Amandeep S. Chohan1 and Elizabeth B. Davidow2 1 Department of Surgical and Radiological Sciences, School of Veterinary Medicine, University of California–Davis, Davis, California, USA 2 Timberline Veterinary Emergency and Specialty, Seattle, Washington, USA The body is principally composed of water, a triatomic molecule composed of two hydrogen molecules bound by covalent bonds to one oxygen molecule (H–O–H). Water behaves like a charged molecule: a dipole created by negatively charged oxygen and positively charged hydrogen. This polarity is responsible for imparting many of water’s chemical and physical properties such as high surface tension, high specific heat, high heat of vaporization, low vapor pressure, and a high boiling point. Because of water’s ionizing nature, substances dissolved in water segregate into individual components. Water also exists in an ionized form, composed of a negatively charged hydroxyl ion (OH−) and a positively charged protonated ion (H3O+). The aqueous milieu created by water provides the framework where all the metabolic and enzymatic processes take place. Water is the single most abundant compound in the body, making up the majority of body weight. On average, water constitutes approximately 60% of adult mammalian body weight. Age, body composition, and sex can influence total body water (TBW). Neonatal animals have greater TBW compared to adults (80% versus 60%), and an age‐related decrease has been described in companion animals during the first six months of life [1]. Adipose tissue has less water content compared to lean body tissues like muscle, so estimation of TBW needs to consider body condition since obesity can lead to overestimation. To understand distribution of body water, the body fluids can be thought of as existing in two major compartments: intracellular fluid (ICF) and extracellular fluid (ECF). ECF can be further divided into two compartments: interstitial fluid (ISF) that surrounds the cells, and intravascular fluid (IVF) that is contained within the vessels. The largest volume of water in the body is contained within the cells, making ICF the largest contributor to body weight. Of TBW, about two‐thirds (i.e., 40% of body weight) is ICF. The remaining one‐third of TBW (i.e., 20% of body weight) is ECF where it is distributed between ISF and IVF in a ratio of 3:1. About three‐quarters of ECF (i.e., 15% of body weight) is ISF that bathes the cells and one‐quarter of ECF (i.e., 5% of body weight) is IVF (i.e., plasma volume). In addition to water, body fluid compartments also contain varying concentrations of different solutes (Table 32.1). This heterogeneity is because the membranes separating fluid compartments have different permeability characteristics for various solutes. The ICF and ECF are separated by cell membranes, while capillary endothelium separates ISF and IVF. Endothelium is freely permeable to water and various ionic solutes, and the concentration of these is similar in ISF and IVF. On the other hand, the cell membrane is freely permeable to water, but by utilizing various carrier proteins, active transport and passive diffusion mechanisms maintain a specific intracellular milieu, resulting in very different solute concentrations in ICF compared to ECF (Table 32.1). The Gibbs–Donnan equilibrium results in slightly different concentrations of cations and anions in the ISF and IVF (i.e., plasma), but clinically this difference is negligible and the plasma concentration of various ions is considered to be a reflection of ECF solute concentrations. The major cation in ECF is Na+, with Cl− and HCO3− being the most abundant anions. In contrast, the major cations in ICF are K+ and Mg2+, and the most common anions are organic phosphates and proteins. Movement of water between ICF and ECF is governed by the osmotic gradient created by the differing concentrations of solutes between these two fluid compartments. The concentrations of solutes within a solution determine osmolality, which is dependent only on the number of particles in solution and not on their chemical formula, valence, molecular weight (MW), or size. Osmotic pressure due to solutes is expressed as milliosmoles per kilogram of water (mOsm/kg). In biologic fluids, the difference between osmolarity (solute osmotic concentration per unit volume of solution) and osmolality (solute osmotic concentration per unit mass of solvent) is not significant, and the milliosmolar concentration of a solution can be expressed as milliosmolarity or milliosmolality of solution. Osmolality (i.e., the number of osmotically active particles) in part determines the volume of water in ECF and ICF. Solutes that cannot cross the cell membrane contribute to effective osmolality (or tonicity) and are called “effective osmoles.” On the other hand, solutes that can freely diffuse across the cell membrane (like urea) are called “ineffective osmoles” and do not contribute to fluid shifts across cell membranes since they are in equilibrium on both sides of the semipermeable membrane. Osmosis is the movement of water across the semipermeable membrane from an area of lower (low solute concentration) to higher (high solute concentration) osmotic pressure. Table 32.1 Solute composition of various body compartments. In both the plasma and ISF, osmolality is mainly the result of ions such as Na+, K+, Cl−, HCO3−, glucose, and urea. Large molecules like albumin, though an important component of colloid osmotic pressure (COP), contribute little to osmolality. Being the most abundant cation in ECF, Na+ and its associated anions are responsible for the majority of ECF osmolality. The ECF osmolality (essentially the plasma osmolality) can be estimated by using the following formula: where Na+ and K+ are expressed in mEq/L, and glucose and blood urea nitrogen (BUN) are expressed in mg/dL. Glucose is an effective osmole by not being freely permeable through cell membranes, but urea is not an effective osmole because it is freely permeable across cell membranes. The concentration of all effective and ineffective osmoles is referred to as “plasma osmolality,” but the concentration of only effective osmoles refers to “effective osmolality” or “tonicity.” When the concentrations of various solutes on either side of the cell membrane are not disturbed (e.g., equilibrium), the fluid is in osmotic equilibrium (same osmolality), and there is no gradient for the movement of water between the two fluid compartments. If there is extracellular loss of free water, the concentration of solutes increases in the ECF, leading to generation of higher osmolality (i.e., osmotic pressure) compared to ICF, resulting in osmotic pull causing net movement (osmosis) of water through the cell membrane into the ECF until a new osmotic equilibrium is achieved. Hence, water is lost from the ICF to replace an ECF free water deficit. Osmolality can be either measured or calculated (as described earlier). Osmolality is measured clinically by freezing point depression of a serum sample. Normal serum osmolality is around 300 mOsm/kg. Clinically, the measured osmolality may not be equal to the calculated value, and the difference between the two (osmolal gap) can be used to predict the presence of unmeasured solutes (such as ethylene glycol and acetone). Fluid shifts between IVF (plasma) and ISF compartments occur at the level of capillaries. The fundamental principles that govern movement were described by Starling in 1896 [2]. Fluid movement across the capillary wall is determined by the imbalance between the osmotic absorption pressure created by plasma proteins (COP) and the capillary hydrostatic pressure. The capillary endothelial barrier slowly leaks plasma proteins into ISF. Staverman’s osmotic reflection coefficient, σ, can be used to quantify the degree of leakiness of capillary membrane to any particular solute. It ranges from 1 (i.e., 100% reflection or not permeable) to 0 (i.e., no reflection or completely permeable). For a membrane separating solutions of a single solute at two different concentrations, the principle of irreversible thermodynamics states that where Jv is the volume filtration rate per unit membrane area A, Lp represents the hydraulic permeability, ΔP the difference in hydrostatic pressures, and Δπ is the difference in osmotic pressures across the membrane. IVF and ISF each contain many solutes (species “n”). Therefore, to describe the movement of fluid across the capillary wall, the above equation can be written as: where Under normal circumstances, Pc is much greater than Pi, producing a hydrostatic pressure gradient at the arterial end that favors movement of fluid out of the capillary into the interstitium. Capillary blood pressure is decreased from the arteriolar to venous end of the capillary, while the πp stays almost the same, resulting in an oncotic pressure gradient that favors the movement of fluid from the interstitium into the capillaries at the venous end [3]. Altogether there is a net filtration pressure that is responsible for the movement of water out of the capillaries into the interstitium. Some of the filtered fluid is normally returned back to the circulation via the lymphatic system. As the understanding of microcapillary structure has evolved, it has been proposed that the filtration properties of the capillary wall actually reside in an endothelial surface layer (ESL) that is composed of a matrix of membrane bound complex sugars called the endothelial glycocalyx (EG). The matrix covers the intercellular clefts and the endothelial cells on the luminal side of the capillaries [4,5]. Traditional Starling’s forces describe that the fluid filtered at the arteriolar end of the capillary is absorbed back into the circulation at the venous end under the influence of the dominant osmotic pressure gradient generated by the difference, πp − πi. Recently, it has been shown that the effect of πi on fluid exchange at the capillary level is much less than predicted by Starling’s original equation. It is now established that many capillaries filter fluid into the ISF throughout their length, and absorption at the venous end of the capillaries does not take place. Plasma protein oncotic pressure (COP) opposes this filtration but does not change the direction of net filtration (i.e., filtration is always occurring toward the ISF). Most of the filtered fluid returns to the circulation via lymphatics. The EG layer separates plasma from a subglycocalyx space that contains a very small amount of protein. This subglycocalyx COP (πg) should replace πi in the Starling’s equation to determine the transcapillary flow (Jv): Low protein concentration in the subglycocalyx space results in a low value of πg causing a higher value for (πp − πg), that is, a bigger opposing force against the transcapillary flow (Jv) that occurs under the influence of differences in hydrostatic pressures around the capillary membrane (Pc – Pi). The fact that the low value of πg results in low Jv, coupled with the important contribution of lymph flow in various tissues in returning fluid back into circulation, are critical features on which the glycocalyx model of transvascular fluid exchange is based. An index of vascular permeability is transcapillary escape rate of albumin (TCERA). In humans, it is about 5% of the plasma albumin per hour. This rate could double during surgery or increase 20% or more in septic patients. Evidence points to a compromised EG in those patients. Loss of plasma albumin causes reduction in πp leading to an increase in Jv. Increasing the πp (COP) in septic patients with synthetic or natural colloids will decrease Jv and prolong vascular expansion compared to crystalloids, though the use of artificial colloids is controversial in septic patients (see discussion below). Crystalloid solutions are prepared by dissolving crystalline compounds in water. Most of the clinically used crystalloids are either polyionic sodium‐based or dextrose solutions in water. Crystalloids are classified mostly based on their tonicity relative to plasma. Isotonic crystalloids have similar tonicity/osmolality compared to the plasma. This means that administration of isotonic fluids will not cause any change in the solute concentration of the ECF. There will not be any alteration in the net osmotic forces that might trigger the water movement between ICF and ECF. Hence, the isotonic crystalloid fluid will stay within the ECF, and no alteration in ICF volume will take place unless previous free water loss occurred from the ICF compartment. After intravenous administration, isotonic crystalloids stay within the ECF and redistribute into the IVF and ISF. This redistribution occurs according to the normal fluid distribution in the body so that within 30–60 min post infusion, only about one‐quarter of the administered amount remains intravascular and the rest moves out to the ISF [6,7]. The most commonly used isotonic fluids and their constituents can be found in Table 32.2. Isotonic fluids contain mixtures of various electrolytes found in plasma with or without additives, which affect the acid–base status of the patient. Some crystalloids contain dextrose. Except for physiological (or “normal”) saline (0.9% NaCl), most of the commonly used crystalloids contain a precursor for bicarbonate production such as lactate or acetate and gluconate ions (Lactated Ringer’s solution [LRS], or Normosol® and Plasma‐Lyte®, respectively). Lactate ions undergo either oxidation or gluconeogenesis primarily in the liver, although kidneys and muscle tissues also participate to some extent. During glycogenesis, lactate is converted to glucose via pyruvate, and this process utilizes H+. So lactate also has a bicarbonate‐sparing effect. Acetate is metabolized mostly in muscle, and gluconate can be metabolized by most tissues. Because these crystalloids increase bicarbonate level in the plasma, they have been called “alkalinizing fluids.” 0.9% sodium chloride, on the other hand, is an “acidifying solution” due to plasma bicarbonate dilution (dilutional acidosis) and high Cl− content that can decrease the strong ion difference and cause hyperchloremic metabolic acidosis [8–10]. The composition of some crystalloids more closely matches plasma (e.g., LRS, Plasma‐Lyte®‐148, Normosol®‐R) compared to others (e.g., 0.9% NaCl), and they are considered balanced isotonic crystalloids in comparison to unbalanced crystalloids. Another way of looking at crystalloids is from the standpoint of their clinical use. Most of the isotonic crystalloids, because of their similar osmolality and solute composition to plasma, do not cause osmotic water shift from blood cells to plasma and can be used for emergency volume resuscitation and are, therefore, called “replacement fluids.” Table 32.2 Characteristics of various isotonic crystalloids compared to plasma. To understand the clinical use of various crystalloids, it is imperative to understand which body compartment is deficient in fluid (intravascular/perfusion versus intracellular/hydration) [11]. When isotonic crystalloids are used for replenishment of IVF and electrolyte deficits, about 75% of the fluid redistributes to ISF. Therefore, relatively large volumes are usually administered to replenish intravascular deficits. In dogs, Silverstein et al. demonstrated the potential of isotonic fluids to increase the blood volume [12]. Immediately following the infusion of fluid, the blood volume increased by 76%, more than 3–4.5 times the increase following hypertonic fluid and colloids. However, at 30 min post infusion, the increase was only 35%. The initial increase in blood volume was attributed to the greater volume of isotonic crystalloids administered. More importantly, the authors also calculated the efficacy ratio (ER; increase in blood volume compared to the volume of fluid infused), and isotonic fluid had an ER of only 0.4 at 30 min post infusion. Typically, three to four times the deficit volume of crystalloid fluids is recommended to replenish the intravascular space. Shock, hemorrhage, and severe volume deficit due to diarrhea, vomiting, excessive diuresis, or third spacing constitute common scenarios when these fluids are administered. Crystalloids are also administered during most elective surgery procedures in healthy patients. It is often recommended that patients with shock should receive up to one blood volume (e.g., 80–90 mL/kg in dogs) in an hour, starting with one‐quarter to one‐third of the shock dose given incrementally with constant monitoring for desired endpoints to guide fluid therapy further (i.e., goal‐directed fluid therapy). A typical fluid rate used for patients under general anesthesia is 5–10 mL/kg/h [13,14], although recent trends have been to reduce these rates further in patients without risk factors for perioperative fluid loss. Slight variability in composition and concentration of various solutes (electrolytes and buffers) in different isotonic crystalloids (see Table 32.2) may make administration of one solution preferable in certain clinical situations. Patients with hypochloremic metabolic alkalosis and hypercalcemia may benefit from the use of 0.9% saline [15,16]. Alkalinizing fluids are not used very commonly in adult dehydrated cattle because metabolic acidosis is not a common occurrence except in cases of grain overload, hepatic lipidosis, chronic ketosis, or kidney disease [17,18]. Patients that are acidotic may benefit from the use of alkalinizing fluids. LRS can contain either a racemic mixture of both L‐lactate (i.e., S(+)‐lactate) and D‐lactate (i.e., R(–)‐lactate), or just L‐lactate. Fluids containing acetate might have a more profound alkalinizing effect, as dogs cannot metabolize D‐lactate as readily [15]. Various isotonic crystalloids have different Na+ ion concentrations, giving them a range of osmolalities (see Table 32.2). In severe electrolyte disturbances, it is recommended to use a fluid with a Na+ concentration closest to that of the patient for resuscitation to avoid rapid changes in Na+ ion concentration (Na+ concentration should not change more than 0.5–1 mEq/L/h). While resuscitating patients with head trauma or cerebral edema, replacement crystalloid solutions with the highest Na+ concentration (i.e., 0.9% NaCl) will be least likely to reduce plasma osmolality that might favor the movement of water into brain cells. With a Na+ content of 130 mEq/L, LRS is relatively hypotonic compared to plasma and has been associated with increased intracranial pressure in some models of traumatic brain injury [19,20]. ISF accumulation/edema can occur after excessive fluid administration, especially in patients suffering from oliguric renal failure, cardiac insufficiency, head trauma, pulmonary contusions, or decreased COP. In clinical scenarios characterized by active intracavitary (abdominal or thoracic) or intracranial bleeding, aggressive fluid administration to achieve normotensive resuscitation endpoints before surgical control of bleeding is achieved could increase hydrostatic pressure and might lead to disruption of blood clots and worsening of bleeding. “Hypotensive” fluid resuscitation is often advocated in these patients, aiming to achieve the lower limit of clinically acceptable mean arterial pressure (60 mmHg, corresponding to a minimally adequate perfusion pressure of various vital organ systems). Others suggest delayed fluid resuscitation until hemostasis is achieved; however, the risk of anesthetizing hemodynamically unstable patients should be weighed against the perceived risk of volume resuscitation. The veterinarian should also be cognizant of the possibility of excessive hemodilution of clotting factors, platelets, plasma protein, and red blood cells (RBCs) when large volumes of fluid are administered [6,7,21]. This may be even more relevant in patients undergoing surgical procedures associated with more blood loss or patients that already have pre‐existing coagulopathies, hypoproteinemia, or anemia. Due to the presence of Ca2+ ions in LRS, it is recommended that blood products not be administered in the same fluid line since Ca2+ may antagonize common anticoagulants, leading to microemboli. Studies have concluded that rapid transfusion rates for whole blood (WB) or packed red blood cells (PRBCs) can be safely used with LRS [22] and that LRS can be safely used to dilute citrate phosphate dextrose preserved PRBCs in emergency settings [23]. Because of the liver’s major contribution to lactate metabolism, there has been some concern about the use of LRS in patients with hepatic insufficiency. However, studies have failed to show any increase in blood lactate levels after LRS administration [24,25]. Hyperlactatemia is common in cancer patients due to use of anaerobic glycolysis by neoplastic cells, but a study in dogs suffering from lymphoma showed only a transient increase in lactate levels that became normal after 2 h of infusion of LRS [26]. Large quantities of rapidly administered acetate solutions can cause vasodilation due to release of adenosine from muscles that could cause hypotension, especially in hypovolemic patients [27–29]. Hypertonic solutions have an increased tonicity/osmolality compared to plasma. Administration of hypertonic fluids will increase osmotic pressure in the IVF and set up a gradient for the movement of water from ISF as well as ICF (with lesser osmotic pressure; lower solute concentration). The most commonly used hypertonic fluids in clinical practice are hypertonic saline solutions (HSSs; Table 32.3). The reflection coefficient of Na+ for cell membranes is maintained close to 1 by the Na+‐K+ ATPase pump, whereas the reflection coefficient of the endothelial membrane is only 0.1. This means that most of the movement of water after HSS administration is from the ICF rather than ISF [30]. Hence, fluid is pulled out of the cells (cellular dehydration) and interstitium into the intravascular space. Analysis of transcapillary driving pressures (governed by Starling’s forces) supports an immediate plasma volume expansion under the effect of HSS that rapidly reverses the transcapillary pressure gradient from a small filtration force under normal physiologic conditions to a large absorptive force (osmotic pressure gradient). Due to a very low reflection coefficient of capillary endothelium for Na+, the generated osmotic pressure is transmitted across the capillary endothelium to the cell membrane and is responsible for pulling the water out of cells. As HSS itself does little to address TBW deficits, it is imperative that hypertonic therapy be followed by administration of appropriate follow‐up fluids (isotonic crystalloids, colloids, or blood products). In cattle, it was recommended, after using hypertonic fluids, to administer fluids ororuminally if the animal does not consume more than 5 gallons of fluid orally [17,31]. HSSs are unbalanced crystalloids and are available in various concentrations having very high osmolality compared to plasma (see Table 32.3). Clinically, 7.5% HSS is most commonly used. Although mannitol is also another hyperosmotic agent, its osmotic effects are typically delayed by 15–30 min [32] and effects are not as profound as 7.5% NaCl because its osmolarity is only half (1250 mOsm/L for 25% mannitol) as much. The discussion here will focus on HSS only. Table 32.3 Characteristics of various hypertonic saline solutions compared to plasma. In hypovolemic animals (hemorrhagic shock models), plasma volume expansion at the end of an HSS bolus/infusion given over 1–10 min at a dose of 4–8 mL/kg varied from 10 to 21 mL/kg with higher doses causing more volume expansion. However, HSS quickly equilibrated within ECF so that after 1–3 h, there was minimal plasma volume expansion [33–36]. In normovolemic dogs, Silverstein et al. showed that the ER (defined as the relative increase in blood volume in relation to the volume infused) was maximum with HSS immediately post infusion (2.7 ± 0.5; approximately three times that of other fluid types) and was still higher at 30 min post infusion compared to isotonic crystalloids and synthetic colloids [12]. In order to prolong the duration of effect, HSS was combined with a colloid (6% dextran 70 [HSD]) that not only increased the extent of immediate plasma volume expansion but the plasma volume expansion persisted 3 h later [34,35]. Other available colloids like hydroxyethyl starch (HES) can also be combined with HSS. This hypertonic–hyperoncotic combination constitutes “small‐volume fluid resuscitation” [17]. Usually, it is constituted by diluting 23.4% HSS with the colloid in the ratio of 1:2.5, or the two can be used separately (2–6 mL/kg of 7% HSS plus 4–10 mL/kg of colloid) [17,31]. Cardiovascular effects of HSS administration are characterized by an increase in preload, heart rate, and myocardial contractility and a decrease in systemic vascular resistance (SVR), leading to an increase in cardiac output (CO) [37–39]. Beneficial immunomodulatory effects have been described characterized by decreased interaction of neutrophils with endothelial cells, reduced neutrophilic activation, and improved activity of lymphocytes and natural killer T cells [40–42]. HSSs have also been shown to reduce cytotoxic edema and improve splanchnic and muscle capillary perfusion in animal injury models of soft tissue contusions, hemorrhagic shock, and superior mesenteric artery occlusion [43–47]. Most of the clinical and experimental literature on HSS/HSD suggests its application in the management of hemorrhagic hypovolemic shock [33–36,48,49]. Another area where HSS has been shown to be beneficial is in the management of intracranial hypertension. Although brain trauma management foundation guidelines [50] still recommend mannitol as the primary hyperosmolar therapy (Level II evidence), new evidence has been emerging that shows hypertonic saline to be a better choice [51–56]. Benefits of hypertonic resuscitation have also been documented in the canine model of gastric dilation‐volvulus‐induced obstructive shock [57], canine model of bile‐induced pancreatitis [58], and murine models of acute pancreatitis [59,60] Large animal practice has found HSS/HSD to be particularly helpful where the patient’s large blood volume makes volume expansion challenging. Oral rehydration therapy has been suggested as acceptable if the calf or cattle is < 8% dehydrated, but a combination of HSS intravenous therapy plus oral rehydration was shown to be successful if dehydration was ≥ 8% [17,31]. Use of hypertonic–hyperoncotic resuscitation has been described in horses with endotoxemia [61,62], in calves with endotoxemia [63] or diarrhea [64–66], and in adult endotoxemic cattle [67]. Use of HSS in patients with uncontrolled hemorrhage can lead to increased bleeding and worsened outcome compared to isotonic resuscitation. However, studies demonstrate that if the hemorrhage is controlled, then HSS can improve hemodynamic variables and final outcomes [68–70]. It has been shown that if a bolus of hypertonic–hyperoncotic fluid (4 mL/kg) is administered slowly (over about 12 min) compared to 1 min, blood loss was significantly reduced and survival improved [69,71]. Pre‐existing dehydration has been suggested as a contraindication to administration of HSS because a dehydrated intracellular environment could decrease the efficacy of intravascular hypertonic fluid resuscitation. Studies performed in dehydrated hemorrhagic swine [72] and sheep [73,74] models with preinfusion plasma osmolalities of 325–340 mOsm/L showed that administration of 4 mL/kg of 7.5% NaCl achieved hemodynamic stability and an increase in plasma volume similar to euvolemic animals. When a rapid onset of action is desired, HSS can in fact be considered as an adjunct with isotonic resuscitation to correct hypovolemia in dehydrated patients. A recent study in endurance horses showed that horses resuscitated with 2 L of HSS followed by 5 L of isotonic saline achieved normal packed cell volume (PCV) and total protein (TP) compared to horses that received 7 L of 0.9% sodium chloride alone that demonstrated residual hemoconcentration [75]. Keeping in mind the diuresis that occurs after HSS administration and mobilization of ICF to the intravascular space with the potential to be filtered, the possibility of subsequent dehydration must be considered in veterinary patients, especially when appropriate follow‐up fluid administration is delayed or impossible (e.g., some field conditions). HSS has been associated with cardiac arrhythmias, bradycardia, hypotension, and volume overload. Such effects are noted mostly when high doses of higher concentration HSS are administered at a very fast rate [76]. The recommended administration regimen of 7.5% NaCl at a dose of 4–6 mL/kg given at a rate no faster than 1 mL/kg/min is not usually associated with complications. Use of recommended doses rarely causes any consistent blood chemistry abnormalities, but the potential for hypernatremia, hyperchloremic metabolic acidosis, hyperosmolar renal failure, and hypokalemia should be considered especially if pre‐existing electrolyte abnormalities or repeated dosing with HSS are considered [8–10,21]. Ajito et al. [77] administered doses of 2.5, 5, and 15 mL/kg of 7.2% NaCl at a rate of 1.6 mL/kg/min to anesthetized Beagle dogs. Administering 5 mL/kg caused only transient elevations in Na+ (153 at 3 min; 165 at 5 min; baseline 142) that returned to below 160 mEq/L at the end of infusion, whereas a dose of 15 mL/kg led to consistent hypernatremia (161–174 mEq/L) from 10 to 90 min after initiation of infusion. A study conducted in dogs showed that the maximum tolerated dose of 7.5% NaCl was 20 mL/kg, although that dose caused transient neurological signs like pacing and lethargy, which resolved within 24 h [78]. Hypotonic crystalloid solutions have lower tonicity/osmolality than plasma (Table 32.4). Dextrose in water is a source of free water because the dextrose is metabolized leaving water that is free of osmotic particles. Dextrose administration decreases ECF solute concentration compared to ICF, creating an osmotic pressure gradient for movement of water from the ECF to ICF (cellular swelling). Less than 10% of infused hypotonic fluid remains in the IVF space after 30–60 min. Due to their large volume of distribution and potential for cellular swelling, hypotonic fluids are not indicated for intravenous volume replenishment. They are used mostly to provide maintenance needs of patients in which water intake is absent because they are anorexic (no feed intake), unable to drink, and/or fluid losses are not beyond normal physiologic losses (unlike vomiting, diarrhea, etc.) [79]. Maintenance fluid requirements can be calculated in several ways [80,81]. The simple empirical formula of 40 mL/kg/day (in large dogs) or 60 mL/kg/day (small dogs and cats) can be used. Since water requirements are tied to metabolism, daily maintenance energy expenditure (and water requirement) may be calculated as 140 kcal × body weight (kg)0.75 [80]. Another formula used to calculate maintenance water requirement is [30 × body weight (kg)] + 70, but if the patient weight is less than 2 kg or more than 50 kg, then it is proposed to use the caloric requirement to calculate the maintenance fluid requirements [80,81]. One milliliter of water is required for every kcal of energy consumed. The ongoing physiologic fluid losses may consist of insensible (feces, saliva, skin, and respiratory tract) and/or sensible (urinary) losses. These losses are typically hypotonic in nature with low Na+ and high K+ compared to that of ECF [81]. Hypotonic fluids are manufactured to match this composition (Table 32.4). However, in patients with normal renal function, isotonic replacement fluids with some added K+ can also be used for maintenance purposes because kidneys can excrete excess electrolytes. This is a frequently used method in many veterinary clinics due to a combination of economic pressures to maintain low inventory as well as lack of familiarity with maintenance/hypotonic crystalloids. Other major indications for hypotonic crystalloids involve free water supplementation (e.g., hypernatremia and diabetes insipidus), dextrose supplementation during hypoglycemia, and perioperative fluid therapy for patients at risk of volume overload (i.e., congestive heart failure). It is important to administer these fluids at a slow rate so that sudden and excessive dilution of plasma solutes is avoided as the reduced ECF osmolality could cause cellular swelling (cerebral edema). It is advisable to monitor electrolyte concentrations in patients receiving hypotonic fluids. Colloids are composed of macromolecular particles suspended in a crystalloid fluid solution. In the absence of increased microvascular permeability, their movement is limited across the endothelium, and they are less able to accumulate in the interstitium. This results in a longer dwell time within the IVF compartment compared to crystalloids, and they can pull fluid into the IVF compartment based on their oncotic properties. Colloidal oncotic pressure is the osmotic pressure generated by the colloids in solution and is determined by the number of particles rather than their size. In clinical practice, colloids are mostly used in patients requiring oncotic support (hypoproteinemia) to prevent ISF accumulation or in hypovolemic patients as a resuscitative fluid [82–87]. Colloidal preparations can be classified as consisting of blood component products (discussed later in this chapter) or synthetic compounds (discussed below). Table 32.4 Characteristics of commonly available hypotonic crystalloids compared to plasma. Synthetic colloids contain compounds composed of three major groups: the hydroxyethylstarch derivatives, dextrans, and gelatins. Most of the following discussion revolves around HES solutions due to their common use in clinical practice, but some basics about dextrans and gelatins are also presented. HESs are the synthetic colloid preparations most commonly used in both human and veterinary medicine. Chemically, HES preparations are modified polymers of amylopectin, a polysaccharide very similar to glycogen. The polymers are suspended in saline or a balanced electrolyte crystalloid solution. Different preparations of HES with variable structural characteristics (Table 32.5) have different physicochemical properties governed by three main variables: mean molecular weight (MW), molar substitution (MS), and C2:C6 ratio. It is important to understand these differences in order to appreciate the clinical variability with respect to the pharmacokinetics, pharmacodynamics, and adverse effect profile of various HES preparations [88–90]. HESs are typically identified by three numbers (e.g., 6% HES 130/0.4). The first number dictates the concentration of the solution, the second represents the manufactured mean MW in kilodaltons (kDa), and the third indicates the MS. The concentration of HES influences the initial volume expansion. 6% solutions are iso‐oncotic, and 10% solutions are hyperoncotic with considerably more volume expansion. MW is the weight‐averaged (Mw) or number‐averaged (Mn) size of the polymers. Since the polymers actually are of varying length (ranging from a few thousand to few million daltons), this parameter is only an average. HESs are arbitrarily classified by the manufactured mean MW into high‐MW (> 400 kDa), medium‐MW (200–400 kDa), and low‐MW (< 200 kDa) preparations. As Mw takes into account the individual weights of different particles, it is influenced by the presence of large molecules in the system and will give a larger value compared to the Mn that represents the total weight of all the molecules divided by the number of molecules. The ratio Mw:Mn provides an index of the polydispersity (that is, the variation in particle size). It is important to understand that the given MW applies to the colloidal preparation in vitro because soon after these fluids are administered to a patient, the low‐MW fraction is excreted by the kidneys and larger molecules are progressively hydrolyzed by serum α‐amylase, resulting in a narrow distribution of MWs with an in vivo mean MW that is lower than the mean MW of the infused preparation. The decrease in COP after initial excretion of the low‐MW fraction is partly compensated for by the availability of a greater number of small MW molecules created by metabolism (cleavage) of larger molecules. Molar substitution (MS) describes the degree of substitution of glucose molecules with hydroxyethyl groups. This substitution increases the polymer solubility and inhibits the metabolism of the polymer by amylase. The degree of MS can be calculated in two ways. One method, termed the “degree of substitution” (DS), is calculated by the number of substituted glucose residues divided by the total number of glucose residues. With the second method, MS is calculated as the average number of hydroxyethyl residues per glucose unit. More than one substitution can occur at each glucose residue, so the MS could be higher than the DS. The value of 0.4 in the 6% HES 130/0.4 indicates that there are four hydroxyethyl residues on average per 10 glucose subunits. Based on DS, HESs have been referred to as “hetastarch” (≥ 0.7), “hexastarch” (0.6), “pentastarch” (0.5), and “tetrastarch” (0.4). The greater the MS, the harder it is to metabolize the HES. The C2:C6 ratio describes the pattern of hydroxyethylation and also impacts the pharmacokinetics of HES preparations. Hydroxyethyl groups at position C2 of the glucose molecule inhibit the access of α‐amylase to the substrate more efficiently than the C6 position. HES products with high MW, larger C2:C6 ratio, and greater MS are metabolized slowly and have prolonged persistence in plasma. Based on these physicochemical properties, HES preparations can be classified in a more clinically relevant way as slowly degradable (e.g., hetastarch) or rapidly degradable (e.g., Voluven® or Vetstarch®) preparations (see Table 32.5). Pharmacokinetics and pharmacodynamics of various HES preparations are complex in part due to their polydisperse nature [91]. After intravenous administration, particles having MWs below the renal threshold (45–60 kDa) are quickly filtered into the urine. Additionally, small amounts diffuse into the interstitium of tissues, eventually redistribute, and are subsequently eliminated. A transient proportion is stored in tissues and degraded by the reticuloendothelial system. Larger molecules are retained in the plasma for a variable period of time subject to their metabolism by α‐amylase. Metabolism is, in turn, influenced by the physicochemical properties of the particular HES preparations as described earlier [88–90]. The resulting smaller fractions after metabolism are further excreted in urine. Studies in animals and humans have demonstrated significantly more accumulation of slowly degradable HES preparations compared to rapidly degradable preparations, especially after repeated administration [91]. Clearance of tetrastarches has been shown to be about 23 times faster than hetastarch and five times greater than pentastarch. The pharmacokinetics of HES have been described in dogs and horses [92–94]. Species‐specific differences in plasma amylase activity have also been documented and contribute to the variable half‐life of HES in different species. Relative to humans, amylase activity has been shown to be greater in dog plasma [92] and less in equine plasma [93]. Table 32.5 Characteristics of hydroxyethyl starch (HES) preparations commonly used in veterinary anesthesia. MS, molar substitution; COP, colloidal osmotic pressure. The pharmacodynamics of synthetic colloid fluids are related to the volume and duration of intravascular volume expansion and may be influenced by many factors including dose, speed of administration, specific colloid preparation, microvascular permeability, species of animal, and preinfusion intravascular volume status [88–91,95]. Iso‐oncotic (6%) preparations of HES have been associated with similar increase in intravascular volume compared to the volume administered, while the hyperoncotic preparations (10%) can expand volume up to 145% by pulling fluid from the interstitial space [88,89]. The initial increase in plasma volume is the result of COP of the colloidal preparation administered and is mostly attributed to the large number of small MW particles. As these smaller molecules are removed from the circulation during the first few hours, a significant decrease in COP occurs, translating clinically into reduced effect on plasma volume expansion over time. Due to the presence of the remaining large MW particles in the plasma, the concentration of the colloidal preparation (mass per unit volume) may still be quite high, but the COP will be low. Slowly degradable preparations have a longer plasma half‐life, but this does not translate into an increased duration of volume expansion. In spite of minimal plasma accumulation of tetrastarch, studies in orthopedic [96,97] and cardiac [98] surgeries have demonstrated its duration of effect (at similar concentration) to be comparable to pentastarch [99] and hetastarch [96,100]. It is important to understand that though the clearance and residual plasma concentration of HES are affected by their physicochemical properties (mainly MS and C2:C6 ratio [101]), the COP depends upon the number of osmotically active particles and not on the concentration of HES [88–90,95]. In a given volume of HES solution, there will be more particles of lower MW product compared to an HES preparation with higher MW translating into a greater COP at similar plasma concentrations. The effect of hetastarch on COP has been described in healthy ponies and horses, hypoproteinemic dogs and horses, hypovolemic, and hypotensive dogs under anesthesia, and dogs undergoing orthopedic surgery [82,83,102–107]. Effects of various HES preparations on COP do not seem to last beyond 24 h. It is important to remember that variables like health status [104,105], pre‐existing volume status [82,91,95], concurrent fluid therapy [106,108], dose [107], type of preparation [83,93,102], and anesthesia [109] all have the potential to influence plasma COP, meaning that results of different studies must be evaluated carefully. A study in horses reported that COP was higher in the group receiving tetrastarch (130/0.4) compared to hetastarch (600/0.75) but returned to baseline at 24 h [83]. Due to its ability to provide colloidal oncotic support and prolonged intravascular retention, HES has proven to be better at providing intravascular support and improvement in hemodynamic indices compared to crystalloids [82–87]. Adverse events have been associated with HES use in clinical patients. It is important to point out that results from in vitro studies cannot be directly extrapolated to clinical scenarios (in vivo) as the side‐effect profile is heavily impacted by the in vivo pharmacokinetic behavior of various HES products and slowly degradable preparations (hetastarch and pentastarch) carry more risk compared to rapidly degradable preparations (tetrastarches) [88–90]. All types of fluids have the potential to cause dilutional coagulopathy, but the HES solutions also impact hemostasis directly by altering both primary and secondary hemostasis [88]. HES decreases the expression of platelet surface GP IIb‐IIIa complex and also physically coats the surface of platelets, causing decreased interaction of agonists like soluble fibrinogen and von Willebrand factor (vWF) with this receptor, thus preventing platelet aggregation. HES also decreases factor VIII/vWF complex concentration, possibly due to accelerated elimination of this complex after binding to HES. These adverse effects have clinically translated into increased blood loss perioperatively. Use of rapidly degradable HES preparations (HES 130/0.4) caused lower blood loss in various types of surgeries in humans compared to slowly degradable HES preparations (HES 200/0.5, HES 450/0.7, and HES 600/0.75) [96,110,111]. Desmopressin has been suggested as a treatment option for mild coagulopathy associated with HES administration [112]. Studies in dogs [103,106,113–118] and horses [62,83,102,107,119] have also reported dose‐related effects [107,116,117,119,120] of hetastarch and tetrastarch on platelet function and factor VIII/vWF complex [115–117,119–121] in vitro and in vivo but have failed to document increases in clinical bleeding [103,106,113]. Several of these studies documented less impact on hemostasis when tetrastarches were used [83,107,116,121]. Human studies [122,123] have shown that HES preparations formulated in a balanced carrier solution containing Ca2+ ions cause less impairment of hemostasis compared to 0.9% sodium‐chloride‐based formulations. Similar in vitro comparisons in dogs [115] and horses [119] did not show improved platelet function as assessed by estimation of platelet closure times. Concerns about hyperchloremic metabolic acidosis with 0.9% sodium chloride formulations have also been expressed [88]. A multicenter blinded trial in severely septic human patients revealed that HES 130/0.42 was associated with more mortality and need for renal replacement therapy compared to the Ringer’s acetate group [124]. A meta‐analysis in humans revealed an increased risk of acute kidney injury (AKI) in critically ill patients receiving HES preparations [125]. In dogs and cats, retrospective studies have shown conflicting impacts of HES on renal function [126–130]. One study revealed the use of 10% HES 200/0.5 and its increasing dose as a risk factor for AKI [126]. Other studies did not show any risk of AKI with 6% HES 130/0.4, but one study documented an association between the length of administration of 6% HES 130/0.4 and grade of AKI within 10 days of HES administration [127]. A case report documented exacerbation of renal dysfunction in a dog with pre‐existing azotemia that received a 10 mL/kg dose of 6% HES 130/0.4 in an effort to increase its osmotic pressure [131]. A subgroup analysis in two studies specifically performed in septic small animal patients did not reveal any association between HES and AKI [129–130]. Due to different doses and types of HES products, no standardization of AKI definition, and small sample size, it is difficult to draw any firm conclusions. Experimental studies did not reveal any association between 6% HES 130/0.4 and biomarkers of AKI within 3 or 72 h post‐HES bolus (20 mL/kg) administration [132,133]. Furthermore, a randomized clinical trial in dogs undergoing shock resuscitation with 6% HES 130/0.4 or Hartmann’s solution did not reveal any difference in urinary biomarkers of AKI [134]. Reports indicating a low incidence of anaphylactoid reactions, increases in α‐amylase level, and tissue accumulation of slowly degradable preparations causing pruritus in humans have been published [88,90]. In cats, hetastarch has been associated with vomiting if administered too rapidly. Most of the dosage recommendations in veterinary patients are extrapolated from manufacturer’s and human literature rather than veterinary‐specific studies, but it seems appropriate to not exceed a dose of 20 mL/kg/day for various types of HES formulations although doses of up to 50 mL/kg/day have been suggested in the past for tetrastarches due to their relatively less negative impact on hemostasis [83,107,116,121]. Increased blood loss was reported in dogs when a > 30 mL/kg dose of hetastarch was used [135,136]. A few studies in horses have suggested a hetastarch dose of 10 mL/kg/day is well tolerated [62,93]. Dextrans are linear polysaccharide molecules and include a low (10% dextran 40) and high (6% dextran 70) MW preparation in 0.9% saline. Smaller molecules are removed by glomerular filtration with bigger molecules staying in circulation longer and eventually being metabolized by dextranase enzyme systems in the kidney, liver, and spleen to produce carbon dioxide and water. Due to the importance of glomerular filtration in excretion of dextrans, half‐life can be increased in patients with renal insufficiency. Dextrans are hyperoncotic compared to plasma and cause plasma volume expansion that can be more than the volume infused (dextran 40 > dextran 70). Dextrans have been used to improve microcirculatory blood flow (especially dextran 40) and as thromboprophylaxis to prevent deep vein thrombosis in humans. Complications associated with their use include anaphylactic reactions, renal function impairment (especially with dextran 40), and impaired hemostasis (inhibited platelet aggregation, dose‐dependent hyperfibrinolysis, decreased levels of vWF, and factor VIII:C activity) [136–141]. They can also coat the surface of RBCs, interfering with cross‐matching procedures. A maximum dose of 10–20 mL/kg/day in dogs and 5–10 mL/kg/day in cats has been suggested to minimize adverse effects. Gelatins are large MW polydisperse proteins formed by hydrolysis of bovine collagen. Three modified newer generation gelatin preparations are succinylated or modified fluid gelatins, urea‐cross‐linked gelatins, and oxy‐cross‐linked gelatins (oxypolygelatins). Gelatin preparations have similar average MWs (30–35 kDa) and are supplied in concentrations of 3.5–5.5% [142]. The small size of gelatin molecules makes them susceptible to glomerular filtration, which is the main route of elimination. The half‐life of oxypolygelatin has been suggested at 2–4 h in dogs [138]. The distribution of total dose administered by 24 h is 71% in urine, 16% extravascular, and 13% remains in the plasma [142]. Rapid renal excretion means less plasma retention, and though similar side effects on coagulation compared to other synthetic colloids are reported, their magnitude is less [138,143,144]. Urea‐cross‐linked gelatins have been associated with anaphylactoid reactions due to histamine release that were blocked by prophylactic use of histamine blockers [145]. Although there are no standard guidelines for gelatin dosages in veterinary species, a dose of 5 mL/kg for oxypolygelatin in dogs has been recommended [138]. Most of the studies in veterinary medicine have looked at the effect of HES, dextrans, and gelatins in healthy animals and have documented dose‐related deterioration in both primary and secondary hemostasis but failed to show exacerbation of clinical bleeding. A meta‐analysis in humans documented increased blood loss with HES compared to crystalloid therapy in perioperative settings [146], and recent human guidelines published for trauma patients recommended avoiding use of HES due to the adverse impact on hemostasis [147]. As effects of synthetic colloids on hemostasis are directly linked to their plasma concentration, it is reasonable to assume that if they are used in dosages higher than the maximum recommended, are used repeatedly, are used when there is decreased clearance (e.g., renal impairment), or are used in patients with pre‐existing bleeding diatheses, the potential to increase clinical bleeding does exist. Renal filtration is the major route of HES excretion, and urine osmolality and specific gravity are increased in dogs administered HES 600/0.75 [148]. Hyperviscosity of the urine after glomerular filtration of colloid molecules theoretically causing tubular obstruction due to stasis of urine flow seems the most likely mechanism of renal impairment [90,140,141]. A case report and a retrospective study in critically ill dogs revealed a positive association between the histopathological evidence of osmotic nephrosis and the severity of renal tubular vacuolization with administration of 6% HES 130/0.4 and cumulative dose of 6% HES 600/0.75, respectively [131,149]. Based on the present evidence [126–134,149], the use of HES in dogs and cats poses a limited risk for AKI when HESs are administered at a relatively low dose and for shorter periods of time. The possibility of renal dysfunction may exist, especially in critically ill patients with pre‐existing renal compromise, warranting renal function monitoring (urine output, creatinine, BUN, etc.) and use of minimal amounts of synthetic colloids in such scenarios. Also, hyperoncotic preparations seem to potentiate this issue so it might be advisable to use iso‐oncotic preparations of synthetic colloids when possible [90,140,141]. The objective of perioperative fluid therapy is to maintain circulatory volume so that end‐organ perfusion and oxygen delivery are not compromised. The traditional approach to achieving this goal entails administration of replacement crystalloids at a dose rate that could vary between 5 and 20 mL/kg/h [13,14]. This high rate of fluid administration was expected to offset the transient intraoperative hypovolemia due to fluid deprivation during preoperative fasting, vasodilation associated with anesthetic drugs, third spacing of intravascular fluids, insensible fluid losses, and intraoperative fluid or blood loss [150,151]. With emerging evidence, most of these physiologic theories are being reexamined. Present perioperative patient management does not advocate prolonged fasting times [152], with patients usually having access to water up until the time of surgery. Additionally, blood volume has been shown to stay normal even after prolonged fasting [153]. The concept of third spacing of fluids during surgery [154] and fluid loading of normovolemic patients to prevent intraoperative hypotension [155] has also been questioned. Stress associated with surgery and anesthesia leads to neuroendocrine responses mediated by stimulation of the hypothalamic–pituitary–adrenal axis, activation of the renin–angiotensin–aldosterone system, and atrial natriuretic peptide and vasopressin release. All of these compensatory mechanisms can alter fluid dynamics independent of renal function [156]. In dogs, antidiuretic hormone concentration increased [157] and urine production decreased after morphine premedication [158], but did not change during anesthesia and surgery when patients received conventional fluid therapy [159] or increased crystalloid fluid administration [7]. Anesthesia‐ and surgery‐related physiological effects cease or decrease at the end of surgery, possibly resulting in a patient who has been given a relatively large quantity of fluids. Postoperatively, such patients end up gaining body weight [159,160]. This positive fluid balance and weight gain mostly occurs in the ECF and has been shown to be associated with a host of abnormalities including increased lung water, decreased pulmonary function, increased infection rate, reduced gut motility, decreased PCV, decreased TP, and a decrease in body temperature eventually translating into increased morbidity and mortality [160–163]. The Confidential Enquiry into Perioperative Small Animal Fatalities (CEPSAF) revealed that cats receiving fluids (likely not goal‐directed dosing) were four times more likely to die, and the authors speculated that excessive fluid administration causing fluid overload could be a cause [164]. Restrictive intraoperative fluid administration could improve postoperative pulmonary function and hypoxemia [165–167]. What constitutes restrictive or liberal fluid therapy is still a question of debate because different randomized controlled trials in humans have used different fluid regimens [168]. Evidence from human anesthesia suggests that low‐risk patients undergoing low‐to‐moderate‐risk surgery benefit from liberal intraoperative fluid therapy (20–40 mL/kg total dose) resulting in reduced postoperative complications like dizziness, pain, nausea, vomiting, drowsiness, and reduced hospital length of stay [167,169,170]. Restrictive fluid therapy in humans was shown to be beneficial in high‐risk patients undergoing major abdominal surgeries [171,172] and was not associated with renal function compromise [166], but findings from a recently published large prospective randomized controlled trial on perioperative fluid therapy in humans were somewhat contradictory. The Restrictive versus Liberal Fluid Therapy in Major Abdominal Surgery (RELIEF) trial conducted in 2983 patients undergoing major abdominal surgery revealed a significantly higher incidence of acute renal injury (8.6% versus 5.0%), need for renal replacement therapy (0.9% versus 0.3%), and surgical site infection (16.5% versus 13.6%) when a restrictive fluid therapy regimen to achieve zero net fluid balance was used intraoperatively and for 24 h postoperatively [173]. Overall, median fluid volume received intraoperatively and in the first 24 h postoperatively in the RELIEF trial was ~6 mL/kg/h and ~1 mL/kg/h in the restricted group and ~11 mL/kg/h and ~1.5 mL/kg/h in the liberal group, respectively. No impact on systolic blood pressure was noticed in dogs receiving fluid rates between 0 and 15 mL/kg/h [174], and increasing fluid rates from 0 to 30 mL/kg/h did not cause any change in urine production or oxygen delivery under anesthesia [7]. Though not based on any specific clinical evidence nor accepted universally, the AAHA/AAFP fluid therapy guidelines in dogs and cats [175] have suggested initially starting fluid administration at 3 mL/kg/h in cats and 5 mL/kg/h in dogs. In short procedures, minimal fluid volume will be administered with these suggested rates, so it is important to closely assess the patient’s hydration and volume status as well as total fluid volume delivered. As measurement of ICF and ISF is not easily accomplished, estimation of IVF volume status is typically undertaken in clinical practice. There is no single variable that can assess the intravascular volume status, which creates some difficulties for application of goal‐directed fluid therapy. Furthermore, it is important to do serial evaluations of a patient to optimize volume status. Clinical examination (mucous membrane color, capillary refill time, pulse rate and quality, temperature of extremities, mentation, skin turgor, moistness of mucous membranes, peripheral edema, thoracic auscultation for crackles, papilledema, respiration rate, pulse oximetry, blood pressure, urine output, and serial body weights), invasive hemodynamic monitoring to obtain objective data (direct blood pressure monitoring, CO monitoring, cardiac filling pressures including central venous pressure [CVP] and pulmonary artery occlusion pressure, pulse pressure variation [PPV] and stroke volume variation [SVV], and transesophageal echocardiography), and laboratory data (arterial and venous blood gases, electrolyte status, and renal function evaluation) all should be used in conjunction to estimate the volume status. Information on various macrovascular (CVP, arterial blood pressure, and urine output) and microvascular (lactate concentration and clearance, base excess, and mixed or central venous oxygen saturation) perfusion parameters help dictate the state of critical oxygen delivery to the tissues [176,177]. Early goal‐directed therapy to normalize some of these endpoints has been shown to improve outcomes [178]. Therapy with synthetic colloids should ideally be monitored using colloid osmometry to measure COP rather than relying on the refractometric analysis because the relationship between COP and refractive index may be different for plasma proteins versus synthetic colloid [179]. There is a poor correlation between the changes in COP and TP as measured by a refractometer after administration of a high‐MW HES formulation (hetastarch) and dextran 70. Both of these synthetic colloids push the refractometric reading toward 4.5 g/dL [180]. Recently published studies in dogs showed that low‐MW 6% HES 130/0.4 formulation (VetStarch®) and 4% succinylated gelatin can also interfere with refractometric measurement of total plasma protein, pushing its reading toward 4.3 g/dL, and this interference could last up to 3 h [181,182]. Refractometric total solids (TS) readings could decrease after colloid administration due to dilutional effects, and this could be falsely interpreted as a need for additional colloidal support, leading to fluid overload [95]. The eventual goal of fluid administration is to improve venous return resulting in an improvement in stroke volume (SV). Venous return depends on the gradient between mean circulatory filling pressure (MCFP) and right atrial pressure and the resistance to blood flow in venous circulation. Physiologically, about 70% of the blood volume is unstressed (volume of blood in the vasculature at zero transmural pressure) and the remainder is stressed (volume of blood that must be removed from the vasculature to drop transmural pressure to zero from existing values) [183]. The stressed volume is a major determinant of venous return and is responsible for generating blood flow by increasing transmural pressure across blood vessels resulting in increased MCFP [184,185]. An unpredictable distribution of administered fluids between stressed and unstressed volumes may in part be responsible for fluid unresponsiveness in certain patients as only about 50% of critically ill human patients respond to fluid challenge with a significant increase in SV [186,187]. It is important to recognize patients that are not fluid responsive (non‐responders) to avoid ineffective or deleterious fluid overloading [188]. Although no standard fluid therapy guidelines have been devised as yet, an individualized fluid therapy approach called “individualized goal‐directed therapy” based on objective feedback from a patient’s fluid responsiveness has been shown to improve outcomes [189–192]. At the core of fluid responsiveness is the Frank–Starling curve [193,194]. In the case of responders, the heart is operating on the ascending part of this curve and they respond with a 10–15% increase in their SV or CO when a fluid bolus is administered to augment the preload [195,196]. The Marik–Philips curve illustrates the relationship between preload and extravascular lung water (EVLW) [197]. Non‐responders operate on the flat part of the Frank–Starling curve resulting in minimal improvement in SV, but, at the same time, the administered fluid results in an increase in EVLW and tissue edema. These curves are influenced by changes in patient physiology imposed by various diseases that necessitate an individualized approach to fluid therapy. Although considered simultaneously, it is important to appreciate the distinction between volume status and fluid responsiveness since a patient that is fluid responsive may not be hypovolemic and vice versa due to changes in vascular permeability, capillary leakage, changes in cardiac function, vascular tone, and complex local and systemic implications of EG damage. Assessment of fluid responsiveness can minimize unnecessary fluid administration, especially in patients predisposed to fluid overload, and may aid in ascertaining the need for inotropic and/or vasopressor therapy. Both static and dynamic indices of preload responsiveness have been described. A parameter is considered static if it is measured at a single time point, whereas a dynamic parameter is measured in real time in response to a change in preload. Based on the Frank–Starling curve, an increase in SV may be expected with fluid administration if the preload is low. This is why the markers of cardiac preload were proposed to predict fluid responsiveness. However, studies have shown that CVP, pulmonary capillary occlusion pressure, and end‐diastolic dimensions of the ventricle are unreliable and generally late indicators of fluid overload, alterations in blood volume, and fluid responsiveness [194,198]. CVP is a poor indicator of right atrial pressure, cardiac filling pressure, and preload changes because the pressure and volume relationship of the ventricle is not linear and CVP is affected by lung, cardiac, and intrathoracic pressures [199,200]. In dogs, it was also shown that CVP performs poorly compared to dynamic indices to evaluate fluid responsiveness [201,202]. The filling pressures may still be helpful to evaluate volume status of the patient such that a low value may justify fluid administration and a high value should prompt stopping or reducing the fluid administration. Originally, pulmonary artery catheterization (PAC) was used to guide optimal oxygen delivery in high‐risk surgical patients but, due to morbidity and mortality increases associated with the use of the PAC itself, and failure to show any clear benefit, it fell out of favor [203–205]. The position of cardiac performance on the Frank–Starling curve is best understood by performing functional hemodynamic testing or the use of heart–lung interactions in mechanically ventilated patients by evaluating respiratory variation in various dynamic indices of preload responsiveness [195,196,206–210]. Functional hemodynamic testing involves administration of an intravenous fluid bolus or performance of a passive leg raise maneuver to shift volume into the central circulation and augment preload [195,196,211,212]. It is desirable to use as small a fluid bolus as possible to assess fluid responsiveness to further minimize the potential for fluid overload. A meta‐analysis in humans revealed that a mini‐fluid challenge can successfully predict fluid responsiveness [213]. A mini‐fluid bolus approach has been reported in dogs using 3–5 mL/kg LRS or Hartmann’s solution administered over 1–5 min [213–217], but the use of 10–20 mL/kg LRS bolus over 5–15 min was reported most often [202,218–222]. Colloids have also been used for fluid challenges in humans [223], and this has been reported in one dog study that used 10 mL/kg of 6% HES 130/0.4 as a fluid bolus that was administered over 13 min [201]. Various veterinary studies have reported a fluid responsiveness rate anywhere from 38% to 100% in healthy and normovolemic dogs under anesthesia [214,201,217,219,221, 222], in dogs undergoing anesthesia for orthopedic or oncologic surgery [220,218], or dogs undergoing abdominal surgery [202]. The variation in fluid responsiveness could be the result of different patient populations, size of the fluid bolus, rate of bolus administration, and modality used to evaluate the change in CO or SV [223,224]. A change in arterial blood pressure is commonly used in veterinary clinical settings as a surrogate for changes in SV or CO, but it is important to appreciate the limitations of this assumption as alterations in SVR may have significant impacts on arterial blood pressure in hypotensive and/or hypovolemic patients [224,225]. Studies in spontaneously breathing anesthetized dogs have demonstrated the inability of mean arterial pressure to discriminate between fluid responders and non‐responders in spite of an increase in CO or SV [201,202,216,220–222]. Various modalities to measure CO have been utilized in anesthetized dogs to evaluate fluid responsiveness including transpulmonary thermodilution [219,221,222], transesophageal echocardiography [218,220], transthoracic echocardiography [201,214–216], uncalibrated pulse contour analysis (PCA) [217], and esophageal Doppler [202]. Many minimally invasive and non‐invasive flow monitoring modalities provide real‐time, continuous, and beat‐by‐beat SV information that is particularly useful when response to a fluid challenge needs to be evaluated. These modalities hold the potential for clinical utility in veterinary medicine, but they should be thoroughly evaluated for their agreement and trending ability with a reference method to measure SV and CO [226,227]. An uncalibrated PCA method does not require any calibration with a reference method, but its performance may suffer during hemodynamic instability and when significant changes in SVR occur [228]. The pressure recording analytical method (PRAM) (incorporated into Most Care Up®) is an uncalibrated PCA method that showed good precision and trending ability with bolus pulmonary artery thermodilution (PATD) CO measurement in dogs under stable circulatory conditions [229] and has been recently used to measure a response to a small volume fluid bolus in dogs [217]. However, in dynamic circulatory conditions created by a hemorrhagic shock dog model [230], and use of various sympathomimetics [231], PRAM revealed a high bias, low precision, and low concordance when compared with bolus PATD CO measurement. Another uncalibrated PCA device (FloTrac/Vigileo®) was found unsuitable to monitor CO in dogs when it was compared with indicator dilution techniques [232,233]. Other non‐invasive methods to measure CO, like electrical velocimetry [234] and pulse wave transit time [235], have also been evaluated in dogs. Different variables related to aortic blood flow have been evaluated in dogs using Doppler technology that could yield information about CO and have been studied in the context of fluid responsiveness [214,215,221,236]. In anesthetized dogs, a > 14.7% change in the velocity time integral (VTI) after a fluid challenge as measured by transthoracic echocardiography tracked closely to the change in SV (> 15%) measured by transpulmonary thermodilution, suggesting that change in the aortic VTI may serve as a surrogate marker for change in SV to predict fluid responsiveness [221]. Another study in conscious dogs with a variety of clinical conditions revealed that an aortic flow VTI of ≤ 10.3 cm showed a sensitivity of 84.6% and a specificity of 100% to predict fluid responsiveness [215]. Although CO obtained from an esophageal Doppler monitor did not show good agreement with bolus PATD CO [237], an abstract in dogs reported a correlation coefficient of 0.9 between the bolus PATD SV and stroke distance (SD) (i.e., the VTI obtained from the descending aorta by the esophageal Doppler) in a hemorrhagic dog model, suggesting that changes in SD may serve as a surrogate marker of changes in SV induced by fluid challenge [236]. Furthermore, the SD variation increased with hemorrhage and decreased when the blood was retransfused. The same abstract showed an increase in descending aortic peak velocity index with hemorrhage and a decrease when the blood was retransfused. Two more studies in dogs revealed significantly higher aortic peak velocity in responders compared to non‐responders with one study showing an area under the receiver operating characteristic curve of 0.95 for prediction of fluid responsiveness, suggesting that changes in aortic blood flow peak velocity could provide information about fluid responsiveness [214,215]. Heart–lung interactions are a dynamic index of volume responsiveness that do not require fluid administration and rely on respiratory variation in preload caused by cyclic alterations in pleural and transpulmonary pressure during mechanical ventilation [202,206–210,238,239]. Reduction in venous return during inspiration with positive‐pressure ventilation is the major determinant of the variation in these dynamic indices. According to the Frank–Starling principle, diastolic filling of the ventricles determines the myocardial stretch and hence SV as related to myocardial contractility. In hypovolemic states, the variation in dynamic indices is high, and the left ventricular contractility depends on the preload to a greater extent. In other words, the ventricle operates on the ascending limb of the Frank–Starling curve. As the volume expands, the left ventricular function shifts in the rightward direction on the Frank–Starling curve and causes an observed decrease in dynamic indices variation. Preload changes result in stroke volume variation (SVV) during inspiration and expiration. In addition, it has been shown that pulse pressure variation (PPV) and systolic pressure variation (SPV) can be used as surrogate markers of SVV and could be easily employed in clinical settings where arterial catheters are placed and patients are ventilated mechanically [202,207–210]. The plethysmographic variability index (PVI) is a non‐invasive dynamic index of preload responsiveness that evaluates changes in perfusion index as obtained by a pulse oximeter (Masimo™ Radical‐7®) [202]. Various canine studies have suggested that SVV > 11–14.7% [217,239], PPV > 7–16% [201,202,217,219,220,240], SPV > 4.1–7.4 mmHg [217,220], PVI > 11–14% [201,202,217,241], and caudal vena cava distensibility index of 24% [214] predict fluid responsiveness with good sensitivity and specificity. A few human and canine studies have suggested PPV to be a better index of fluid responsiveness compared to SPV and PVI [202,208,217]. An abbreviated echocardiogram (“cardiac point‐of‐care ultrasound”) is also being used increasingly due to its rapid, non‐invasive, and real‐time ability to evaluate patient volume status [242]. Estimation of volume status mostly focuses on the subjective assessment of atrial and ventricular lumen size, ventricular wall thickness, and cardiac contractility, all of which require minimal training to learn [243,244]. Studies performed in dogs and cats revealed these changes to be proportional to the severity of hypovolemia [245]. The difference in cutoff values, sensitivity, and specificity of various dynamic indices among different studies is a reflection of a lack of standardization of the volume and rate of fluid administration and ventilatory parameters, including tidal volume, peak inspiratory pressure, and positive end‐expiratory pressure, and is also affected by patient–ventilator asynchrony, changes in respiratory system compliance, changes in vascular tone, arrhythmias, right heart failure, and intra‐abdominal hypertension [223,246–251]. The proportion of responders to fluid bolus has not been shown to be influenced by the type of fluid [223]. In clinical veterinary settings, tidal volumes are often altered, patients may buck the ventilator, many patients undergo minimally invasive laparoscopic procedures coupled with increased intra‐abdominal pressure, and critically ill patients may have cardiac arrhythmias or be exposed to vasoactive drugs to support arterial blood pressure. In order to improve the utility of published thresholds for dynamic indices, it is important to understand the context of the published study and the clinical scenario in which the dynamic index will be employed. One should refrain from interpretation of these tests in isolation since they are not 100% sensitive and specific. Every patient has an individual set of Frank–Starling and Marik–Philips curves that are influenced by various physiological and pathological factors. Understanding of the individual’s physiology should allow clinicians to estimate the impact of fluid administration in patients but obviously without absolute certainty. Also, it is important to understand that fluid responsiveness does not equate to fluid requirement unless there is clear cut evidence of benefit to a patient’s hemodynamic status as well as a lack of associated risks and various confounding factors [252–255]. Administration of fluids to the point of lack of fluid responsiveness could lead to fluid overload and hemodilution that could be detrimental to the patient [255]. Arterioles, capillaries, and venules are the structural components of the microcirculation and are the conduit of oxygen and nutrient delivery to the tissues. As described above, the endothelial glycocalyx (EG) is a protective layer of carbohydrate scaffold that coats the luminal surface of endothelium and plays a vital role in cell signaling and transvascular permeability [4,5,256]. The EG is composed of proteoglycans, glycosaminoglycans, and glycoproteins. Together with the mobile or soluble protein components, including albumin and antithrombin, it constitutes the ESL. The ESL regulates vascular permeability and controls local inflammation. Its system‐wide degradation has been associated with severity of illness, increased vascular permeability, and exacerbated systemic inflammation via soluble heparan sulfate and low‐MW hyaluronan that are shed into the circulation after EG damage [257–258]. Though clinicians rely heavily on the measurement of macrocirculatory perfusion parameters like CO and arterial blood pressure, it has been demonstrated that despite their normalization, heterogeneity in capillary blood flow could still persist [259,260]. There is evidence that ESL degradation can alter microcirculation resulting in reduced capillary blood flow [261]. EG biomarkers are components of the ESL that are released into circulation after degradation and data supports an association between the concentration of biomarkers like hyaluronan, syndecan, and heparan sulfate and the volume of fluid administered (that is, excessive fluid therapy itself degrades the ESL). Inflammation and shock can result in ESL degradation even before any fluid therapy is administered via proinflammatory cytokines like tumor necrosis factor‐α, matrix metalloproteinases released by activated leukocytes, ischemia and reperfusion, reactive oxygen species, and bacterial components like lipopolysaccharides. Hemodilution caused by crystalloids leads to a reduction in ESL thickness and an increase in vascular permeability. In addition, bolus fluid therapy results in degradation of the EG and increased vascular permeability due to release of atrial and brain natriuretic peptides (ANP and BNP) from the atria and ventricles during myocardial stretch [262,263]. Fluid loading in human surgical patients resulted in a parallel increase in ANP and EG biomarkers syndecan‐1 and hyaluronan in serum [264]. A rapid volume expansion with a balanced isotonic crystalloid in a canine hemorrhagic model, however, did not show an increase in ANP but did reveal an increase in hyaluronan concentration and enhanced inflammatory response indicated by increased plasma concentrations of interleukin‐6 and interleukin‐10 [265]. Although the clinical relevance of ESL degradation with fluid therapy is not yet clear, it may promote microcirculatory dysfunction, inflammation, and coagulation. It is therefore advisable to avoid excessive hemodilution and hypervolemia. Careful fluid titration should be considered in septic patients suffering from vasodilation and microcirculatory dysfunction due to lack of hemodynamic coherence between the macro‐ and microcirculations [259,266,267]. At the same time, caution is advised in restricting fluid volume in non‐septic patients as a recent randomized controlled trial revealed a higher rate of AKI and surgical site infection in patients receiving restrictive fluid therapy [173]. There is also some evidence that rapid fluid administration may result in more heterogeneity in microcirculatory blood flow and higher inflammatory cytokines due to amplified EG degradation; slower fluid administration may help achieve similar endpoints without deleterious effects on the EG [268,269]. The question about the type of fluid (crystalloids or colloids) to be used for volume expansion has long been debated. The relatively higher efficacy of colloids to restore intravascular volume has been shown in patients without systemic disease [270]. However, more recent clinical studies in systemically ill people have failed to support these findings and suggested a colloid‐to‐crystalloid volume expansion ratio of 1:1 to 1:1.5 [271]. Damage to the EG caused by systemic illness results in fluid extravasation of both colloids and crystalloids resulting in loss of the volume efficacy effect of colloids [272,273]. In addition, the duration of the volume expansion by colloids is reduced under systemic inflammatory conditions due to increased vascular permeability. Although there is some evidence that colloids may be associated with less ESL degradation [274–276], altering the fluid choice to optimize microcirculation based on its potential beneficial or detrimental impact on ESL is premature. In the absence of any clear‐cut benefit on mortality, the human literature does not advocate the use of one over another type of fluid [277]. Sodium is the most abundant cation in the ECF (see Table 32.1). Embedded in the cell membrane, Na+‐K+ ATPase maintains a relatively low intracellular concentration of Na+. The concentration of Na+ in the serum is a reflection of the amount of Na+ relative to total ECF and does not provide any indication about the content of total body sodium. Disorders of sodium are mostly the result of changes in the amount of body water so changes in Na+ concentration should be viewed as changes in free water content of the body. In response to changes in intravascular volume, antidiuretic hormone (ADH), signals from baroreceptors and osmoreceptors, thirst mechanisms, the renin–angiotensin–aldosterone (RAAS), and ANP all act accordingly to promote homeostasis of volume and tonicity of body fluids via regulation of Na+ and water balance [278]. Hypernatremia could result from hypotonic dehydration (resulting in hypovolemic hypernatremia), a deficit of free water (resulting in normovolemic hypernatremia), or ingestion or iatrogenic administration of high sodium‐containing fluids (resulting in hypervolemia) [279]. Hypernatremia results in cellular dehydration (especially of brain cells) as water moves from the ICF to the ECF. Shrinking of brain tissue can cause tearing of blood vessels, resulting in subarachnoid and intracerebral hemorrhage [278]. The pathophysiological consequences of hypernatremia are determined mainly by the rapidity of onset of hypernatremia rather than the absolute value. Under chronic circumstances (> 2–3 days), brain cells increase intracellular osmolality by accumulation of osmotically active solutes called “idiogenic osmoles” [280,281]. Lien et al. [281] found that hypernatremia in rats increased concentrations of myoinositol, bataine, glycerophosphorylcholine, phosphocreatine, glutamine, glutamate, and taurine in the brain. Idiogenic osmoles help maintain intracellular volume and prevent efflux of fluid. Hence, in chronic hypernatremia, rapid correction of the sodium level could decrease extracellular osmolality and favor the movement of water into brain cells, causing cerebral edema. If hypernatremia is of relatively acute onset (< 24 h), rapid correction is better tolerated because idiogenic osmoles are not yet present. Intracranial hemorrhage and neuronal dehydration associated with hypernatremia could result in muscle weakness, lethargy, ataxia, seizure, coma, and death [279]. Treatment should involve correction of the underlying problem and fluid administration to replenish free water content. In hypovolemic hypernatremic patients, intravascular volume deficits should be corrected using a fluid that is isotonic to the patient followed by correction of the free water deficit with fluids like 5% dextrose or 2.5% dextrose in 0.45% saline [282]. The formula given below is used to calculate the free water deficit (FWD):
32
Clinical Pharmacology and Administration of Fluid, Electrolyte, and Blood Component Solutions
Physiology of body fluids
Total body water
Solute composition of various body fluid compartments
Movement of water between intracellular and extracellular fluid compartments
Ions
Extracellular fluid (mEq/L)
Intracellular fluid (mEq/L)
Interstitial fluid
Intravascular fluid (plasma)
Na+
145
142
12
K+
4
5
140
Ca2+
2
2
4
Mg2+
2
2
34
Cl–
112
104
4
HCO3–
27
24
12
HPO42–, H2PO4–
2
2
40
Protein–
1
14
50
Fluid shifts between intravascular (plasma) and interstitial fluid compartments
∆πn represents the sum of the difference in osmotic pressure exerted across the capillary wall by all the solutes in IVF and ISF. In the above equation, ΔP describes the difference between local capillary blood pressure (Pc) and ISF hydrostatic pressure (Pi). In most microvascular beds, the capillary wall is freely permeable to water and other ionic solutes like Na+, K+, and glucose because of their very small σ of ≤ 0.1. In other words, they are ineffective osmoles in this scenario and do not contribute to water distribution between ISF and IVF. Only the macromolecular solutes (like proteins) with high σ, and which are present in significantly different concentrations across the capillary wall, will contribute to generation of osmotic gradients (σΔπ). If πp is the osmotic pressure generated by plasma proteins (COP) in IVF and πi is the osmotic pressure generated by macromolecules in ISF, then the above equation can be written as the conventional expression for Starling’s equation as follows:
Endothelial glycocalyx model of transvascular fluid exchange and modification of Starling’s equation
Different types of fluids used in clinical practice
Crystalloids
Isotonic crystalloids
Fluid (isotonic, replacement)
Electrolytes (mEq/L)
Buffers (mEq/L)
Osmolarity (mOsm/L)
pH
Na+
Cl−
K+
Ca2+
Mg2+
Lactate
Acetate
Gluconate
0.9% NaCl
154
154
0
0
0
0
0
0
308
5.0
Lactated Ringer’s solution
130
109
4
3
0
28
0
0
272
6.5
Plasma‐Lyte®‐148
140
98
5
0
3
0
27
23
294
5.5
Normosol®‐R
140
98
5
0
3
0
27
23
294
6.4
Plasma‐Lyte®‐A
140
98
5
0
3
0
27
23
294
Plasma
142
104
5
5
3
HCO3− = 24
300
7.4
Hypertonic crystalloids
Composition
Hypertonic saline solutions
Normalsaline
Plasma
3%
5%
7.2%
7.5%
23%
0.9%
Na+ (mEq/L)
513
856
1232
1283
4004
154
142
Cl– (mEq/L)
513
856
1232
1283
4004
154
104
Osmolarity (mOsm/L)
1026
1712
1464
2566
8008
308
300
Hypotonic crystalloids
Colloids
Fluid
Electrolytes (mEq/L)
Buffers (mEq/L)
Osmolarity (mOsm/L)
pH
Glucose (g/L)
Na+
Cl–
K+
Ca2+
Mg2+
Lactate
Acetate
Gluconate
0.45% NaCl
77
77
0
0
0
0
0
0
154
5.0
0
0.45% NaCl with 2.5% dextrose
77
77
0
0
0
0
0
0
280
4.5
25
Plasma‐Lyte®‐M with 5% dextrose
40
40
16
5
3
12
12
0
376
5.5
50
Normosol®‐M with 5% dextrose
40
40
13
0
3
0
16
0
364
5.5
50
Dextrose 5%
0
0
0
0
0
0
0
0
252
4.0
50
Plasma
142
104
5
5
3
HCO3− = 24
300
7.4
Synthetic colloids
Hydroxyethyl starches
Type of HES
HES product
Physicochemical properties
Carrier
COP (mmHg)
Osmolarity (mOsm/L)
Plasma volume expansion (%)
Concentration
Weight (kDa)
MS
C2:C6
Molecular
Number average
Rapidly degradable
Tetrastarch (Voluven®, Vetstarch®)
6%
130
70–80
0.4
9:1
Saline
High 30s
308
130
Slowly degradable
Hetastarch (Hextend®)
6%
670
0.75
4–5:1
Balanced
Low 30s
307
100
Hetastarch (Hespan®)
6%
450
69
0.7
4:1
Saline
Low 30s
309
100
Dextrans
Gelatins
Clinical implications of synthetic colloid use in veterinary medicine
Perioperative fluid therapy
Liberal versus restrictive approach
Monitoring fluid balance
Individualized goal‐directed fluid therapy
Improving the utility of dynamic indices of preload responsiveness
Microvasculature and fluid therapy
Electrolytes
Sodium
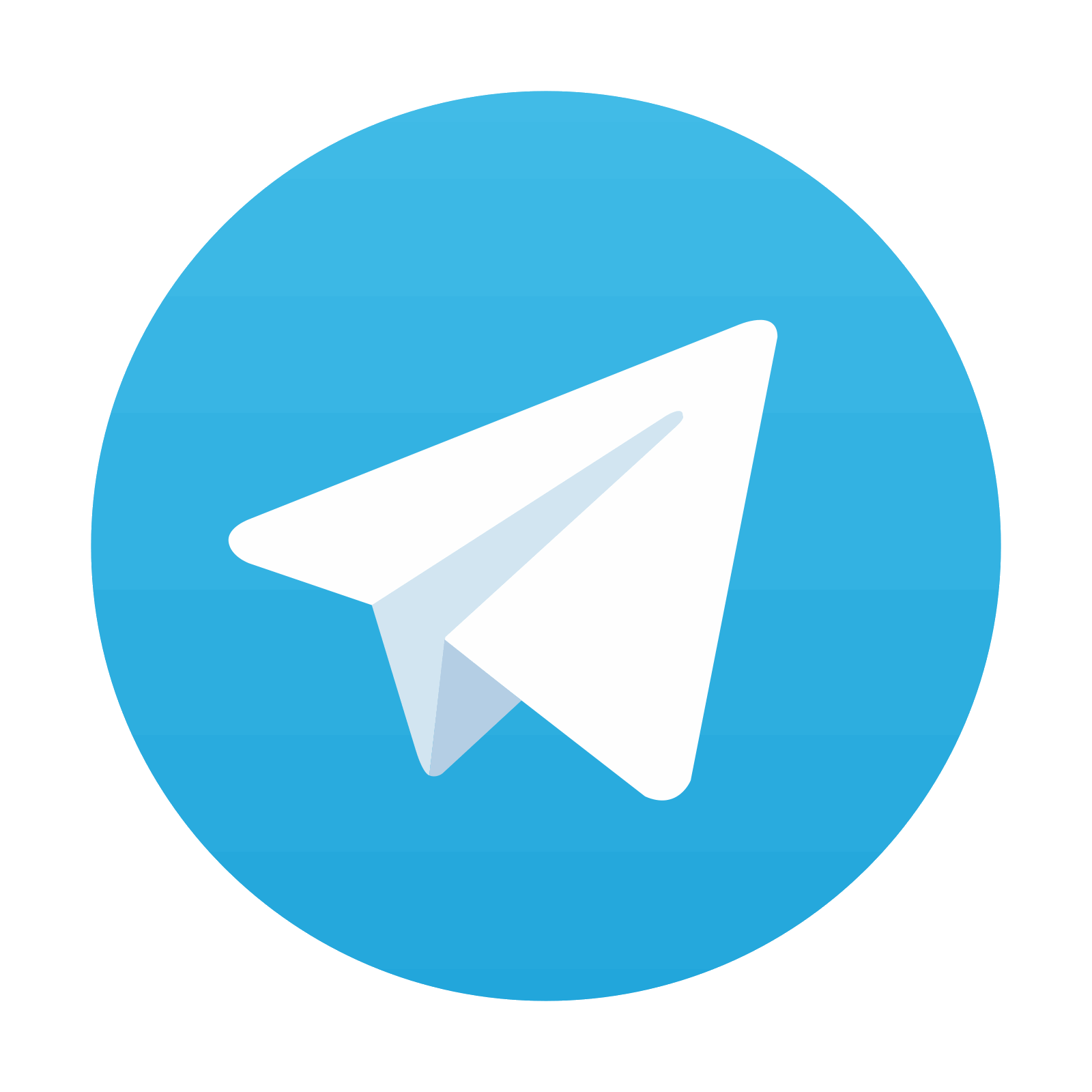
Stay updated, free articles. Join our Telegram channel
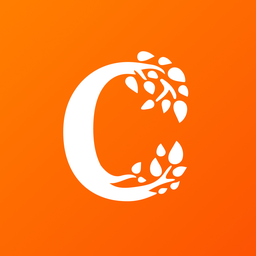
Full access? Get Clinical Tree
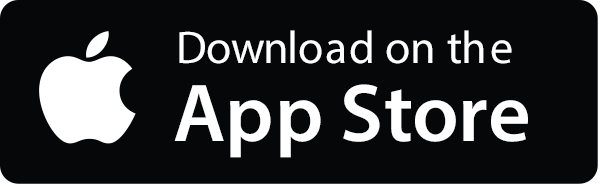
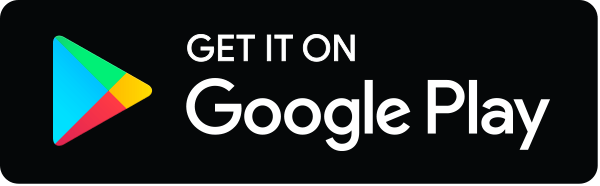