Julie A. Balko1 and James E. Bailey2 1 Department of Molecular Biomedical Sciences, College of Veterinary Medicine, North Carolina State University, Raleigh, North Carolina, USA 2 Innovative Veterinary Medicine, Inc., Ponte Vedra, Florida, USA Marine mammals are a diverse group of mammals that have evolved physically and physiologically to survive and thrive in varied marine environments, often at extremes of temperature, water depth, and pressure, and in limited daylight. Marine mammals are classified into four different taxonomic groups: cetaceans (whales, dolphins, and porpoises), pinnipeds (seals, sea lions, and walruses), sirenians (manatees and dugongs), and marine fissipeds (polar bears and sea otters). Anesthetic considerations for fissipeds (carnivorous mammals with separated toes) are discussed elsewhere. The ancestors of Cetacea developed on land, returning to the water 55 million years ago to evolve anatomically, physiologically, and behaviorally for a completely aquatic existence today [1]. Most clinical experience has been with the bottlenose dolphin (Tursiops truncatus, Tursiops truncatus gilli, and Tursiops truncatus aduncus), the most common cetacean managed in research, exhibit, and rehabilitation facilities. Other odontocete species have also been successfully managed in zoos and aquaria for many years, including killer whales (Orcinus orca), beluga or white whales (Delphinapterus leucas), Pacific white‐sided dolphins (Lagenorhynchus obliquidens), false killer whales (Pseudorca crassidens), rough‐toothed dolphins (Steno bredanensis), as well as several hybrid delphinoid species [2,3]. With increasing longevity in managed care, dolphins 50 years of age or older are not uncommon, with a median age of 32.0 years versus 19.9 years in free‐ranging counterparts, demonstrating the overall health of managed care populations [4,5]. Pinnipeds are aquatic, carnivorous marine mammals with streamlined torpedo‐shaped bodies, and limbs modified into flippers (pinniped meaning “feather” or “wing”‐footed). The living representatives of the order Pinnipedia include three families: Phocidae, the earless or “true” seals, Otariidae, the eared seals (fur seals and sea lions), and Odobenidae, the tusked seals (walruses). Pinnipeds are quite variable in body conformation with head‐to‐tail lengths ranging from 1.5 m to 6.5 m and body weights ranging from 35 to 3500 kg. Most clinical experience has been with the harbor seal (Phoca vitulina), the California sea lion (Zalophus californianus), and Pacific walrus (Odobenus rosmarus divergens), as these are the most common species in managed populations [6]. Fortunately, these species are not at conservation risk, and most pinnipeds in captivity are not captured from the wild, but rather the result of managed care breeding programs [7]. Many other pinnipeds species have been successfully managed in zoos and aquaria as part of conservation efforts, including gray seals, harp seals, Hawaiian monk seals, Stellar sea lions, and Atlantic walruses, to name a few. Unfortunately, anesthetic expertise is lacking for the many other species that will likely require conservation measures in the future. Sirenians, commonly called “sea cows,” are fully aquatic marine mammals comprised of three species of manatees (Trichechidae) and one species of dugong (Dugongidae), distributed throughout fresh, brackish, and marine tropical waters of North and South America, Africa, and Australia [8,9]. These hindgut fermenters, genetically related to elephants, are the sole obligate herbivores of the marine mammal world, although they have been observed to ingest invertebrates [10]. Sirenians are currently listed internationally as vulnerable or threatened, with some species considered endangered [9–13]. Habitat loss, natural disease, and human interaction mishaps (e.g., boat strikes) have resulted in this state of duress for the species and have, at times, necessitated advanced surgical and anesthetic intervention. Advancements in medical and surgical interventions for managed marine mammals have necessitated increasingly frequent application of sedation and general anesthesia in this cohort. While this has improved the longevity and well‐being of this mature and relatively closed population, it has also resulted in a growing amount of clinical anesthesia experience. As these techniques develop and translate to application in a field setting, free‐living marine mammals too will benefit. As the 1956 Nobel laureate in Medicine, Dr. Dickenson Richards, once said, “Breathing is truly a strange phenomenon of life, caught midway between the conscious and the unconscious, and peculiarly sensitive to both” [14]. Too often, anesthesia textbooks are dedicated to drug combinations and protocols rather than understanding the consequences of those choices and the management of expected (and unexpected) physiologic fallout. Of particular importance to marine mammal species is an understanding of the influence of drug choices on breathing and ventilation. Pinnipeds, cetaceans, and sirenians have an apneustic breathing pattern; the normal conscious breathing pattern of many marine mammals involves exhalation followed immediately by inhalation and a breath‐hold or apneustic interval. The rhythm for breathing is generated by a complex neural network in the brainstem involving both excitatory and inhibitory circuits. The medulla oblongata is the lower half of the brainstem and contains the dorsal and ventral respiratory groups. In vivo and in vitro experiments suggest a site in the rostral ventrolateral medulla, the preBötzinger Complex (preBötC), as the site of excitatory neurons both driving inspiration and generating the respiratory pattern [15,16]. Expiratory activity is regulated primarily by the Bötzinger complex (BötC), also located in the rostral ventrolateral medulla; in contrast, it contains mostly inhibitory neurons. The BötC has direct projections to and from the preBötC. The retrotrapezoid nucleus (RTN) of the central respiratory network, which has direct connection to the BötC, contains pH‐sensitive neurons. These neurons express the transcription factor paired‐like homeobox 2B (Phox2B); subjects with naturally occurring or induced mutations of Phox2B do not respond to CO2 or the pH change induced by CO2 [17]. As such, these Phox2B‐expressing neurons are fundamental to the generation and coordination of inspiration and expiration related to chemosensory control of breathing. Although the overall central respiratory network is far more complex than described here, it should be clear that perturbations of the function of this network severely disrupt breathing. Inhalation anesthetic agents alter the folding, localization, and aggregation of the Phox2B protein structure, thus affecting its ability to trigger breathing in response to rising blood CO2 concentrations [18,19]. Phox2B has been identified in the medulla of a wide variety of species [20,21], but it is theorized that the degree of expression, intrinsic functionality, degree of protein structural change, and localization when exposed to anesthetic agents may vary by species. As a result, this may alter each species’ CO2 responsiveness during equipotent anesthetic exposure. Inhalation anesthetic agents produce dose‐dependent depression of respiration, with a decrease in responsiveness to blood CO2 concentrations as the anesthetic concentration rises; this appears to relate to RTN responsiveness to changes in CO2 (or associated pH changes) [22]. This depression varies with species and clinically appears to follow the trend: human ~ canid < equid < otariid < phocid ~ cetacean [23]. The shift in the response to CO2 is such that, anecdotally, anesthetized otariids will not spontaneously ventilate until the arterial partial pressure of CO2 (PaCO2) is 60–80 mmHg. This appears even more pronounced in phocids, cetaceans, and sirenians, which are unlikely to spontaneously ventilate at equipotent levels of anesthetic exposure, even when the PaCO2 rises to the point of becoming anesthetic itself (PaCO2 ≥ 90–95 mmHg). Additionally, these species are likely to be apneic at light planes of anesthesia, where even gross purposeful movement may be evident. It has long been recognized that exogenously administered opioids are potent depressants of ventilation [24]. This is the result of a number of mechanisms, mainly activation of μ‐opioid receptors at multiple neuronal sites including BötC, preBötC, and other locations within the central respiratory network. The end result is decreased respiratory rate, tidal volume, and response to hypercapnia and hypoxemia, as well as loss of upper airway control (i.e., swallowing and prevention of aspiration); these effects are reversible with μ‐opioid receptor antagonists [25,26]. Interestingly, the respiratory depression of inhalation anesthetic agents is, at least in part, due to the influence of endogenous opioids [26]. As marine mammal species are anecdotally sensitive to opioids and prone to respiratory depression, further investigation of the endogenous opioid systems (β‐endorphins, enkephalins, and dynorphins) and their receptors (μ or MOR, δ or DOR, and κ or KOR) is warranted. γ‐Aminobutyric acid (GABA) is the most abundant inhibitory neurotransmitter in the mammalian central nervous system (CNS) with GABAA receptors distributed throughout cortical and subcortical areas. Considering its bounty, the receptor’s importance to memory, awareness, and consciousness becomes evident. Generally speaking, most modern anesthetic agents enhance the function of GABA and GABAA receptors which is important to anesthesia and emergence [27,28]. GABAA receptors are also present in the preBötC and stimulation can depress breathing, in part explaining the respiratory depressive effects of GABA agonist anesthetic drugs seen in some species [29]. Similar to terrestrial mammals, this effect appears to be both rate‐ and dose‐dependent in many marine mammal species, and overly rapid delivery of most modern anesthetic agents may cause apnea prior to sufficient relaxation of the jaw and upper airway, complicating intubation. Not all anesthetic agents exert their effect through action on GABA and GABAA receptors. While ketamine possesses some GABA effects, it primarily acts through the N‐methyl‐D‐aspartate (NMDA) glutamate receptors. Clinically, ketamine commonly alters the respiratory pattern, but infrequently interrupts spontaneous breathing at clinically relevant dosages. Ketamine has been successfully used to induce anesthesia of pinnipeds, mainly in free‐ranging settings. However, it has rarely been used in cetaceans and sirenians [30,31]; this is likely due to historical investigators’ needs to emulate sleep, as ketamine enhances cortical cholinergic tone and alters electroencephalographic (EEG) activity, interfering with sleep studies. As ketamine likely produces less respiratory depression than opioids in marine mammals and modulates pain through NMDA glutamate receptor inhibition, it remains a candidate for further investigation in marine mammals. To summarize, anesthetic agents depress the adequacy of spontaneous, involuntary ventilation in marine mammals, and even subanesthetic dosages may cause significant respiratory depression or apnea, especially in phocids, cetaceans, and sirenians. Biologists and veterinarians have long been fascinated by the interplay of sleep and breathing in fully aquatic mammals. Sleep might be simply defined as a state of immobility that is rapidly reversible and is best conducted in a place perceived as comfortable and safe. The superficial appearance of sleep and anesthesia led many investigators to equate drug‐induced unconsciousness with sleep; however, significant differences between the two states exist. Sleep involves the brainstem and frontal lobe function, while anesthetic drugs, such as propofol, have widespread or global influence on the brain [32]. In the 1960s, John Lilly observed that bottlenose dolphins slept with one eye open [33]. While he never produced any EEGs to support this hypothesis, he proposed from these observations what we now know as unihemispheric sleep. Unfortunately, he also misinterpreted barbiturate‐induced respiratory depression as the absence of involuntary breathing in cetaceans, leading to death [34]. Misinformed and misguided, many still reference Lilly believing that dolphins only breathe voluntarily, only undergo unihemispheric sleep, and must “wake” for every breath. Instead, the existing literature supports the conclusion that ventilation in marine mammals is automatic or involuntary, albeit sensitive to anesthetic drugs, and marine mammals are capable of bihemispheric sleep in the aquatic environment. Bihemispheric (both cerebral hemispheres) slow‐wave sleep is the traditional sleep pattern of most mammals in which both eyes are shut and both halves of the brain are simultaneously unconscious. In contrast, unihemispheric slow‐wave sleep is a sleep pattern in which one‐half of the brain is at rest while the other half remains vigilant. The concept of sleep, breathing, thermoregulation, and vigilance while in action was first described in birds “on the wing” [35] and has since been documented in marine mammals. In the 1970s, Serafetinides reported the first EEG evidence of unihemispheric sleep in cetaceans using subcutaneous needle electrodes in a pilot whale (Globicephala scammoni) [36]. Mukhametov used screw electrodes that allowed a degree of free movement around a small, shallow pool to record EEG readings of multiple, isolated dolphins over 24‐h periods [37,38]. These dolphins demonstrated that the vast majority of their slow waves were unihemispheric (with the contralateral eye held open), appearing first in one hemisphere and then the other. Remarkably, each hemisphere appeared to accumulate a separate sleep debt and when deprived of slow‐wave sleep for several days, only that hemisphere showed the typical sleep rebound; the non‐deprived hemisphere continued to alternate its slow‐wave sleep in the usual amount [39]. Using a small implantable telemetry unit, Ridgway replicated these earlier unihemispheric EEG findings and also recorded symmetrical EEG readings in both hemispheres during anesthesia and the recovery period after thiopental and halothane [40]. Short periods of EEG slow waves were identified days later in both hemispheres in the unanesthetized state. Pinnipeds can sleep on land and in water, with species differences in time spent sleeping in the water. Pinnipeds demonstrate a slower respiratory frequency when in water and when compared with terrestrial mammals of similar size [41]. Respiration during sleep, both in the water and on land, has been examined in a number of pinniped species including the gray seal, walrus, northern fur seal, southern sea lion, northern elephant seals, and Caspian seals [38,42–49]. The first variant of sleep in pinnipeds, and the predominant pattern for pinniped sleep studied on land, is bilaterally symmetrical slow‐wave sleep (BSWS) and rapid eye movement (REM) sleep, similar to that observed in terrestrial mammals [45,47]. The second pattern is characterized by a striking interhemispheric EEG asymmetry during slow‐wave sleep (SWS) and a very small amount of REM sleep. This pattern is recorded when fur seals sleep in water and is somewhat similar to cetacean unihemispheric slow‐wave sleep [36–40,50]. Sleep varies significantly between otariids and phocids; for example, respiratory pauses increase significantly during sleep of some phocids (e.g., ringed seal) [51]. Generally speaking, it has been theorized these differences relate to their respective ecological niches or range of living conditions. Although previous reports in the Antillean manatee (Trichechus manatus manatus) demonstrated bilateral δ waves with no unihemispheric sleep, a later study in a separate species, the Amazonian manatee (Trichechus inunguis) using EEG readings collected over a longer period of time (5 days) demonstrated a different pattern [52]. That is, unihemispheric slow waves occupied approximately one‐quarter of all waves, and bihemispheric slow waves characterized the majority of sleep. Sleep was characterized by short arousals for the act of respiration, followed by a rapid return to sleep during respiratory pauses. Overall, results demonstrated a circadian rhythm for sleep and wakefulness, and similar slow‐wave sleep to that evident in other aquatic species. In humans, home methods have been developed to study sleep as laboratory settings are uncomfortable and produce a barrier to accurate study [53]. Unfortunately, historic marine mammal laboratory sleep studies were often performed in wild‐caught subjects which were minimally acclimated, held in isolation in a very restricted space, had surgically implanted wires, and were frequently under the influence of barbiturates, benzodiazepines, or propofol to induce a “sleeplike” state. Even in these suboptimal conditions, marine mammal subjects clearly demonstrated bihemispheric and slow‐wave sleep for a portion of the time and none experienced respiratory arrest. In short, marine mammals are capable of both unihemispheric and bihemispheric sleep and appear to be involuntary breathers that can bring breathing under voluntary control as needed, similar to terrestrial mammals. Unihemispheric sleep affords them the benefits of sleep, breathing, thermoregulation, and vigilance while remaining in their aquatic environment. Unfortunately, there is limited evidence‐based data on the respiratory mechanics of anesthetized marine mammals. The elastic properties of excised lungs of marine mammals have been examined and the static inflation and deflation, pressure–volume curves have been measured. Inflation curves of excised dolphin and sea lion lungs were similar to dog lungs [54–56]. However, the deflation curves for the dolphin demonstrated greater recoil with smaller residual volumes compared with terrestrial mammals [54,57]. This may be due to the greater abundance of elastic fibers and muscle in the supportive tissue of the lung and conducting airways in the dolphin; however, possible biochemical differences must still be examined. Pulmonary morphology and mechanics of actively breathing dolphins have been reviewed [58], but data on the mechanics of ventilation for the unconscious and anesthetized dolphin remains unstudied. In general, there is a tendency for the lungs and chest wall of mammals to return to their original shape after being inflated mechanically, secondary to elastance. For air to flow into the lungs, the elastic resistance of the lungs and the chest wall must be overcome. This elastic impedance of the lungs and chest wall is additive. The inverse of elastance is compliance, or the ease with which the chest wall and lungs are distended. When the chest wall and lungs are very stiff and resist expansion (low compliance), there is a greater tendency for the lungs and thorax to collapse, and extra work is necessary to deliver a normal volume of air to the apneic patient when using a mechanical ventilator. In marine mammals, the extensive cartilaginous reinforcement of the airways from the trachea to the level of the alveolar sac (respiratory bronchioles are absent) should allow higher flow rates and faster gas exchange during the brief surface intervals of these animals [56]; however, this also contributes to elastic recoil and alters total compliance in the anesthetized, mechanically ventilated animal. This overall elastic recoil is so profound that it is the sole driving force for expiration in the surfacing pilot whale [59]. Others have recently noted that the chest wall of larger marine mammals appears to be much less compliant than terrestrial large animals [54,60]. Although studies are needed to confirm these observations of low total compliance, low compliance is particularly evident in anesthetized dolphins when out of water (beached). Specific respiratory mechanical data of unconscious marine mammals, in both beached and neutral buoyant states, is needed. It is theorized that total compliance will be significantly increased where large marine mammals, particularly cetaceans, can be maintained near‐neutral buoyant under anesthesia, such as applied in the past [61]. Investigations into methods of neutral buoyant (simulated microgravity) anesthesia are underway. Controlled mechanical ventilation (CMV) is the mechanical ventilation method available on nearly all veterinary anesthesia ventilators. It has often been treated as merely a convenience, taking the place of squeezing the anesthetic reservoir bag by hand to deliver a breath. However, new modes of ventilation, previously used only in intensive care units, are becoming available not only to mechanically ventilate the anesthetized patient, but to be used as therapeutic tools. The conventional CMV mode begins the ventilator cycle at a baseline (ambient) airway pressure and elevates it to deliver a resultant tidal volume (Fig. 56.1). Disadvantages of CMV include the need for application of relatively high peak inspiratory pressures (PIP) compared with other ventilation methods. This may lead to reduced venous return and cardiac output, and poor distribution of inspired gas flow with subsequent development of atelectasis and ventilation–perfusion mismatch. The addition of extrinsic positive end‐expiratory pressure (PEEP) to CMV in an attempt to reopen small airways and collapsed alveoli has been applied to large animal patients, but has proven minimally effective except at very high pressures that consequently significantly reduce cardiac output [62]. Early research in dolphins attempted to overcome the inadequacy of CMV for dolphin anesthesia by mimicking the normal respiratory pattern of dolphins with apneustic plateau ventilation (APV) [61,63–69]. With this mode, a prolonged inspiratory pause (apneustic plateau) is used and airway pressures then return to ambient pressure (0 cmH2O) at the end of expiration. Though a significant improvement in ventilation methodology, APV still failed to mitigate the development of significant atelectasis by allowing excessive time for lung deflation at ambient pressure [66]. Figure 56.1 A. Schematic idealized illustrations of observed pressure, flow, and volume versus time waveforms for controlled mechanical ventilation (CMV) and apneustic anesthesia ventilation (AAV). The gray background indicates periods of tidal ventilation corresponding to inspiratory time during CMV and the time (Tlow) at the low‐pressure setting (Plow) during AAV. The white background indicates expiratory time during CMV and the time (Thigh) of applied high pressure (Phigh) during AAV. CMV intermittently raises pressure from ambient to peak inspiratory pressure with most of the cycle time spent at ambient pressure. AAV intermittently releases pressure from Phigh to Plow to preserve lung volume. Importantly, the duration of each AAV release (Tlow) is limited to prevent expiratory flow stagnation (i.e., an expiratory flow of zero). Above, the first AAV release illustrates proper limitation of release time whereas the second illustrates excessively long release time with flow stagnation (arrow). B. Theoretical schematic illustration of dynamic pressure–volume curves (thin lines) for controlled mechanical ventilation (CMV) and apneustic anesthesia ventilation (AAV) superimposed on individual pressure–volume curves (bold lines) for the respiratory system. AAV was designed to preserve lung architecture and compliance in the anesthetized human by periodically releasing airway pressure from a high to low level while maintaining lung volume at or above functional residual capacity (FRC). By contrast, CMV ventilates the lung from below FRC leading to the development of atelectasis, shifting of the pressure–volume curve to the right, and lower compliance. In this scenario, higher pressures are required during CMV to achieve the same tidal volume. Source: Dr. James Bailey, Innovative Veterinary Medicine Inc., 2023; with permission. Unlike CMV, airway pressure release ventilation (APRV) employs an elevated baseline pressure followed by a drop in airway pressure to accomplish tidal ventilation. As first described in 1987, APRV involves continuous positive airway pressure (CPAP) with an intermittent pressure release phase [70,71]. More specifically, APRV is better described as applying continuous airway pressure (Phigh) identical to CPAP to maintain adequate lung volume and promote alveolar recruitment, with a time‐cycled release phase to a lower pressure (Plow) very transiently. Mechanically, this is not too dissimilar from the early APV mode used on dolphins, however, APV uses a Plow of 0 cmH2O and time sufficient for alveoli to collapse. APRV was developed to maintain functional residual capacity (FRC, the volume of gas remaining in the lung after a normal expiration) at a level that would optimize gas exchange and lung compliance in the spontaneously ventilating patient in respiratory failure. Unfortunately, when APRV is applied to the unconscious, non‐spontaneously ventilating patient (e.g., marine mammals), it allows lung volume to drop below FRC and may promote the development of atelectasis. Apneustic anesthesia ventilation (AAV) was developed to maintain lung volume at a level slightly greater than FRC. In this mode of ventilation, tidal ventilation occurs when airway pressure is transiently reduced from Phigh (CPAP) to Plow (the release pressure), allowing lung volume to decrease to no lower than FRC and supporting partial inflation of alveoli (Fig. 56.1). Immediate reapplication of Phigh leads to the alveoli remaining partially inflated (at a volume above FRC) prior to the lung volume increase. This intermittent pressure release and reapplication permits tidal breathing to occur without allowing development of atelectasis. Target Phigh and Plow are determined by optimizing compliance and the desired tidal volume. Note that mechanically, AAV and APV modes of ventilation are similar. However, the key distinction is that exhalation to lower airway pressures for a longer duration during APV can result in atelectasis, decreased compliance, derangement of ventilation and perfusion (V/Q mismatch), and hypoxemia. During AAV application, V/Q matching is maintained at baseline levels in anesthetized patients, and lung mechanics are normalized [72–74]. Of note, published studies in horses indicate AAV should not be applied in a static mode and is best used clinically as a dynamic mode of ventilation involving regular adjustments [74]. That said, it might better be described as a continuum of recruitment maneuvers. When applied in this dynamic mode, the advantages of AAV over other modes of ventilation include lower mean intrathoracic (pleural) pressure, augmented venous return, improved cardiovascular performance, and better distribution of inspired gas flow resulting in improved ventilation–perfusion matching [75]. The AAV mode is specifically indicated in patients with low lung and thoracic compliance, as may be the case in large marine mammal species, particularly the “beached,” anesthetized dolphin. APV, APRV, and AAV will each mimic the breathing patterns of conscious, actively breathing marine mammals, and live subject testing is needed to elucidate their role in the target marine mammal population. Of these modes, AAV would be anticipated to provide superior ventilatory support to the anesthetized, non‐spontaneously ventilating marine mammal and is actively under evaluation in California sea lions and bottlenose dolphins [76]. Additional testing of these modes of ventilation, including the evaluation of lower inspired oxygen concentrations, is currently underway and is producing promising results in both terrestrial and marine mammal species [68,74,76,77]. In theory, “lung protective” ventilation strategies that utilize lower tidal volumes may limit alveolar overdistension and minimize lung injury; however, they will concurrently lead to hypercapnia, commonly referred to as “permissive hypercapnia” in medical literature [78]. Fundamentally, when properly performed, AAV will produce a degree of intentional hypercapnia. Both intentional hypercapnia (whereby hypercapnia is intentionally implemented as part of the ventilation mode such as in AAV) and permissive hypercapnia (whereby hypercapnia is accepted in avoidance of potentially negative ventilatory effects) are generally well tolerated and are described in greater detail elsewhere in this textbook. Allowing a degree of intentional or permissive hypercapnia will allow implementation of lung protective ventilatory strategies and can both increase cardiac output and promote offloading of oxygen to tissues if held to a reasonable level. Although one may rationalize a degree of benefit of hypercapnia, recent work has suggested that high levels of CO2 are not beneficial to pinnipeds and indicate a need for enhanced monitoring and support [79]. If intentional or permissive hypercapnia is used, the following parameters are suggested limits: PaCO2 < 80 mmHg; pH > 7.20; and PaO2 > 80 mmHg. The authors apply both intentional hypercapnia and eucapnia, situationally dependent. Since hypercapnia increases cardiac output and blood pressure, individuals accustomed to use of permissive hypercapnia who achieve eucapnia, as a choice or out of necessity, may need to be prepared to manage hypotension they may not routinely observe. Additionally, hypercapnia may increase pulmonary vascular resistance of the ventilated lung, which can result in increased shunt fraction. Interestingly, sea lions appear to undergo hypoxic pulmonary vasodilation rather than vasoconstriction, perhaps increasing shunt fraction in improperly ventilated marine mammal species under anesthesia [80]. The first step in any sedation or anesthetic event is determination of whether chemical restraint is even necessary. Many minimally invasive procedures (e.g., blood sampling and diagnostic imaging) can be completed under behavioral control alone and addition of simple local anesthetic blocks may even facilitate more invasive procedures (e.g., tissue biopsy). If needed, this can be combined with mild sedation for short, minimally invasive procedures. Immobilization or general anesthesia will be warranted for longer or more invasive procedures (e.g., surgery), in free‐ranging species or those without behavioral control, or with large, potentially hazardous marine mammal subjects. While true anesthetic risk is still largely unknown for the vast majority of marine mammal species, it is likely higher than traditional domestic species due to a number of factors, including unknown health status, extrapolated pharmacology, and challenges with monitoring; a single retrospective study reported a perianesthetic mortality rate of 4.3% for free‐ranging pinnipeds undergoing sedation or anesthesia at a renowned rescue and rehabilitation facility [81]. In managed populations where a voluntary preanesthetic health assessment is feasible, examination and laboratory tests can provide an overview of the animal’s current health status and identify concurrent disease states. This is especially relevant when considering that many anesthetic procedures in managed populations are performed on geriatric patients. Where possible, this should include, at minimum, a physical examination, complete blood count and serum chemistry panel with a urinalysis, and imaging (e.g., radiographs, abdominal ultrasound, and echocardiography) performed where appropriate. Trainers must be vigilant to maintain necessary medical behaviors, but the anesthetist must recognize how disease (e.g., decreased vision), pain, and medications (e.g., preoperative opioids) can alter or lead to loss of medical behaviors. In situations in which a preanesthetic health assessment is limited (e.g., behaviorally non‐compliant patients, and free‐ranging subjects), visual inspection from a distance and interview of the managing trainers and veterinarians where possible should be performed. A limited preanesthetic assessment could put subjects at greater risk for negative outcomes and the anesthetist should prepare accordingly. When tailoring an anesthetic protocol, the anesthetist must consider the species, size, age, demeanor, training, as well as the working environment. Anesthesia of marine mammals requires a balance of behavioral, mechanical (physical), and chemical restraint. Though often useful, excessive reliance on mechanical restraint may lead to physical injury to the patient (e.g., improper desensitization, “off behaviors” due to illness), hyperthermia, or exertional myopathic conditions [82]. Similarly, even though darting an animal with ultrapotent anesthetic agents to cause immobilization can be efficacious, it may result in severe negative physiologic effects. As such, emphasis should be placed on preprocedural behavioral training for voluntary medical procedures whenever time and resources exist, as these may reduce the need for mechanical or aggressive chemical restraint. When mechanical restraint is needed, desensitization training to mechanical restraint devices, such as netting, slings, and squeeze cages is recommended. With proper desensitization training and chemical sedation, mechanical devices may be used to improve the safety of anesthetic procedures for both the patient and personnel, without undue added risk. The choices of anesthetic and perianesthetic drugs applied to marine mammal species have actually been rather limited, yet have been reviewed many times over the years, with focus primarily on immobilization cocktails and field applications of anesthetic drugs [83–90]. Induction of anesthesia of marine mammals requires a balance of behavioral, mechanical, and chemical restraint (Fig. 56.2). For instance, the process of anesthesia for pinnipeds has ranged from heavy mechanical restraint by lasso of juvenile Stellar sea lions followed by dowel and board squeeze/entrapment and mask induction with inhalation anesthetic alone [91,92], to solely chemical restraint by various potent and efficacious combinations of anesthetic agents delivered by dart, to remarkable behavior control by training for mask induction with an inhalation anesthetic agent alone under trainer command. In any situation, the concept of balanced anesthesia (use of a combination of drugs and techniques at lower individual doses to minimize dose‐dependent side effects) should be employed. Additionally, for marine mammals, a balanced approach to anesthesia usually includes considerations beyond anesthetic drug selection. For example, with sufficient personnel, it is feasible to manually restrain small cetaceans and sirenians, but pinnipeds of size will require additional tools for restraint. Figure 56.2 Anesthesia of marine mammal species requires a balance of behaviors, mechanical restraint, and chemical restraint. Behaviors are useful responses taught to marine mammals by qualified trainers. Although free‐living subjects may have natural behaviors that can be leveraged for capture, behavioral control will be poor. Mechanical restraint is the physical control of the subject by devices such as nets, slings, husbandry boxes, or squeeze cages. Chemical restraint is the application of pharmacologic agents (drugs) to bring the subject to a manageable state by producing tranquilization, sedation, neuroleptanalgesia, immobilization, or anesthesia. Sedation is not immobilization; however, immobilization is anesthesia. Immobilization cocktails should be considered higher risk. Risk increases as we move from behavioral control to chemical immobilization, particularly delivered by remote means leading to lack of proximity to the subject and delayed support. Source: Dr. James Bailey, Innovative Veterinary Medicine Inc., 2023; with permission. The rationale for the anesthetic protocol begins with assessment of behaviors. Behaviors are useful responses taught to marine mammals by qualified trainers. Desensitization training and voluntary medical behaviors contribute in no small part to more positive outcomes in managed populations, eliminating the need for aggressive restraint methods or potent cocktails with severe physiologic consequences. Skilled trainers and animal handlers are necessary to support safe anesthesia, and they must be given both the opportunity and time required for training where possible. Examples of useful medical behaviors are holding on target for injections by hand, tolerance of mechanical restraint in a squeeze cage, self‐beaching of dolphins, or acceptance of an anesthetic mask for inhalation anesthetic delivery. Even in subjects that could be very difficult to restrain, such behaviors can be sufficiently robust to allow free‐standing application of anesthetic agents without physical restraint. Without medical behaviors, there is greater reliance on both mechanical restraint, which can become dangerous and lead to injury, and chemical restraint, which often needs to be more profound. With chemical restraint, ut est rerum omnium magister usus or, experience is the best teacher [93]. Anesthesia methods applied to free‐living subjects are highly variable and often depend on the experience of the personnel, as well as the historical success of the method applied. For example, though less commonly used in managed species, the combination of tiletamine and zolazepam (Telazol® or Zoletil®) is still the preferred immobilization method for free‐living elephant seals. As most free‐living subjects are anesthetized to facilitate study of the species as a whole, these subjects are usually healthy individuals that may better tolerate the physiological challenges of a broader range of necessary mechanical and chemical restraint methods. On the contrary, rescued free‐living subjects are often debilitated or otherwise unhealthy and are at greater risk of negative outcomes in the face of immobilization methods necessary for the safety of human caretakers. The authors recognize the broad range of sedation and anesthesia methods applied to the wide variety of marine mammal species over the past many decades, and offer only to provide some general, repeatable methods in hopes of improved outcomes for all the species under our care, particularly the growing geriatric populations. Historically, drugs used in the anesthetic and perianesthetic period in marine mammals have been quite limited. These have been extensively reviewed in other texts and primarily focus on immobilization cocktails and field applications of anesthetic drugs [83–86]. To tabulate that historical information again here would not serve the anesthetist well and, instead, a brief summary of common drug choices for each species is provided below. As noted previously, anesthesia of marine mammals requires a balance of behavioral, physical/mechanical, and chemical restraint, although various combinations of the above have been used. Managed populations do not generally require aggressive restraint methods or potent cocktails for induction, especially if preprocedural behavioral training is implemented. When selecting an anesthetic protocol, the risk‐benefit ratio for each drug and technique should be carefully considered and the anesthetist should be reminded that, “There are no safe anesthetic agents; there are no safe anesthetic procedures; there are only safe anesthetists” (Robert Smith) [94,95]. The importance of vigilant perianesthetic monitoring and support in conjunction with any anesthetic protocol cannot be overstated and will be discussed further. While specific drugs will not be discussed in detail, α2‐adrenergic receptor agonist use in pinnipeds warrants discussion. One of the most commonly administered drug combinations in this cohort involves various combinations of midazolam, butorphanol, and medetomidine, and has proven effective in field immobilization of young, healthy otariids for short procedures [96]. While useful in certain situations, the authors have anecdotally appreciated a higher incidence of negative outcomes in some managed populations with use of an α2‐adrenergic receptor agonist. Medetomidine was used in > 50% of induction protocols reported in a retrospective assessment of anesthetic mortality in a cohort of rescued, untrained, free‐living otariids of variable disease condition and severity; perianesthetic mortality in that study was 4.3% [81]. Additionally, a high negative outcome rate (e.g., ciliary body hemorrhage, hyphema, and death) was anecdotally reported in pinnipeds following medetomidine or dexmedetomidine administration for ophthalmologic procedures. In a series of 13 prolonged ophthalmologic procedures on geriatric California sea lions (median age 22.5 years) immobilized with a combination of midazolam, butorphanol, and dexmedetomidine, one subject vomited, two had arrhythmias, two had prolonged recoveries, and three died during anesthesia or within 72 h of recovery [86,97]. For these reasons, α2‐adrenergic receptor agonists, despite their reversibility, may not be a reasonable choice in geriatric marine mammals or those undergoing lengthy procedures, and would be ill‐advised in phocids and odobenids [98]. This is not to say the α2‐adrenergic receptor agonists are not effective or on occasion necessary; however, the anesthetist must recall Maslow’s Axiom that “it is tempting, if the only tool you have is a hammer, to treat everything as if it were a nail” [99]. While it is easy, and sometimes necessary, to dart an animal with potent efficacious anesthetic agents (including α2‐adrenergic receptor agonists), all options should be explored and the physiologic consequences for this convenience should be considered. That being said, more judicious use of α2‐adrenergic receptor agonists may yet prove beneficial.
56
Comparative Anesthesia and Analgesia – Marine Mammals
Introduction
Breathing, sleep, and ventilation
Breathing
Sleep
Ventilation
Permissive or intentional hypercapnia
Preanesthetic assessment and planning
Protocol selection
Sedation and anesthesia
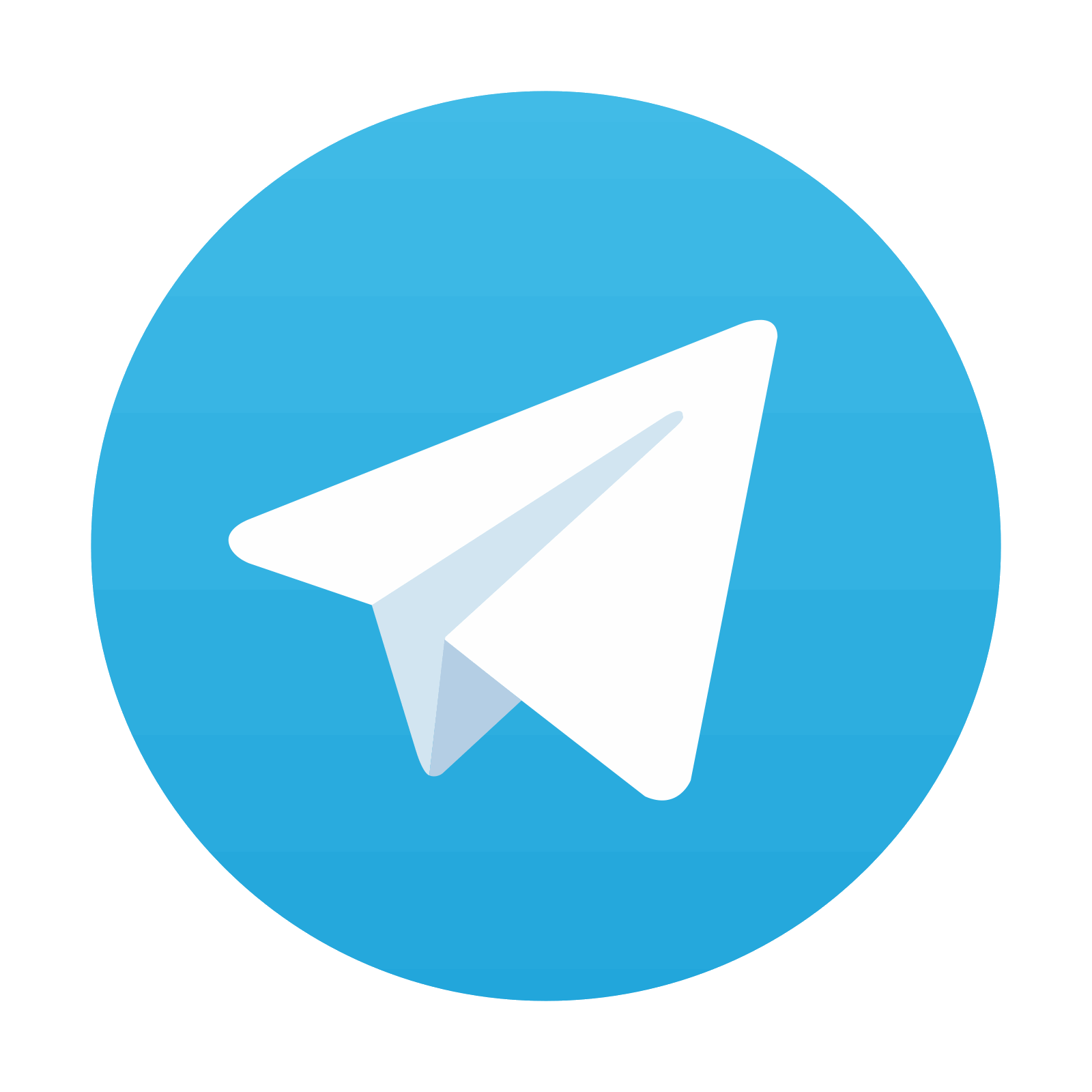
Stay updated, free articles. Join our Telegram channel
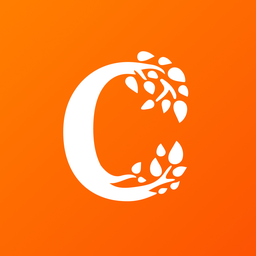
Full access? Get Clinical Tree
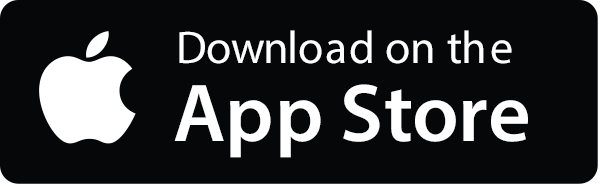
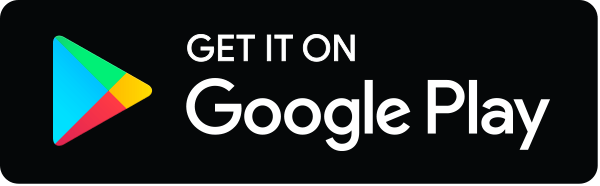