Sathya K. Chinnadurai1 and Cornelia I. Mosley2 1 Chicago Zoological Society/Brookfield Zoo, Brookfield, Illinois, USA 2 Elanco Animal Health, Mississauga, Ontario, Canada Reptiles, amphibians, and fishes are unique animal classes encountered in veterinary medicine and are very different in terms of anatomy, physiology, and behavioral adaptations from the more familiar mammals. Regardless of the differences among these animal classes, by using sound anesthetic principles reasonably safe and effective anesthesia can be performed, even in the absence of species‐specific information. In the following sections, emphasis has been placed on providing comparative anatomic and physiologic information relevant to the anesthetic management of each class of animals. A comprehensive review of the anesthetic and analgesic literature is beyond the scope of this text but is available elsewhere and should be consulted for additional detail [1–6]. Anesthetic management of reptiles is associated with many challenges. Reptilia represents a diverse class of animals in terms of size, environmental requirements, behavior, physiology, and anatomy. There are over 7800 species represented by four main orders (Crocodylia, Testudines, Squamata, and Rhynchocephalia). Compounding the difficulties created by these inherent species differences, historically there was relatively little peer‐reviewed research investigating anesthesia and analgesia in reptiles and much of the earlier literature is anecdotal in nature and persists as accepted fact despite contradictory evidence. Fortunately, there has been considerable interest in this area recently and scientific studies investigating anesthesia and analgesia in reptiles are becoming more available. Applying generalized anesthetic recommendations to such a large and varying group of animals is difficult. Clinical application of various techniques is often ascribed to one of the three principal orders: the crocodilians (crocodiles and alligators), the chelonians (tortoises and turtles), and the squamates (snakes and lizards). An understanding of normal physiology, pathophysiology, the action and disposition of anesthetic and related drugs, and a familiarity with the design and use of related anesthetic equipment are important considerations. A thorough preanesthetic assessment and carefully designed anesthetic plan with attention to premedication, induction, maintenance, monitoring, supportive care, recovery, and ongoing postoperative support and analgesia all contribute to the reduction of risk associated with anesthesia. Reptiles have long been considered to be the class of vertebrates that reflect the evolutionary transition between the aquatic and amphibious ectothermic vertebrates, and endothermic birds and mammals. Reptiles arguably represent one of the most ancient, successful, and well‐adapted classes of animals, with some species surviving essentially unchanged for hundreds of millions of years. Recent investigations recognize reptilian physiologic evolutionary adaptations as “optimal or advantageous,” enabling exothermic animals to inhabit almost all of the available non‐polar ecological niches. Although many aspects of reptilian physiology are similar to those of endothermic vertebrates, significant differences are present. Such differences may alter both the action and disposition of anesthetics and analgesics. The ectothermic nature of reptiles and their generally lower metabolic rates are probably the two most striking differences between mammals and reptiles that impede extrapolation of anesthetic principles and practices between the two groups. Reptiles derive much of their body heat from the surrounding environment and use behaviors such as basking or burrowing to regulate their body temperatures. Reptiles can also alter their body temperature through changes in their cardiovascular physiology. During periods of warming, some reptiles increase their heart rate and the degree of right‐to‐left cardiac shunting to increase the fraction of blood flow that is directed to the periphery for heating and ultimate return to the body core [7]. This adaptation facilitates more rapid and efficient warming of the animal. Reptiles will tend to choose a preferred optimal temperature zone (POTZ) maintaining a body temperature that is presumably ideal for the animal. In most instances, reptiles undergoing anesthesia should be maintained at the average or the high end of their POTZ to ensure optimal metabolic function. Species‐specific ranges can often be found in general husbandry references or extrapolated from known species, which are found in similar natural habitats. In general, 20–25 °C is recommended for most aquatic and temperate reptile species, and 25–35 °C for most tropical species [8]. Although ambient temperature is one of the main determinants of metabolic rate in resting reptiles, and consequently the metabolism and excretion of drugs [9], there are significant interspecies and intraindividual variations. Minimum and maximum oxygen consumption rates of individual reptilian species range from almost zero to values similar to a resting mammal [10]. The oxygen consumption of any individual is a function of the species, the temperature, and the individual. In general, the varanid and lacertid lizards tend to have relatively high metabolic rates, and boid snakes and chelonians have lower rates. Surface‐dwelling squamates have higher metabolic rates than burrowing species, and species of lizards that eat insects or other vertebrates have higher metabolic rates than herbivorous species [11]. Those species with higher inherent metabolic rates may be expected to more rapidly metabolize and excrete drugs compared to those with lower metabolic rates, but this has not been demonstrated conclusively. The non‐crocodilian reptile heart has three chambers: two completely separate atria and a single anatomically continuous ventricle. The crocodilian heart is more typical of that seen in mammals and birds with two completely divided atria and two ventricles. In the crocodilian heart, the foramen of Panizza allows for some intravascular shunting under circumstances of breath holding, such as diving. In non‐crocodilian reptiles, the ventricle is divided into two main chambers by a septum‐like structure called the “Muskelleiste” or “muscular ridge” (Fig. 57.1). This ridge originates from the ventral ventricular wall and runs from the ventricular apex to the base, dividing the ventricle into two anatomically defined but connected chambers: the cavum pulmonale and the cavum dorsale [12,13], comparable in function to the right and left ventricles of mammals, respectively. The cavum dorsale is sometimes further subdivided into the cavum venosum and cavum arteriosum. The dorsolateral border of the muscular ridge is free, permitting the flow of blood between the cavum pulmonale and cavum dorsale. However, during ventricular systole, the muscular ridge presses against the dorsal wall of the ventricle and separates the cavum pulmonale from the cavum dorsale; thus, in a functional sense, the heart is capable of acting as a two‐circuit pump. The pulmonary artery arises from the cavum pulmonale, and two aortic arches arise from the cavum venosum of the cavum dorsale. The left aortic arch becomes the dorsal aorta while the right aortic arch forms the subclavian and carotid arteries and a third branch joins the dorsal aorta. However, there is considerable anatomic variation among species. Figure 57.1 A generalized schematic demonstrating the anatomy and separation of blood flow in the non‐crocodilian reptile heart. The cavum venosum is the region of the heart where mixing of oxygenated and deoxygenated blood may occur. Deoxygenated blood returning to the heart via the right atrium passes through the cavum venosum to the cavum pulmonale. The volume of blood remaining in the cavum venosum after the muscular ridge contacts the dorsal wall of the heart (during systole), relative to the volume of oxygenated blood in the cavum arteriosum, will determine the magnitude of the shunted fraction of blood. The shunt fraction is ultimately controlled by pressure differences between the systemic and pulmonary circulations resulting in larger or smaller volumes of deoxygenated blood remaining in the cavum venosum during systole. Cardiac shunting occurs commonly in reptiles [14–16]. Cardiac shunts can occur in both directions, and under some circumstances may occur simultaneously in both directions [14,17,18]. The direction of the net shunt determines whether the systemic or pulmonary circulation receives the majority of the cardiac output. Intracardiac shunting has three important functions. First, shunting serves to stabilize the oxygen content of the blood during respiratory pauses. Second, the right‐to‐left shunt is partly responsible for facilitating heating by increasing systemic blood flow. Third, a right‐to‐left shunt directs blood away from the lungs during breath holding. During anesthesia, cardiac shunting can affect systemic arterial oxygen content and the uptake and elimination of inhaled anesthetics. The size and direction of the shunts are ultimately controlled by pressure differences between the pulmonary and systemic circulations and the washout of blood remaining in the cavum venosum (an anatomic subchamber of the cavum dorsale described in many reptiles) [14,16,18,19]. The pressure differences are principally controlled by cholinergic and adrenergic factors that regulate the vascular resistance of the pulmonary and systemic circulation [14,20–25]. Large right‐to‐left shunts limit the amount of anesthetic uptake early in the anesthetic period and slow anesthetic elimination at the end of anesthesia. Such shunts can delay the induction to and recovery from inhaled anesthesia. Changes in the level and direction of shunts may account for the unexpected awakening seen in some reptiles anesthetized with inhalant anesthetics. Intracardiac shunts also have implications for patient monitoring, in particular airway gas monitoring and pulse oximetry. Blood pressure in reptiles is controlled by mechanisms similar to those described in mammals [26]. The cardiovascular system of reptiles responds to both cholinergic and adrenergic stimulation in a manner similar to mammals and the presence of a baroreceptor reflex has been described [27]. The resting blood pressures of reptiles tend to be stable in the absence of external stimuli but may vary with temperature, activity, or state of arousal [28,29]. In contrast to mammals, systemic arterial blood pressures vary greatly among various reptilian species, making it difficult to identify a “normal” arterial blood pressure [26]. “Normal” blood pressure in reptiles may be more profoundly affected by environmental stresses such as habitat and temperature, species activity level, and size compared to the role of these factors in influencing blood pressure in mammals. This greater variability may originate from a reptile’s poor ability to regulate normal homeostasis independent of temperature and environment. Chelonians tend to have the lowest mean arterial pressures (15–30 mmHg) while some varanids have resting arterial pressures similar to mammals (60–80 mmHg) [30]. In the green iguana, normal resting mean arterial blood pressures are reported to be in the range of 40–50 mmHg while mean pulmonary arterial pressures are in the range of 15–30 mmHg [30]. The systemic blood pressures in snakes correspond to the gravitational stress they are likely to experience [31–33]. Snakes from arboreal habitats tend to have higher arterial pressures than those that are primarily aquatic. An allometric relationship between arterial blood pressure and body mass has also been described in snakes. As body mass increases, so does blood pressure. Several anesthetics, such as sevoflurane, isoflurane, halothane, propofol, tiletamine–zolazepam, and ketamine, have been shown to induce cardiopulmonary changes in reptiles similar to those seen in mammals [34–42]. The most significant difference between the respiratory physiology of reptiles, mammals, and birds is the lower oxygen consumption rate of reptiles. This difference reflects the lower reptilian metabolic rate. Both reptile respiratory anatomy and physiology vary markedly across species. The lungs of non‐crocodilian reptiles are suspended freely in the common pleuroperitoneal cavity and are not located in a closed pleural space. In reptiles, the lungs tend to be sac‐like with varying degrees of partitioning (Fig. 57.2). Highly aerobic species such as the varanids tend to have highly partitioned lungs with numerous septae and invaginations that increase the surface area for gas exchange. Chelonians and lizards tend to have paired lungs while most snakes have a single functional right lung. The functional units of the lung are referred to as “ediculi” and “faveoli,” which are structures analogous to mammalian alveoli. Most reptile lungs exhibit areas of both types of parenchyma. There is little detail regarding the trachea and extrapulmonary bronchial tree system in reptiles. The tracheal rings of chelonians tend to be complete, necessitating care when placing an endotracheal tube. In addition, the trachea may bifurcate quite proximally, so inadvertent endobronchial intubation may occur (Fig. 57.3). Many snakes also possess a tracheal lung, the significance of which is unclear. The lungs of reptiles tend to have a smaller respiratory surface area relative to lung volume. Figure 57.2 The lungs of many reptiles tend to be “sac‐like” with a comparatively low respiratory surface area relative to total lung volume. However, lung structure varies markedly among species with highly aerobic species having more partitions and invaginations to increase respiratory surface area. The image shows the dissection of the lung in a boid snake demonstrating the sac‐like structure. Figure 57.3 The trachea of many chelonians bifurcates quite proximally, increasing the risk of unilateral bronchial intubation. Because reptiles lack a diaphragm, they rely on the thoracic musculature for ventilation. Both inspiration and expiration are active processes so the respiratory depression associated with anesthesia may be more profound than that observed in mammalian species where expiration is a passive process. Because the muscles of ventilation include many of the same muscles used for locomotion, these two functions are relatively incompatible. Chelonians are faced with additional respiratory challenges since expansion of the thoracic cavity by movement of the ribs is not possible. The dorsal surface of the lungs is attached to the carapace and the ventral surface is attached to the abdominal viscera. Inspiration is accomplished by enlarging the visceral cavity, and expiration occurs by forcing the viscera up against the lungs, driving air out. This is accomplished by contraction of various posterior abdominal muscles and several pectoral girdle muscles. The control of respiration in reptiles is poorly understood. Both peripheral receptor and centrally mediated control have been proposed. It seems most likely that there is an interaction between a central system, which generates the pattern of respiration and afferent chemoreceptor input [43,44]. Both carbon dioxide partial pressure and pH appear important for stimulating normal ventilation but there is evidence that oxygen tension may play a role in normal ventilation, even under normoxic conditions [45]. Although there are some species variations, reptiles are generally viewed as episodic breathers [46–48]. Breathing occurs in respiratory “bursts” of activity followed by a pause of varying duration. Pulmonary vascular perfusion is also intermittent and changes in perfusion are in concert with respiratory rate and rhythm [22,49,50]. Ambient temperature has variable effects on the frequency, tidal volume, and minute ventilation [51] and due consideration should be given to maintaining the optimal temperature for a particular species. The response of reptiles to inspired carbon dioxide (CO2) is quite variable. Inspiration of greater than 4% CO2 in snakes and lizards produces an increase in tidal volume, a decrease in respiratory frequency, and an overall decrease in minute ventilation [52,53]. In turtles, specifically Pseudemys scripta and Chrysemys picta, the response to an increase in CO2 is an increase in minute ventilation as a result of increases in both respiratory frequency and tidal volume [26,54–56]. In turtles, breathing less than 21% but more than 10% oxygen produces little change in the respiratory pattern. At fractional inspired oxygen concentrations below 10%, some species increase ventilation while others retain their resting minute ventilation. Others may decrease ventilation [48,52,57–59]. In those species in which minute ventilation decreases or remains unchanged, metabolic oxygen consumption decreases. During anesthesia, most reptiles are maintained using an inhalant anesthetic delivered in nearly 100% oxygen. The delivery of a high oxygen concentration may further compound respiratory depression by blunting the contribution of oxygen for the stimulation of normal ventilation. In several reptile species, exposure to 100% oxygen significantly decreases minute ventilation [52,54,60–62], suggesting that high inspired oxygen may be responsible for at least some of the respiratory depression seen during anesthesia. The magnitude of this effect is likely small compared to the effects of anesthetics on central control of respiration and the muscles of respiration. However, there is some evidence that in the green iguana, recoveries from isoflurane anesthesia may be faster when the animal is breathing room air rather than 100% oxygen, possibly by improving ventilation and the subsequent removal of the inhalant from the body [63]. Interestingly, in studies using Dumeril’s monitors (Varanus dumerili) and bearded dragons (Pogona vitticeps), no significant differences in recovery times from either isoflurane or sevoflurane anesthesia were found between animals ventilated with room air or those ventilated with 100% oxygen [64]. A newer method described to shorten the time of anesthetic recovery from inhalant anesthesia is administration of intramuscular epinephrine. Epinephrine administered at 0.1 mg/kg IM was shown to significantly reduce the time from cessation of inhalant anesthesia to spontaneous ventilation and recovery in snapping turtles [65]. The authors of these studies proposed that the sympathomimetic effects of epinephrine may speed inhalant expulsion from the lungs, by reducing cardiopulmonary shunting. These findings may be relevant to other reptilian taxa. Reptiles cannot produce urine more concentrated than plasma, making the excretion of nitrogenous wastes more challenging for terrestrial reptiles. Most reptiles excrete nitrogenous waste as uric acid (uricotelic). Some turtles and crocodilians can also excrete urea. Uric acid is produced in the liver, and, unlike ammonia and urea, it is very insoluble in water and is excreted as a semi‐solid in dilute urine. In the reptilian kidney tubule, urine is very dilute so uric acid remains in solution. The cloaca forms a common conduit for products of the urogenital and gastrointestinal systems. It is often described as three distinct compartments but the actual degree of anatomic separation of these compartments varies by species. The most craniad portion is the coprodeum, which is a continuation of the terminal colon followed by the urodeum that receives connections from the urogenital system and urinary bladder (if present; not all reptiles have a urinary bladder, e.g., snakes) and the proctodeum, which is the most caudal section also commonly described as the vent. Dilute urine empties into the urodeum and then into the bladder or large intestine (via the coprodeum) where water is reabsorbed causing the uric acid to precipitate. This results in the excretion of nitrogenous waste with relatively little water. The bladder of some reptiles can be used for the storage of water. Reptilian urine is not a good indicator of renal function. Many reptiles also have specialized salt‐excreting glands that allow for the excretion of very high concentrations of sodium, potassium, and chloride. Many reptiles living in extremely arid locations can tolerate the marked fluctuations in total body water and plasma osmolarity that can occur in these environments. When faced with limited water supplies, plasma osmolarity can rise to levels higher than those known in any other vertebrate species. Many reptiles also have a renal portal system where blood from the hind legs and tail will pass through the kidneys before returning to the heart [66]. The effect of this system on the pharmacokinetics of drugs seems to be of little clinical significance in healthy animals. However, it is probably still best to avoid injection of nephrotoxic drugs or those that are likely to be significantly metabolized by the kidney into the hindlimbs and tail of reptiles [67]. The reptilian liver appears to be similar in structure and function to the liver of other vertebrates. Although there is little detail known about the reptilian liver, it is assumed that it probably plays important roles in tolerance to anaerobic metabolism, hypothermia, and adaptation to the physical environment. The liver of reptiles has a lower metabolic capacity compared to mammalian livers [68] and the metabolic rate is very sensitive to changes in temperature [69]. The lower metabolic rates for the reptilian liver probably account for at least some of the prolonged effects commonly seen with drugs such as antibiotics. The lower metabolic capacity of the liver may partly contribute to the prolonged anesthetic recoveries seen when using drugs that require extensive hepatic metabolism for termination of their clinical effect. Detailed information regarding clinical anesthesia in reptiles has been reviewed elsewhere and the reader should consult these references for specific information on anesthetic and analgesic techniques, drugs, dosage, and patient monitoring recommendations [1–6]. A few general concepts regarding reptile anesthesia will be presented below. Regardless of species or procedure, a preanesthetic assessment should be performed on all patients. Patient assessment should include a complete history, species identification, and physical examination. Any additional diagnostic tests such as blood work and imaging should be performed as indicated. Because most anesthetics produce some degree of cardiopulmonary depression, animals should be physiologically stable prior to the induction of anesthesia if possible. In some reptiles, the size, disposition, or anatomy may prevent the performance of a physical examination. In these animals, an assessment of body weight and general appearance may assist in determining the general health status of the animal. Species identification and information on their natural habitat may be useful. All animals should be kept at their preferred body temperature (PBT) throughout the anesthetic period and recovery. Performing anesthetic procedures early in the day allows animals predisposed to prolonged recoveries to recover during regular working hours rather than late into the night when support staff and patient supervision may be reduced. Intramuscular drug administration is most common in reptiles. Historically, hindlimb and tail sites have been avoided because of concerns related to the first‐pass effect associated with passage of administered drugs through the kidneys via the renal portal system during absorption. However, studies in some reptiles (turtles and green iguanas) suggest that this may be more of a theoretical than practical concern because only a small fraction of blood from the hindlimbs and tail passes through the kidney [66,67]. However, it is probably best to avoid hindlimb and tail administration of nephrotoxic drugs. The epaxial muscles provide a suitable injection site in most snakes. In lizards, the muscle mass of the forelimb (triceps and biceps), hindlimb (quadriceps, semimembranosus, and semitendinosus), and tail can be used. Caution should be used in species known to autotomize (drop) their tails (many geckos), because it is possible for an animal to “shed” their tail during handling. In chelonians, injections are most often administered in the triceps muscle. The cranial surface of the foreleg should be avoided since the proximity of the radial nerve to injection sites in this area increases the risk of damage to this nerve. The pectoral muscles can also be used, though in many chelonian species, there is a lack of significant muscle mass in this area. While intravenous drug administration is not always feasible in reptiles, the combination of good technique, practice, appropriate patient selection, and skilled physical restraint can facilitate predictable access to the ventral coccygeal vein in even very small snakes and lizards and the dorsal coccygeal vein in tortoises and freshwater turtles. In sea turtles, the dorsal cervical sinus has also been used for intravenous administration of drugs [70]. Intravascular injection decreases the latency of onset of action of an administered drug. It also decreases the variability in uptake and tissue irritation that is associated with intramuscular injections in reptiles. Techniques for catheterization of the coccygeal vein in both lizards and crocodilians have been described [71]. Intravenous catheterization of the coccygeal or abdominal veins is most often performed without direct visualization. In some species of turtles and tortoises, the jugular vein can be visualized but this technique often requires a skin incision and blunt dissection. Venous sinus sites are not ideal for intravenous catheter placement, but over‐the‐needle catheters can be used. A small gauge wire stylet through a needle (Seldinger technique) can be used to facilitate difficult catheterization. When required, cut‐down procedures should be performed in conjunction with a local or general anesthetic. Lidocaine diluted down to a 1% solution with sterile saline can be used for local infiltration. Although toxic doses have not been determined in reptiles, it is probably best to use less than 8–10 mg/kg. The most common sites for vascular access and associated technical tips are presented in Box 57.1. Intraosseous catheterization has been described in the green iguana (Iguana iguana), sea turtles, and desert tortoises [72–75]. Studies in green iguanas found similar renal uptake of the radioactive substance whether administered intraosseously or intravenously [72]. In desert tortoises, intraosseous administration via the humerus resulted in delivery to the systemic circulation (84%) that was similar to jugular administration, followed by the femur, plastocarpacial junction, and the gular region of the plastron [75]. This suggests that intraosseous drug administration is a suitable alternative to intravenous administration. To the authors’ knowledge, propofol is the only anesthetic drug that has been studied with intraosseous administration, but many other anesthetic and non‐anesthetic drugs have been administered successfully via this route. Intubation is easily accomplished in most reptiles because the glottis is in a relatively rostral position in the mouth at the base of the tongue. A small drop of lidocaine (diluted to 1%) can be used to desensitize the glottis and may facilitate tracheal intubation. In some aquatic reptiles, anatomic modifications of glottal folds may obscure direct visualization. The animal should be intubated with the largest diameter tube that can be placed easily. The mucus of reptiles tends to be very viscous and mucoid plugs can form in endotracheal tubes during longer procedures. It is important that this be recognized quickly as an inability of the lungs to fully deflate during expiration will occur. The trachea of some chelonians bifurcates quite rostrally, and endobronchial intubation is possible. The tracheal rings in chelonians and crocodiles are complete, and in most reptiles cuffed endotracheal tubes are avoided to prevent accidental overinflation and damage to the tracheal structures. Analgesic management of reptiles is challenging as a result of their unique physiologic, anatomic, and behavioral adaptations. Reptile pain and nociception have not been extensively studied, although there is compelling evidence that reptiles are capable of nociception. However, the sensory significance of nociception to the individual is poorly understood. Pain is normally defined as “an unpleasant sensory and emotional experience associated with, or resembling that associated with, actual or potential tissue damage” [76]. By this definition, pain is essentially an emotional experience. Arguably, it is impossible to describe this experience in reptiles given our current level of understanding and available technology. However, undoubtedly reptiles experience noxious insults as some sort of negative event and modify their behavior to escape such insults. A more robust and inclusive definition of pain may be more appropriate when describing pain in non‐human animals. For example, the following definition of pain would avoid the emotional aspects associated with the human definition and yet is suitably robust to encompass a range of experiences reasonably expected to result in what we might refer to as pain in a human: “a sensory experience representing awareness of damage or the potential for tissue damage that results in a behavioral and physiologic response to minimize/prevent the recurrence and promote healing of damage” (modified from Molony [77]). The experience of pain in non‐human animals may be similar to the experience in humans but it is important to consider that among classes and species of animals, pain may differ quantitatively and qualitatively. There are many gaps present in our understanding of reptile nociception, pain, consciousness, and suffering and although tempting, extrapolation from other vertebrate lineages should be undertaken cautiously. Reptiles have undergone millions of years of independent biologic evolution, separate from other vertebrate classes. While it is appealing to assume that all lineages of vertebrates have evolved similar survival strategies, it may also be somewhat presumptuous. It is true that the ability to sense noxious stimulus or potentially damaging stimuli is arguably necessary for the survival of all animals (invertebrates and vertebrates), but it is the significance of this sensation to the individual that remains less clear. Does the ability to perceive and suffer from pain, as defined in human terms, offer an evolutionary or biologic advantage to all animals or only some animals (i.e., humans)? Unfortunately, this is a complex and elusive question but one that nonetheless merits investigation. Regardless of an animal’s ability to experience pain and suffering as a negative emotional response, the aim should be to limit the biologic perturbations associated with the physiologic (nociceptive) processing accompanying a noxious stimulus. Various criteria have been used to evaluate the balance of evidence for the presence or absence of pain in animals. These criteria as they pertain to reptiles are briefly described below. The origination of the sensation associated with a noxious stimulus, transduction, occurs in specialized sensory nerve endings called “nociceptors.” Primarily nociceptive neurons (slowly adapting polymodal C fibers) have not been identified in reptiles. However, there is no evidence in the literature that they have been sought and not found but rather no one has looked specifically for these types of fibers. However, high‐threshold Aδ mechanical nociceptors [78] and C fiber mechanical nociceptors [79] have been identified in one species of snake. These fibers are nociceptors capable of transmitting nociceptive information. Spinothalamic projections analogous to the neospinothalamic (fast pain) and paleospinothalamic (slow pain) tracts have been identified [80] in reptiles and are presumed to be capable of transmitting nociceptive information from the body to the brain. Trigeminal tracts have also been identified [78,81] that may be capable of transmitting pain from the head to the brain. Unfortunately, there are no functional studies examining the role of these pathways in nociceptive processing. Reptiles appear to possess brain structures necessary for experiencing pain (neocortex) and morphologically there are direct spinal connections to the brainstem and dorsal thalamus in the midbrain. However, once again functional studies are lacking, and it is possible and even likely that brain processing of information may occur differently in different animals. For example, in some animals, smell and vision are highly developed and vast regions of the brain are dedicated to these activities, possibly producing sensations far beyond those a human could experience or even imagine. It has been suggested that differences in reptiles’ brain systems compared to birds and mammals may be ones of degrees of elaboration rather than presence or absence [82]. It is possible that the response to a noxious stimulus in reptiles is associated with less fidelity and scope compared to mammals. Perhaps a reptile’s “emotions” and the experience of pain are an “all or none” phenomena (rage or no rage, pain or no pain) rather than the graded experiences of human emotions (rage, anger, irritation, discontent, neutral, happiness, etc.). Certainly, humans describe a range of painful experiences from those that are simply a mild irritant to those that are intolerable. The opioid system has been well studied in reptiles and the presence of opioid receptors and endogenous opioids has been described [83–85]. Their exact role, receptor subtypes present, and their anatomic locations are somewhat less clear. Although opioids are important for modulating the neural processing of noxious stimuli, they also play roles in many other body systems, such as the reproductive, thermoregulatory, gastrointestinal, and circadian systems. In addition, several nociceptive‐related neuropeptides (i.e., glutamate, substance P, and calcitonin gene‐related peptide) have been identified in reptiles but their functions are not well elucidated. It is important to note that behavioral evidence does not always suggest awareness or conscious recognition of the nociceptive stimulus (i.e., pain perception). Many of the tests used to examine an animal’s response to a known or presumptive analgesic are actually measuring the effect the substance has on the animal’s nociceptive or nocifensive response. “Nocifensive” is a poorly defined term that is sometimes used to describe the unconscious withdrawal or movement away from a noxious stimulus. It is a response that can occur below the level of consciousness and hence differs from the response associated specifically with pain perception. Several experimental studies have examined the response of reptiles to substances known to be analgesic in mammals, primarily opioids. These studies have involved evaluating responses to various types of noxious stimuli, including thermal, mechanical, and chemical irritation. In general, these studies suggest that opioids reduce the response to some of these stimuli, although there is inconsistency among the different experimental nociceptive models used, the response of different reptile species to similar testing situations, and the drugs themselves.
57
Comparative Anesthesia and Analgesia – Reptiles, Amphibians, and Fishes
Introduction
Reptile anesthesia
Anatomy and physiology
Metabolism and thermoregulation
Cardiovascular system
Pulmonary system
Control of respiration
Effects of inspired carbon dioxide and oxygen
Renal system
Hepatic system
Clinical anesthesia
Patient presentation/assessment
Routes of drug administration
Endotracheal intubation
Pain, nociception, and analgesia
Evidence for pain in reptiles
Presence of nociceptors
Ascending transmission pathways
Brain structures
Nociceptive receptors and substances
Known analgesic actions on nociceptive or nocifensive behaviors
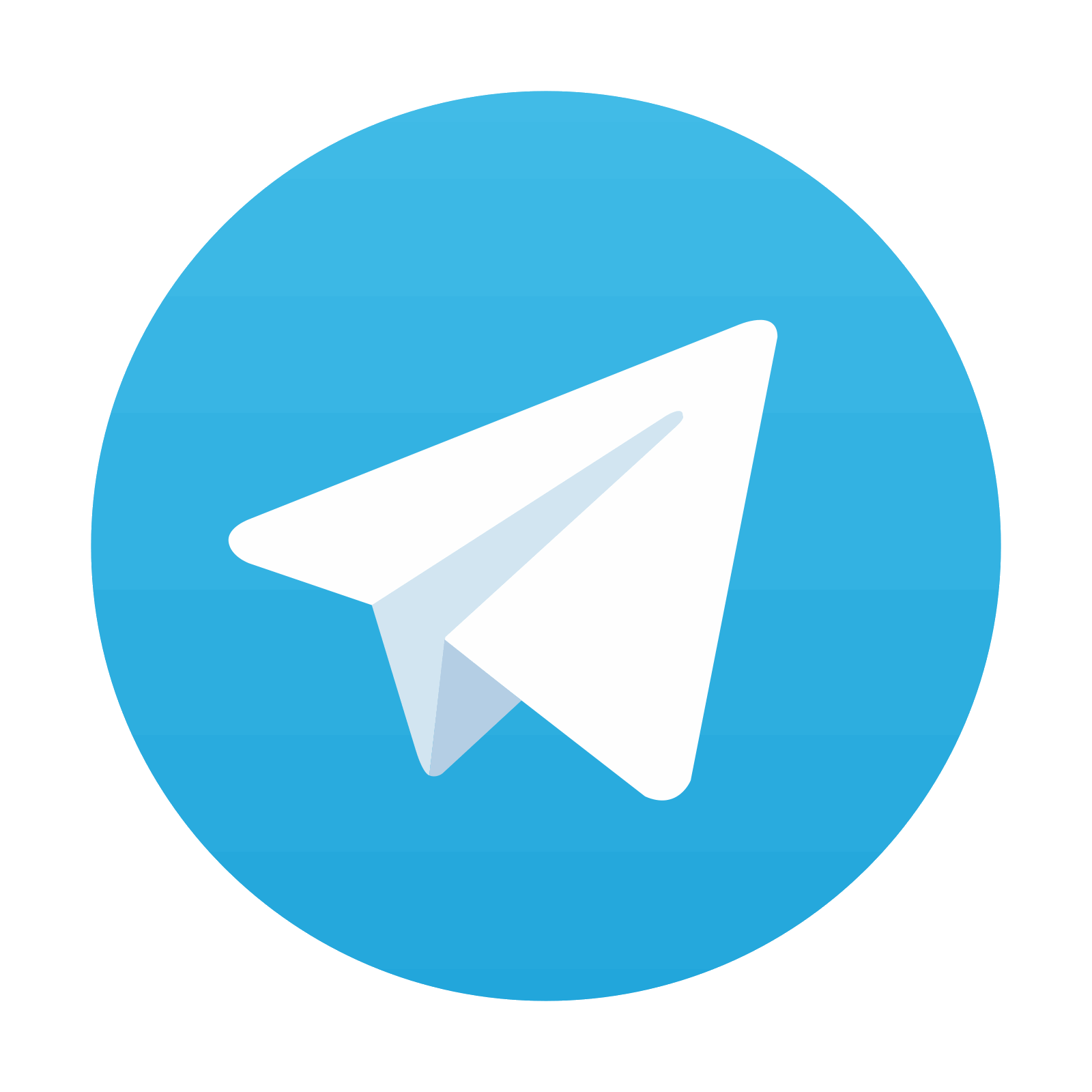
Stay updated, free articles. Join our Telegram channel
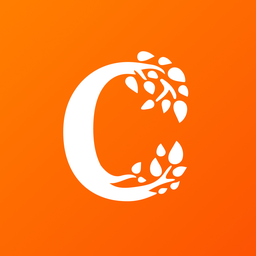
Full access? Get Clinical Tree
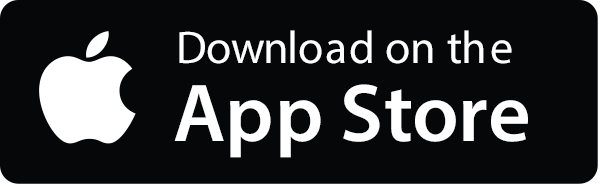
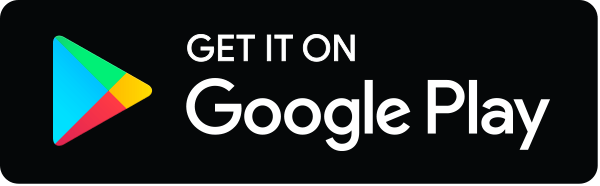