GENERAL OVERVIEW
The cell is the smallest unit of life, and all living matter (protoplasm) is composed of cells. Cells possess the unique abilities to replicate and to create energy from inanimate matter, which are qualities that characterize life itself. Many living creatures are unicellular (i.e., consist of a single cell), but higher organisms are comprised of a multiplicity of cells that specialize in different functions including absorption, digestion, and excretion.
In primitive prokaryotic cells, like bacteria, there is little organization of the many molecules that are needed for the life processes. In contrast, in eukaryotic organisms, the genetic material is organized in a nucleus (karyon) and many other processes have become restricted to membrane-bounded organelles. Such compartmentation prevents unordered mixing of different biochemical pathways, thus allowing for more sophisticated functions. The compartmentation also allows the cells to increase in size by ordered delivery of membrane-delimited molecules to their appropriate destinations. Thus, bacteria only reach 1 to 5 µm in size, while most eukaryotic cells are 5 to 50 µm.
Eukaryotic cell structure and function thus depend upon membranes and membrane-enclosed structures. The cell itself is delimited by the cell membrane. The cytoplasm consists of organelles, inclusions, and cytoskeletal components enclosed in a semiviscous liquid, the cytosol (Fig. 1-1). The study of the structural components of the cell is referred to as cytology, while the study of the integration of cells to form tissues and organs is referred to as histology. Such integration involves three different components: the cells themselves, the extracellular matrix (ECM), and tissue fluids. The ECM components are synthesized by cells and are present in different proportions in different tissues. Tissue fluids transport nutrients, hormones, gases, and waste products to and from the cells.
Many parts of the cell can be studied in the light microscope, which allows maximal resolution of structures down to 0.2 µm in diameter at magnifications up to 1000 to 1500 times. However, many cellular structures are smaller and the electron microscope, offering much better resolution (down to 0.1 nm) and higher magnifications (up to ×400,000), has to be used for their study.
FIGURE 1-1 Schematic drawing of the general organization of a cell.

In most cases, it is necessary to stain cells and tissues to increase their contrast in the light or electron microscope. A common light microscopy stain is a combination of the dyes, hematoxylin and eosin, which stain nuclei blue and cytoplasm pink, respectively. When negative molecular groups bind positively charged (basic) dyes (such as derivatives of hematoxylin), the stained structures are described as basophilic. In contrast, basic components (hemoglobin in mature red blood cells) attract positively charged (acidic) dyes such as eosin and are termed acidophilic. Electron-absorbing heavy metal ions are used as stains for electron microscopy. These stains show little chemical specificity, but impart contrast to cellular structures. In contrast, histochemistry allows the localization of specific molecules by use of antibodies tagged with labels that are detectable in the light or electron microscope (immunohistochemistry). Often, antibodies are labeled with fluorescent dyes that can be observed in special fluorescence microscopes. Enzyme histochemistry makes use of enzyme substrates that produce colored or electron-dense reaction products that precipitate at the site of enzyme activity. The study of living cells requires the use of special optical methods (phase contrast or interference contrast microscopy), which impart contrast to different cell components. Additionally, living cells can be stained with vital dyes that make it possible to follow fluorescent proteins expressed by genetically manipulated living cells.
CELL MEMBRANE
The cell membrane (plasmalemma) encloses the cell and forms its area of contact with the surrounding environment. The internal milieu of the cell must be kept constant, so the cell membrane is involved in the active and passive transport of ions, water, oxygen, carbon dioxide, nutrients, and secretory and excretory products. The cell membrane is also involved in anchoring the cell to surrounding structures, as well as participating in signaling and recognition events. This structure is sometimes referred to as a unit membrane, which follows the theory that all biologic membranes share the same structure.
The cell membrane is 8 to 10 nm thick and has a characteristic trilaminar structure in electron micrographs (Fig. 1-2). The trilaminar structure consists of an outer and inner electron-dense lamina separated by an electron-lucent layer. Biochemically, the cell membrane is composed of phospholipids, which have a hydrophilic head and hydrophobic tails. The phospholipids form a bilayer in the membrane with the hydrophobic tails facing each other. Thus, the hydrophilic heads of the outer layer face the outside of the cell, while the heads of the inner layer face the cytosol (Fig. 1-3). Such arrangement of the phospholipids has been correlated to the trilaminar structure with the polar heads and associated proteins making up the electron-dense laminae and the hydrophobic tails forming the electron-lucent layer. The bilayer also contains other lipids, such as cholesterol and carbohydratecontaining glycolipids.
FIGURE 1-2 Trilaminar plasma membrane (arrowhead) of a neurolemmocyte (×415,000).

Proteins associated with the cell membrane are either inserted into the phospholipid bilayer (integral proteins) or attached to its inner or outer surfaces (peripheral proteins). Transmembrane proteins are integral proteins that span the entire thickness of the lipid bilayer. A fluid mosaic is created by transmembrane proteins, which can diffuse laterally in the lipid bilayer. Protein diffusion is restricted by cytoskeletal components, cell junctions, and peculiar membrane substructures known as lipid rafts. Lipid rafts contain high concentrations of cholesterol and sphingolipids, which decrease the fluidity of the lipid bilayer. Some rafts contain proteins called caveolins, which reorganize the membrane into pear-shaped invaginations known as caveolae. These invaginations are believed to participate in signaling events and the cellular uptake of certain proteins such as albumin.
Some integral membrane proteins attach to oligosaccharides, forming glycoproteins, while others attach to larger polysaccharides (glycosaminoglycans), forming proteoglycans. Both oligosaccharides and glycosaminoglycans are present on the outside of the cell membrane. Together with the carbohydrate portion of the glycolipids, they form a carbohydrate-rich external cell coat, the glycocalyx, which is involved in many important functions relating to cell recognition, signaling, and mechanical protection. Blood group antigens form part of the glycocalyx of blood cells. The glycocalyx can be demonstrated by histochemical staining using the periodic-acid-Schiff method or with labeled lectins, which are proteins that bind to specific carbohydrate structures. The cell membrane is asymmetrical with respect to membrane proteins, the glycocalyx, and phospholipids.
Some transmembrane proteins are involved in cell-to-cell or cell-to-matrix interactions, while others form carriers or channels that transport substances such as ions and glucose through the membrane. Still other transmembrane proteins form receptors that transmit signals from outside the cell to inside. Certain hormones, including steroid hormones, can pass through the plasmalemma and bind directly to intracellular receptors. Most hormones, growth factors, and neurotransmitters are either too large or too hydrophilic to pass through the cell membrane, and therefore use transmembrane proteins as receptors. Binding to the extracellular region of such receptors evokes intracellular activation of so-called second messengers, such as cyclic adenosine monophosphate (cAMP). Second messengers are intracellular molecules that convey the extracellular message into the interior of the cell.
FIGURE 1-3 Schematic drawing of the organization of the cell membrane.

NUCLEUS
The shape, structure, and position of the nucleus vary considerably between cell types, and these characteristics are useful for cell identification. For example, lymphocytes have a round nucleus while granulocytes have a lobulated nucleus. The nucleus is centrally located in many cell types, but it is displaced to the periphery in others (e.g., fat cells and skeletal muscle cells). Most cells have a single nucleus, but some, like osteoclasts, possess multiple nuclei. Multinucleated cells may originate either from fusion of mononuclear cells or from incomplete division. Mature, mammalian red blood cells lack a nucleus altogether.
Nuclear Envelope
The nucleus is enclosed by a nuclear envelope, which contains nuclear pores that control the flow of materials between the nucleus and the cytoplasm. The nuclear envelope is comprised of two concentric unit membranes that are separated by a narrow (25 to 70 nm) space (Fig. 1-4). The outer membrane is often studded with ribosomes and may be continuous with the rough endoplasmic reticulum. On the inside of the inner membrane, the nuclear lamina is present. The nuclear lamina is a fibrous sheath, composed of specialized intermediate filaments called lamins that provide the nucleus with mechanical strength. At intervals, circular nuclear pores interrupt the nuclear envelope.
Materials pass through these pores in a manner that is strictly controlled by proteins of the nuclear pore complex. In this way, protein synthesis is restricted to the cytoplasm, permitting the nucleus to process and refine messenger ribonucleic acids (RNAs) without risking their translation into proteins during processing. This refinement is not possible in prokaryotes and provides eukaryotic organisms with possibilities for more complex exploitation of the genome (e.g., through alternative splicing of primary messenger RNAs).
Nucleoplasm
Chromatin refers to deoxyribonucleic acid (DNA) complexed with proteins, of which the basic histones form the quantitatively most important part and are involved in chromatin packing. Nonhistone proteins refer to proteins that are also involved in chromatin packing and protection or that participate in regulation of DNA duplication, transcription, and repair.
In specimens stained with hematoxylin or other basic dyes, the nucleus has areas of intense staining referred to as heterochromatin. Heterochromatin often occurs at the periphery of the nucleus attached to the nuclear lamina and consists of condensed DNA. Additionally, the nucleus contains lightly stained euchromatin that represents uncoiled DNA, which is accessible for transcription into RNA. Ultrastructurally, heterochromatin appears as electron-dense granular masses while euchromatin is electronlucent (Fig. 1-4). The proportion of heterochromatin to euchromatin varies between cells and is often an identifying feature.
Chromatin is packed by coiling at several levels of organization. The basic unit is the nucleosome that is formed from 200 base pairs of double-stranded DNA complexed with several histones. The exact number of base pairs involved is species variable. The nucleosomes are separated by shorter (about 50-base-pair) sequences of double-stranded DNA. Even during transcription, euchromatin is composed of nucleosomes and internucleosomal strands while transcriptionally inactive heterochromatin is further coiled. Heterochromatin is characterized by 30-nm chromatin fibers consisting of spirals of coiled nucleosomes. Even more elaborate coiling and structuring of chromatin occurs in conjunction with mitosis or meiosis and results in recognizable chromosomes.
FIGURE 1-4 Part of the nucleus (N), with electron-dense heterochromatin and electron-lucent euchromatin, and the cytoplasm (C) of a neurohypophysial glial cell. The nuclear envelope is pierced by pores (arrow). The perinuclear space between the inner and outer nuclear membranes is continuous with a cisterna of the endoplasmic reticulum (ER) (×67,000).

In all cells of female individuals, one of the two X chromosomes is permanently inactivated and does not participate in transcription. The inactive X chromosome is recognizable in epithelial cells from the oral cavity as a single granule called sex chromatin-(Barr body) that is attached to the nuclear lamina. In neutrophils, the sex chromatin takes the form of a drum stick-like appendage to the nucleus (Fig. 1-5). These structures have been used for gender testing in the past, but today, in situ hybridization techniques using labeled DNA probes that specifically bind to X chromosomes are preferred. The formula for genetic information encoded in the DNA is written using four letters representing the bases adenine (A), guanine (G), thymine (T), and cytosine (C). The sequence of these bases determines the information encoded in genes of the DNA, and this information is passed on (transcribed) to RNA. Hybridization refers to the unique sequence of bases of the applied probe that binds to the complementary sequence of nucleotides in the DNA being investigated (A binding to T and G to C). This technique affords a high degree of specificity and the probe can be labeled with a variety of substances that are detectable in either the light or electron microscope. Probes that are complementary to specific chromosome regions or genes can be used for prenatal diagnosis of hereditary diseases (Fig. 1-6).
The nuclear matrix refers to filamentous material remaining after enzymatic digestion and extraction of the nucleus. It is believed that the nuclear matrix is important for positioning chromosomes in the nucleus in a pattern resembling that observed during mitotic cell division.
FIGURE 1-5 Sex chromatin (arrow) in the nucleus of a neutrophil (×3800).

FIGURE 1-6 Bovine chromosome pair 7 identified by their intense (white) fluorescence using in situ hybridization on spread metaphase chromosomes (1), on cultured bovine endothelial cells, observed in fluorescence (2), and in interference contrast to bring out morphologic details (3). DNA has been gently counterstained by a more weakly fluorescent dye. Note in 2 and 3 the presence of a dividing cell (anaphase; left), in which the two pairs of chromosome 7 are being divided between the two daughter cells. Also note in 2 and 3 two interphase cells (right), which each contain two fluorescent dots corresponding to two copies of chromosome 7. By this approach, the number of chromosomes can easily be determined also in interphase cells, obviating lengthy preparation of metaphase chromosomes (×2000).

Nucleoli
The nucleoli are prominent, spherical structures involved in production of ribosomes (Fig. 1-7). At the light microscopic level, nucleoli range up to 1 µm in diameter and usually stain with basic dyes such as hematoxylin, depending on their RNA content. The number of nucleoli is determined by the number of active nucleolar organizing regions (NORs), which are chromosomal regions responsible for encoding ribosomal RNA (rRNA). Normally, there are fewer nucleoli than NORs because either some NORs are inactivated or several fuse to form one nucleolus. At the electron microscopic level, nucleoli have areas of varying electron density. A granular component representing maturing ribosomal subunits is often prominent. Fibrillar components representing NOR DNA and transcribed rRNA are also observed. Nucleoli, along with nuclear chromatin, are dispersed in a nuclear sap consisting of water, ions, free nucleotides, RNA, and protein.
FIGURE 1-7 Motor neuron from the spinal cord. The euchromatic nucleus contains a distinct nucleolus. The ergastoplasm (chromatophilic or Nissl substance) is visible as dark, pleomorphic masses throughout the cytoplasm. Cresyl violet Luxol fast blue (×1100).

CYTOSOL
The cytosol makes up about half of the cell volume and contains water, ions, sugars, amino acids, nucleotides, hundreds of soluble enzymes (e.g., of the glycolytic pathway), subunits for the cytoskeletal components, messenger RNA, transfer RNA, and myriad other molecules. Polymerization of actin is involved in regulating the viscosity of the cytosol. Transition of the cytosol from a gel state to a more soluble (gel-to-sol) state assists the formation of extensions (pseudopodia) involved in cell motility.
ORGANELLES
Rough and Smooth Endoplasmic Reticulum and Ribosomes
The endoplasmic reticulum (ER) forms an anastomosing (interconnected) network of membrane-delimited sacs (cisternae) and tubules. The rough ER (rER) is densely studded with ribosomes and is engaged in protein synthesis (Fig. 1-8), while the smooth ER (sER) lacks ribosomes and is engaged in lipid synthesis, calcium sequestration, steroid hormone synthesis, and detoxification of harmful agents. Both forms of ER are interconnected and the rER connects to the outer nuclear membrane. The ribosomes and membranes of the ER are below the resolution of the light microscope. However, the cytoplasm of cells that synthesize large amounts of proteins is rich in ribosomes and mRNA and is therefore strongly basophilic. Accumulations of basophilic, well-developed rER are sometimes referred to as ergastoplasm in pancreatic cells or Nissl substance in neurons (Fig. 1-7).
FIGURE 1-8 Electron micrograph of pancreatic zymogen cell, containing abundant cisternae of rough endoplasmic reticulum (rER) (arrows) and several secretory granules (arrowheads) (×6600).

The sER does not have attached ribosomes and is not engaged in protein synthesis. Instead, sER synthesizes lipids of cellular membranes and steroid hormones. Endocrine cells of the gonads and adrenal cortex have a well-developed system of tubular sER (Fig. 1-9). In addition, several drug-metabolizing enzymes that participate in detoxification of noxious and carcinogenic materials are present in sER. Finally, the sER performs calciumsequestering functions in many cells. Release of calcium from the sER can be elicited by several external stimuli and may precipitate a variety of cellular actions. Skeletal and cardiac muscle cells contain an elaborate system of sER, referred to as the sarcoplasmic reticulum, which sequesters calcium ions that are released upon stimulation of muscle contraction.
Ribosomes are bound to membranes of rER or present in the cytosol as free ribosomes. Each individual ribosome is formed from two spherical subunits of different sizes. The subunits contain several different proteins, all synthesized in the cytosol, and rRNA. A variety of cytosolic proteins enter the nucleus through the nuclear pores and are assembled with rRNA in the nucleolus. The immature ribosomal subunits then exit back to the cytoplasm through nuclear pores. Additional ribosomal proteins are added in the cytosol.
FIGURE 1-9 Adrenal cortical cell containing abundant smooth endoplasmic reticulum, mitochondria (M), and lipid droplets (L) in the vicinity of the nucleus (N) (×27,000).

Proteins are formed through the transcription of genes in the nucleus, which results in the formation of messenger ribonucleic acids (mRNAs). The mRNAs are exported to the cytosol, where they combine with the ribosomal subunits. An mRNA may be simultaneously translated by multiple ribosomes, thus forming a polyribosome (polysome). Multiple polyribosomes are often scattered in the cytoplasm of cells.
The nucleotide sequence of the mRNA depends upon the sequence of the gene from which it was transcribed and will, in turn, encode different proteins. All mRNAs attach to ribosomes by their initiator codon (AUG representing adenine, uracil, and guanine), which encodes methionine. For every amino acid encoded in the mRNA sequence, there exists a corresponding transfer RNA (tRNA) that transports an amino acid to the mRNA–ribosome complex. The first tRNA carries methionine to the complex. Subsequently, the ribosome moves along the mRNA to the next codon and recruits the next corresponding tRNA. The amino acids released from the tRNAs join together by peptide bonds and form a growing peptide chain that emerges from the mRNA–ribosome complex.
If a protein is predestined for lysosome incorporation, membrane insertion, or export from the cell, it will start with a short hydrophobic signal peptide sequence. The signal peptide binds to a signal recognition particle (SRP) present in the cytosol. The ensuing complex then attaches to an SRP receptor on the cytosolic face of the rER, which results in threading of the protein into the cisternae of the ER. Once inside the rER, the protein undergoes several posttranslational modifications including the cleavage of the signal peptide as well as folding and glycosylation. Cytosolic proteins that are destined to remain in the cell lack signal peptides and are synthesized by free ribosomes (not attached to the rER) in the cytosol.
Golgi Complex and Vesicular Transport
The Golgi complex consists of a series (usually 3 to 10) of flattened cisternae with a convex or cis side that is usually oriented toward the nucleus and a concave or trans side that generally faces the cell periphery (Fig. 1-10). At the trans side, anastomosing tubules and cisternae form the trans-Golgi network (TGN) (Fig. 1-11). In addition, small vesicles are present at the periphery of the cisternae and larger vesicles or vacuoles are often detected at the trans side (Fig. 1-10). Although the Golgi complex can be visualized by silver impregnation in light microscopic preparations, electron microscopy is necessary to delineate its structure.
Transmembrane, secretory, and lysosomal proteins are transferred from the rER to the Golgi complex. Small transport vesicles filled with newly synthesized proteins bud off the cisternae of the rER and fuse with the cisternae on the cis side of the Golgi complex (Fig. 1-11). Subsequently, the protein from the vesicles is transported through the Golgi complex in a cis-to-trans direction. During this transport, the protein becomes concentrated and undergoes posttranslational modifications, including glycosylation. At the trans side, appropriately modified proteins are sorted in the TGN and packaged in vesicles. Mannose-6-phosphatecontaining lysosomal enzymes bind to specific mannose-6-phosphate receptors in the TGN and become packaged in vesicles that will become primary lysosomes. When budding off from the TGN, these vesicles are coated with a protein, clathrin.
FIGURE 1-10 Electron micrograph of the Golgi complex (Go) and surrounding cytoplasm of an adenohypophysial cell. As the secretory granules mature, they move away from the concave (trans) side of the Golgi complex and their content becomes more electrondense. In the sequence of maturation of the secretory vesicles, the newest vesicle is I while the most mature vesicle is IV (×23,700).

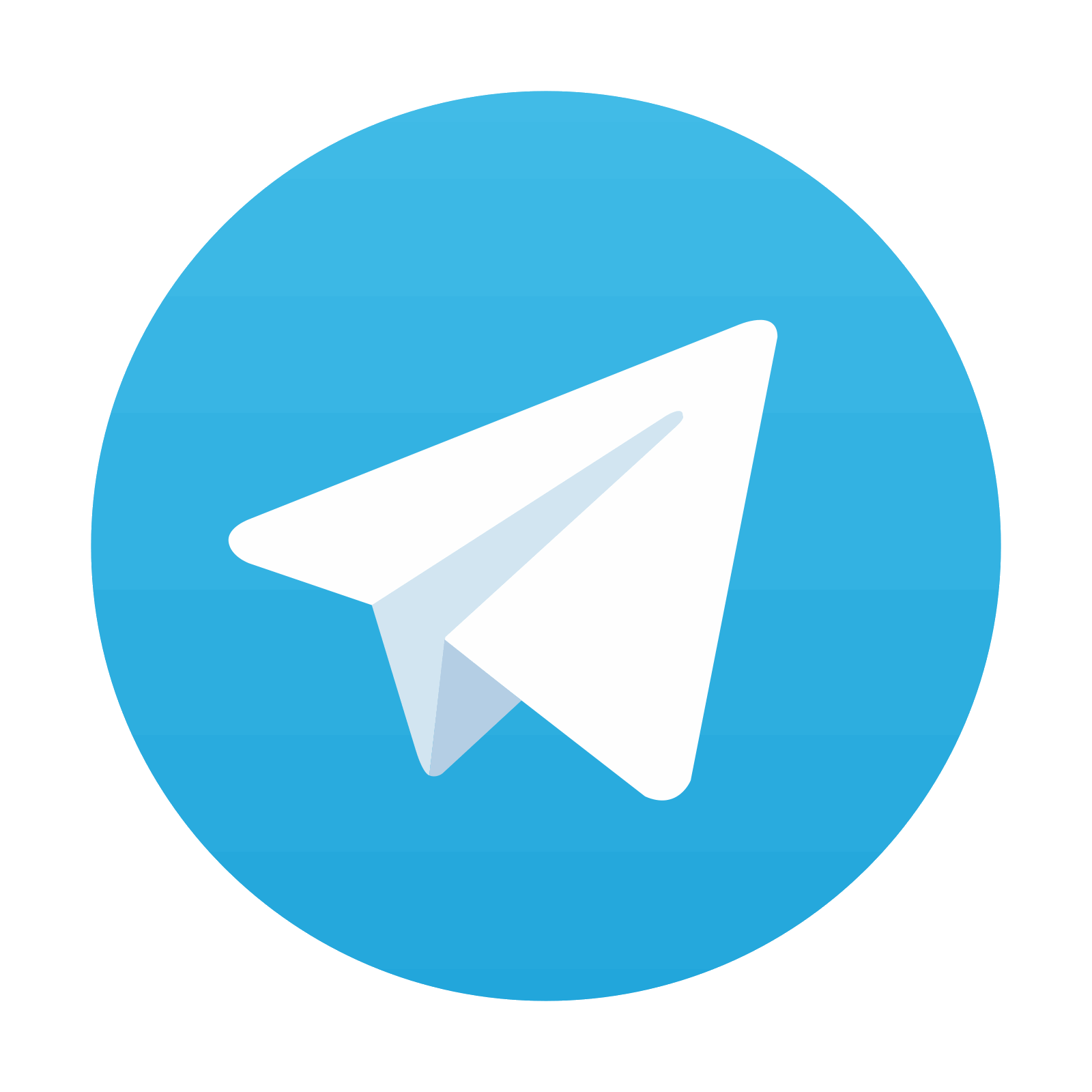
Stay updated, free articles. Join our Telegram channel
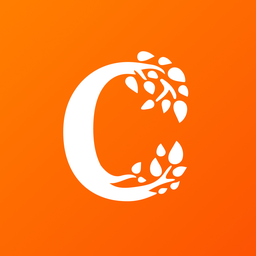
Full access? Get Clinical Tree
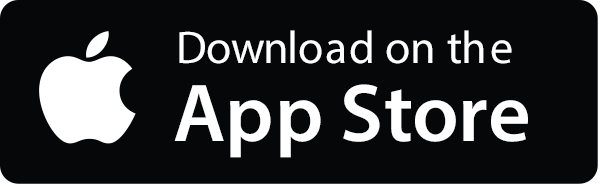
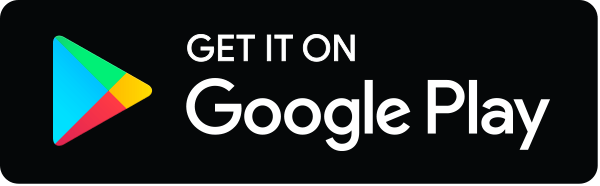