects of the outer dense fibres result in abnormal sperm morphology in men. The helically arranged mitochondrial sheath surrounds the outer dense fibres in the midpiece, whereas only the fibrous sheath surrounds the outer dense fibres in the principal piece. Only the microtubules terminate in the endpiece (Fawcett, 1975; Mortimer, 1997; Eddy, 2006). The neck is a short connecting segment between the head and the tail of the spermatozoon (Fig. 3.2). The sperm head and tail are connected via the basal plate at the caudal end of the nucleus, and the capitulum, which adheres to the basal plate of the implantation fossa of the nucleus (Fawcett, 1975; Mortimer, 1997). The neck and the axoneme are formed by a pair of centrioles that are composed of nine circularly arranged microtubular triplets. These two centrioles are present in the spermatid at the time of sperm tail formation. The distal centriole forms the axoneme, whereas the proximal centriole is associated with the formation of capitulum (Barth and Oko, 1989; Mortimer 1997; Eddy, 2006). Thus, the connecting piece has a dual origin from both the proximal and distal centrioles (Gordon, 1972; Fawcett, 1975). The midpiece (Fig. 3.3) is the region of the tail between the neck and the annulus (Jensen’s ring). The annulus is a structural element of the mammalian sperm tail, which connects the midpiece and the principal piece. In the midpiece segment, the outer dense fibre–axoneme complex is surrounded by a helically-wrapped mitochondrial sheath, which extends from the neck to the annulus (Fawcett, 1975; Sullivan, 1978; Mortimer, 1997; Olson et al., 2002; Eddy, 2006). The mitochondria of the midpiece generate energy in the form of ATP, which is used for sperm locomotion. The inner mitochondrial membrane is the site of energy production (Mortimer, 1997). The elongated mitochondrial helix surrounds approximately 80% of the midpiece. There is a wide range of variation among mammals in the length of the midpiece and in the number of mitochondria present (Fawcett, 1975; Sullivan, 1978; Eddy, 2006). The principal piece is the longest part of the sperm flagellum and extends from the annulus to the terminal piece (Fig. 3.4). Due to the termination of mitochondria in the mid-piece, the diameter of the tail in the principal piece is reduced. The principal piece is characterized by the presence of a fibrous sheath, which provides stability for the contractile elements of the tail (Fawcett, 1975; Sullivan, 1978; Mortimer, 1997; Garner and Hafez, 2000; Eddy, 2006). The endpiece is the region beyond the distal end of the fibrous sheath. This region contains only the terminal segment of the axoneme, surrounded only by the cell membrane of the sperm tail (Fawcett, 1975; Mortimer, 1997; Garner and Hafez, 2000). It has been well documented that a variety of sperm structural modifications are present in ejaculates of all species. The morphometric shapes and/or size of the sperm cells that are different from the normal structure that is characteristic of the individual species are referred to as abnormal or teratozoic spermatozoa. The distinct morphological deviations from normal structure can be usually identified during routine clinical evaluation of semen quality. The importance of morphologically abnormal spermatozoa to fertility was first brought to the attention of other investigators by Williams (1920). Williams and Savage (1925), and then Lagerlof (1934), reported the production of abnormal spermatozoa by infertile and sterile bulls. For more than 70 years, there have been extensive studies involving the identification of abnormal sperm morphology and its relationship with fertility (Lagerlof, 1934; Salisbury et al., 1942; Cupps and Briggs, 1965; Blom, 1972; Lorton et al., 1983; Barth and Oko, 1989; Söderquist et al., 1991; Foote et al., 1992; Howard et al., 1993; Saacke, 2008). Abnormal sperm cells have long been associated with sub-fertility or sterility, depending on the type or frequency of the morphological abnormalities. However, the prediction of fertilizing ability is still largely a mystery owing to the fact that abnormal sperm cells coexist along with normal sperm cells, and the presence (i.e. number) of normal cells can be adequate for normal fertility. Still, when a very high frequency of several morphological abnormalities is found in the ejaculate, fertility of the male is expected to decline (Salisbury et al., 1942; Barth and Oko, 1989). Thus, relatively arbitrary thresholds of the percentage of morphological abnormalities have been established by andrologists in the animal breeding industry in order to consider the ejaculate as worthy of use in animal breeding. The economic impact of sub-fertility is of the utmost importance, even in light of superior genetics. Therefore, periodic sperm morphological evaluations during either natural or artificial breeding programmes serve as a valuable tool for maintaining optimal fertility levels. There are various morphological schemes that are used by andrologists to characterize sperm abnormalities and the importance of each abnormality with respect to fertility (Salisbury et al., 1942; Blom, 1972; Barth and Oko, 1989; Söderquist et al., 1991; Saacke et al., 1994). These schemes include those described below. Earlier researchers classified morphological abnormalities as primary, secondary or tertiary according to their origin (Williams and Savage, 1925; Lagerlof, 1934; Salisbury et al., 1942; Blom, 1950; Garner and Hafez, 2000). The primary abnormalities were considered to be developmental abnormalities occurring within the seminiferous epithelium as a result of abnormal spermatogenesis before spermiation. These abnormalities include sperm head and acrosome abnormalities, midpiece abnormalities, e.g. the Dag defect, proximal cytoplasmic droplets and primordial cells. Secondary abnormalities were considered to be those originating after the sperm cells exit the testis. These abnormalities were attributed to altered epididymal maturation, prolonged retention of the sperm cells in the genital tract and abnormal composition of the seminal plasma introduced during ejaculation (Elmore, 1985; Roberts, 1986; Garner and Hafez, 2000). Secondary abnormalities include distal cytoplasmic droplets, simple bent tails, free normal heads and detached acrosomes. Tertiary abnormalities were characterized as being the result of improper semen handling during or after collection. Examples include fast cooling, high ambient temperature, contamination with urine or water and improper slide preparation for examination (Mitchell et al., 1978; Elmore, 1985; Roberts, 1986; Garner and Hafez, 2000). Although the classification of sperm abnormalities based on their origin has been widely accepted, it is often difficult to distinguish the anatomical origin of those abnormalities, even with the use of the electron microscope. For example, although the Dag defect was considered to be a primary defect, it also was reported to occur during the caput epididymal transit, although it was normal in the testis (Blom, 1972, 1973). Blom (1972) reported that some sperm head abnormalities were not related to improper testicular function, but occurred after the cells exited the testes. Due to the fact that the origin-based classification was observed to be not consistent, Blom (1972) suggested a new scheme in which a sperm abnormality is either associated with a major adverse impact on male fertility, i.e. ‘major defects’, or is associated with a minor effect on male fertility, i.e. ‘minor defects’. A high incidence of major defects is associated with impaired fertility or sterility and probably results from abnormal conditions in the testis or epididymis, or from hereditary genetic defects. The minor defects are considered to be less important for male fertility, unless they are present in a large percentage within the ejaculate. Sperm morphological abnormalities have more simply and traditionally been classified based on where the abnormalities are localized on the spermatozoa. Thus, the abnormalities are categorized as being of the acrosome, the head, the neck or midpiece, or the tail (Söderquist et al., 1991; Al-Makhzoomi et al., 2008). Spermatozoal abnormalities have also been classified as compensable or uncompensable based upon the ability to overcome the presence of the abnormalities and achieve optimal fertility by increasing number of spermatozoa per breeding dose (Saacke et al., 1994; den Daas et al., 1998; Saacke et al., 2000). It should also be noted that non-motile sperm and sperm with gross abnormal morphologies are prevented by the female reproductive tract from reaching the site of fertilization; so an insufficient number of spermatozoa may then be present to fertilize the oocyte, and decreased fertility is the result (Saacke et al., 1998). The incidence of compensable sperm defects can be overcome by increasing the number of normal spermatozoa in an artificial breeding dose. Therefore, the fertility of the bull will be at the maximum level for that individual. Increasing the number of normal spermatozoa further will not affect (i.e. increase) fertility. It should also be noted that different individuals exhibit different maximum fertility levels (Pace et al., 1981; Saacke et al., 1994; Saacke, 2008). Increasing the number of spermatozoa does not overcome the negative effects of uncompensable sperm defects, which may be of molecular or genetic origin. Uncompensable sperm defects are likely to be identified as normal or slightly irregular during routine laboratory evaluations. These sperm are often capable of reaching the site of fertilization, and penetrating and activating the oocyte, but fail to support zygotic and embryonic development (Saacke et al., 1998; Evenson, 1999; Saacke, 2008). Genetic sperm defects occur without environmental effects and at a constant rate; they can be transmittable to future generations (Chenoweth, 2005). The diagnosis of such defects is especially important as, otherwise, impaired fertility occurs in both current and future generations (Sullivan, 1978; see Chapter 7 (Chenoweth and McPherson) for a complete discussion of sperm genetic abnormalities). ROS are important modulators of sperm capacitation and function (Agarwal et al., 2005). At high concentrations, ROS can be detrimental to sperm viability; however, at low concentrations, they are important to cell signalling (de Lamirande et al., 1997; Griveau and Le Lannou, 1997; Rivlin et al., 2004; Sanocka and Kurpisz, 2004; Roy and Atreja, 2008). ROS include superoxide anions, hydrogen peroxide, nitric oxide and lipid peroxides. Intracellular protein peroxides are created through photo-oxidation of organic molecules by ultraviolet (UV) and visible light, creating singlet oxygen (Wright et al., 2002). O’Flaherty et al. (2006) demonstrated that ROS modulate phosphorylation events at multiple points in the phosphorylation pathways during the capacitation of human sperm. Tyrosine phosphorylation is one such major pathway (Naz and Rajesh, 2004), and this has also been demonstrated in boar (Flesch et al., 1999; Bravo et al., 2005) and equine sperm (Baumber et al., 2003). Physiologically, an increase in ROS has been shown to have a detrimental effect on sperm function. Gong et al. (2012) found that decreased levels of peroxiredoxin enzymes that scavenge free radicals are correlated with increased levels of DNA damage and with decreases in human sperm quality. Indeed, increased levels of ROS are commonly associated with human male infertility (Aitken et al., 2012). Wang and Liu (2012) found increased levels of 8-hydroxy-2’-deoxyguanosine (8-OHdG), a product of DNA oxidation, in sperm from human males clinically diagnosed with idiopathic asthenozoospermia. Increases in ROS production can also be related to observed sperm morphological alterations, such as seen in sperm from infertile men (Aziz et al., 2004), stallions (Ball et al., 2001) and dogs (Cassani et al., 2005). Such differences in ROS production are also commonly seen in sperm from males of the same species, either as an endogenous trait responding to environmental conditions, as demonstrated in Bos taurus and B. indicus when responding to seasonal changes (Nichi et al., 2006), or in response to other stressors such as advancing disease and pathology in the human male reproductive tract (Vicari et al., 2006). An increase in ROS in ejaculated sperm has been associated with increases in DNA damage in humans and rams (Fedder and Ellerman-Eriksen, 1995; Hughes et al., 1998; Twigg et al., 1998a,b; Wang et al., 2003; Said et al., 2005a,b; Kasimanickam et al., 2006). An important component of mitochondria is cytochrome C (cytC), which, in conjunction with Complex IV, participates in establishing the electrochemical gradient of mitochondria and the subsequent production of ATP. When the structural integrity of the mitochondrial inner membrane is compromised, cardiolipin (CL) is released and binds to cytC (Kagan et al., 2009). The resultant cytC/CL complex produces hydroperoxides (Kagan et al., 2006). Other critical proteins, such as apoptosis inducing factor (AIF), also emerge from the deteriorating mitochondrial membrane (Susin et al., 1999; Candé et al., 2002; Fleury et al., 2002; van Loo et al., 2002a,b; Philchenkov, 2004) and are present in sperm (Martin et al., 2004; Paasch et al., 2004; Grunewald et al., 2005a,b). Mature sperm are terminally differentiated cells that are also transcriptionally silent (Monesi, 1965), unlike somatic cells, which are transcriptionally active during apoptosis. The caspase proteins, which are part of the signalling pathways to programmed cell death, i.e. apotosis, are absent from mature sperm cells. Their presence in sperm then is indicative of immature or damaged cells, for example after the cryopreservation and thawing processes. The presence of activated apotosis signalling, which has been termed ‘apoptotic like’, is indicative of decreased fertilizing ability (Grunewald et al., 2008, 2009). Not all research groups have noted a clear correlation between increased ROS production and negative fertilization effects or morphological abnormalities (Moilanen et al., 1998). Zan-Bar et al. (2005) found that ram sperm were more susceptible to light-induced ROS generation than were tilapia sperm. It is also possible that the ability to discern differing levels of ROS production could be compromised, as ROS production has been shown to decline over time during the assay period (Kobayashi et al., 2001); this could potentially skew the results. Since ejaculates are comprised of millions to billions of individual sperm cells, variability in the overall quality of the ejaculate is reflected in the total number of individual defects. Sperm with defects in the nuclear chromatin or the protamine milieu have been demonstrated to cause problems to sustained embryo development (Ward et al., 2000; Seli et al., 2004; Lewis and Aitken, 2005; Nasr-Esfahani et al., 2005; Suganuma et al., 2005). Excess lipid peroxidation has also been shown to be correlated with midpiece abnormalities in human semen (Rao et al., 1989). However, mitochondrial abnormalities cannot be too severe or the resulting pathology will be reflected in the overall midpiece structure, thereby ablating or seriously attenuating the motility and subsequent fertilizing ability. The ability of sperm to progress though cervical mucus is seriously compromised in sperm with midpiece deformities, while sperm of normal morphology are favoured (Jeulin et al., 1985). It would therefore be logical to believe that midpiece deformities prevent sperm from reaching the site of fertilization. Because of the role of mitochondria in creating and regulating ROS during oxidative phosphorylation (Brookes et al., 2004), and because ROS may have a detrimental effect on sperm, significant disturbances in the functional physiology of the midpiece, which are not necessarily reflected in the morphology, are still of great concern. Major disruptions to any of the mechanisms regulating testicular temperature can be detrimental to spermatogenesis. In mammals, the spermatogenic apparatus of the testicles functions optimally at a temperature 2–4°C below that of the core body temperature. This cooler temperature range is maintained in most terrestrial mammals by a combination of sweating and evaporative cooling of the scrotal epidermis (Waites, 1991), by changes in testicular position relative to the body as adjusted by the tunica dartos muscle (Maloney and Mitchell, 1996) and by the countercurrent exchange system of the pampiniform plexus (Coulter and Kastelic, 1994). Waites and Voglmayr (1962) also provided evidence that in rams the apocrine sweat glands of the scrotal epidermis are stimulated by adrenergic sympathetic nerves in response to elevated temperature. Johnson et al. (1969) reported a decrease in the testicular weights of rams exposed to an increase in ambient air temperature. The weight changes corresponded to increased levels of lipids and free cholesterol, possibly due to decreased androgen production. Ambient heat is relevant to sperm quality issues not only because it can be reproduced for scientific study, but also because animals are indeed subjected to elevated temperature and humidity indices. Extremes of heat can compromise sperm quality, as evidenced by an increase in the number of abnormal sperm (Austin et al., 1961; Igboeli and Rakha, 1971; Kumi-Diaka et al., 1981; Parkinson, 1987; Mathevon et al., 1998). Spermatogenesis in animals occurs in a progressive, cyclical manner, known as the cycle of the seminiferous epithelium. The time required for these progressive changes is the duration of the cycle, and differs by species. When scrotal insulation is applied under research conditions, specific patterns of sperm cell abnormalities arise in relation to the temporal characteristics of spermatogenesis (Barth and Oko, 1989; Vogler et al., 1993; Karabinus et al., 1997; Zhu and Setchell, 2004; Enwall, 2009; Rahman et al., 2011). Cryptorchid animals are a ‘natural’ example of the effects of heat on spermatogenesis. The temperature of the cryptorchid testis reflects that of the body temperature. Bilaterally cryptorchid individuals are infertile (Setchell, 1998), but spermatogenesis in cryptorchid pigs (Frankenhuis and Wensing, 1979) and rats (Karpe et al., 1984) has been restored if the testicles are removed from the warmer body cavity and moved into the cooler scrotum. Warming the scrotum in a heated water bath (Sailer et al., 1997), heating the neck of the scrotum (Kastelic et al., 1996), or insulating the entire scrotum (Vogler et al., 1991, 1993; Karabinus et al., 1997; Brito et al., 2003) can also induce changes to normal spermatogenesis via heat stress. When using semen from rams that had been scrotally insulated for 16 h/day on 21 consecutive days, Mieusset et al. (1992) noted that, while pregnancy rates in ewes did not decrease, embryonic mortality was significantly increased. Spermatogenesis in the bovine bull occurs over a 65 day process whereby the spermatogonial stem cells undergo mitosis and meiosis, and subsequently physiological and morphological alterations, to produce mature sperm (Johnson et al., 1994). When dairy bulls were subjected to 2 or 3 wk long periods of elevated ambient air temperature (in excess of 37°C), sperm concentration, motility and total numbers were decreased and spermatogenesis was almost ablated (Casady et al., 1953). When scrotal insulation is applied for 48 h, specific patterns of sperm cell abnormalities arise in relation to the temporal characteristics of spermatogenesis (Vogler et al., 1993; Brito et al., 2003; Walters et al., 2005a; Enwall, 2009). Dutt and Hamm (1957) reported that in unshorn rams subjected to an ambient temperature of 32°C for 1 wk, spermatogenesis was negatively affected 5 wk later. In male rabbits exposed to an air temperature of 43°C for 1 h, an effect was seen on fertilization but not on sperm motility (El-Sheikh and Casida, 1955). In roosters, a decrease of sperm numbers in the ejaculates occurred after a 3 h heat stress event, while sperm number recovered within 2 wk following the stress (Boone and Huston, 1963). Heat stress to the scrotum not only alters the morphology of testicular spermatogenic cells (Kumi-Diaka et al., 1981; Malmgren and Larsson, 1989), but also affects the morphology of ejaculated sperm (Parkinson, 1987; Vogler et al., 1991, 1993; Barth and Bowman, 1994; Kastelic et al., 1996; Sailer et al., 1996; Enwall, 2009). The resulting altered sperm morphology has also been linked to decreased fertility (Skinner and Louw, 1966; Thundathil et al., 1998, 1999; Ostermeier et al., 2000; Jung et al., 2001; Karaca et al., 2002; Brito et al., 2003; Walters et al., 2005a,b, 2006; Enwall, 2009). This is not surprising given that alterations in the nuclear structure of sperm have been shown to correspond to decreased fertility with or without heat stress (Sailer et al.
1Alta Genetics, Inc. Watertown, Wisconsin, USA; 2University of Istanbul, Istanbul,
Turkey; 3New Tokyo Medical College, Kolonia, Pohnpei, Federated States of
Micronesia; 4Mississippi State University, Mississippi State, Mississippi, USA
Introduction
Normal Sperm Morphology
The head
The nucleus
The acrosome
The tail
The neck
The midpiece
The principal piece
The endpiece
Abnormal Sperm Morphology
Classification of Abnormal Sperm Morphology
The origin of the abnormalities
Impact on fertility: major and minor sperm abnormalities
Localization of abnormalities
Compensable and uncompensable sperm defects
Genetic sperm defects
Reactive Oxygen Species (ROS) and Abnormal Sperm Production
The Environment and Abnormal Sperm Production
Temperature
Stay updated, free articles. Join our Telegram channel
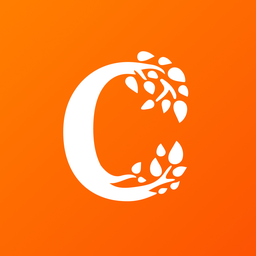
Full access? Get Clinical Tree
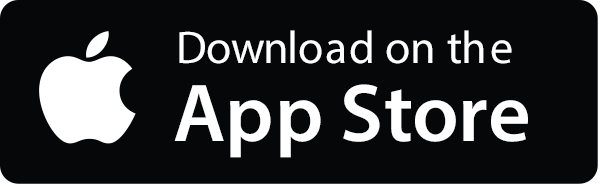
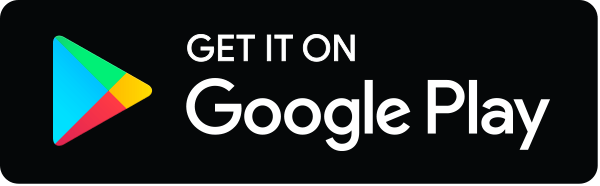