CHAPTER 30 Clinical Chemistry of the Puppy and Kitten
This chapter focuses on the typical development and acquisition of normal biochemical constituents in puppies and kittens and illustrates the many differences between adult and neonatal biochemical parameters. Although published reference ranges are provided (Tables 30-1 to 30-5) based on the available research in current literature, it is recommended that reference intervals be established for each laboratory because of the lack of standardization among reference laboratories. Practitioners, however, may use these ranges as guidelines for interpretation of serum biochemical results in puppies and kittens aged less than 1 year. Hereditary conditions affecting biochemical parameters of young dogs and cats are shown in Table 30-6.
TABLE 30-6 Hereditary conditions affecting biochemical parameters of young dogs and cats
Condition | Mode of Inheritance |
---|---|
Benign familial hyperphosphatasemia in Siberian Huskies | Likely autosomal (exact mode of inheritance not known) |
Musculodystrophy | X-linked |
Hyperchylomicronemia in cats | Believed to be autosomal recessive |
Pancreatic acinar atrophy and exocrine pancreatic insufficiency in German Shepherd Dogs | Autosomal recessive |
Severe combined immunodeficiency | |
Basset Hounds | X-linked |
Jack Russell Terriers and Cardigan Welsh Corgis | Autosomal recessive |
IgA deficiency | Unknown |
Hypercholesterolemia in Rottweilers and Dobermans | Unknown |
Hereditary renal dysplasia in Lhasa Apsos | Autosomal recessive |
Congenital dwarfism | Autosomal recessive |
Hyperkalemia in posthemolysis in Akitas, Japanese Shibas, and Jindos | Believed to be autosomal recessive |
Glucose
Hypoglycemia
Common causes of hypoglycemia in neonates are listed in Box 30-1.
Hyperglycemia
Juvenile or type 1 diabetes mellitus is more common in young dogs, and it has not been well documented in feline medicine. Juvenile diabetes is discussed in more detail elsewhere in this text but should be suspected in puppies with persistent hyperglycemia, glucosuria, and clinical signs of disease (see Chapter 45).
Common causes of hyperglycemia in neonates are listed in Box 30-1.
Protein
Globulins
In puppies, 5% to 10% of maternally derived IgG, and possibly IgM, may be transferred transplacentally. The remainder of IgG is transferred in the colostrum, resulting in an initial spike in globulins immediately after colostral ingestion. Because IgM circulates as a pentamer, it is unlikely that IgM is absorbed through the colostrum because of the large size of the molecule. This is supported by studies showing that puppies given adult canine serum subcutaneously at birth have higher IgM concentrations than puppies given the same serum orally. Additional information pertaining to passive transfer of maternal antibodies can be found in Chapter 2. The half-life of IgG and IgA in puppies is 10 days and 4 to 5 days, respectively. Therefore, a decrease in globulins and an increase in albumin within the first 6 weeks of life are identified as a result of degradation of maternally derived antibodies and increased synthesis of albumin as normal liver function develops. This leads to a peak in the albumin to globulin (A/G) ratio at 6 to 8 weeks, after which the A/G ratio decreases, consistent with a slight increase in globulin fraction likely related to maturation of the immune system.
Hypoproteinemia
An inherited deficiency of B lymphocytes has been reported as an X-linked trait in Basset Hounds and also as an autosomal recessive trait in Cardigan Welsh Corgis and Jack Russell Terriers (see Table 30-6). This syndrome, known as severe combined immunodeficiency syndrome (SCID), results in a marked decrease in immunoglobulin production and occurs with profound lymphopenia in affected puppies. Selective immunoglobulin deficiencies have also been identified in other breeds of puppies, including IgA deficiency in German Shepherd Dogs, Beagles, and Shar-Peis (see Table 30-6).
Causes of hypoproteinemia are listed in Box 30-2.
Lipids
Hypolipidemia
Causes for hypolipidemia are summarized in Box 30-3.
Hyperlipidemia
Primary/congenital hyperlipidemia is described in dogs and cats (see Table 30-6). For example, primary hyperlipidemia secondary to hypercholesterolemia with normotriglyceridemia has been reported in some dog breeds such as Doberman Pinschers and Rottweilers. Fasting hypertriglyceridemia (>5 mmol/L or >454.54 mg/dl) with severe hemolytic anemia may be associated with idiopathic hyperlipidemia in kittens, a congenital condition in cats caused by a defect in lipoprotein lipase, the enzyme necessary for cellular uptake of triglycerides by myocytes and adipose. Elevated chylomicrons and VLDLs with or without low LDL and HDL are also identified in affected kittens. Clinical signs of this syndrome include weakness, inappetence, peripheral neuropathy (hindlimb paralysis), retinal disease (lipemia retinalis), tachycardia, and tachypnea resulting from anemia.
Congenital hypothyroidism with hypercholesterolemia has been reported in puppies and kittens. The mechanism is not completely understood; however, it may be associated with decreased hepatic metabolism of lipids and decreased fecal excretion of cholesterol. This disorder is discussed in more depth in Chapter 45. Some common causes for hyperlipidemia are summarized in Box 30-3.
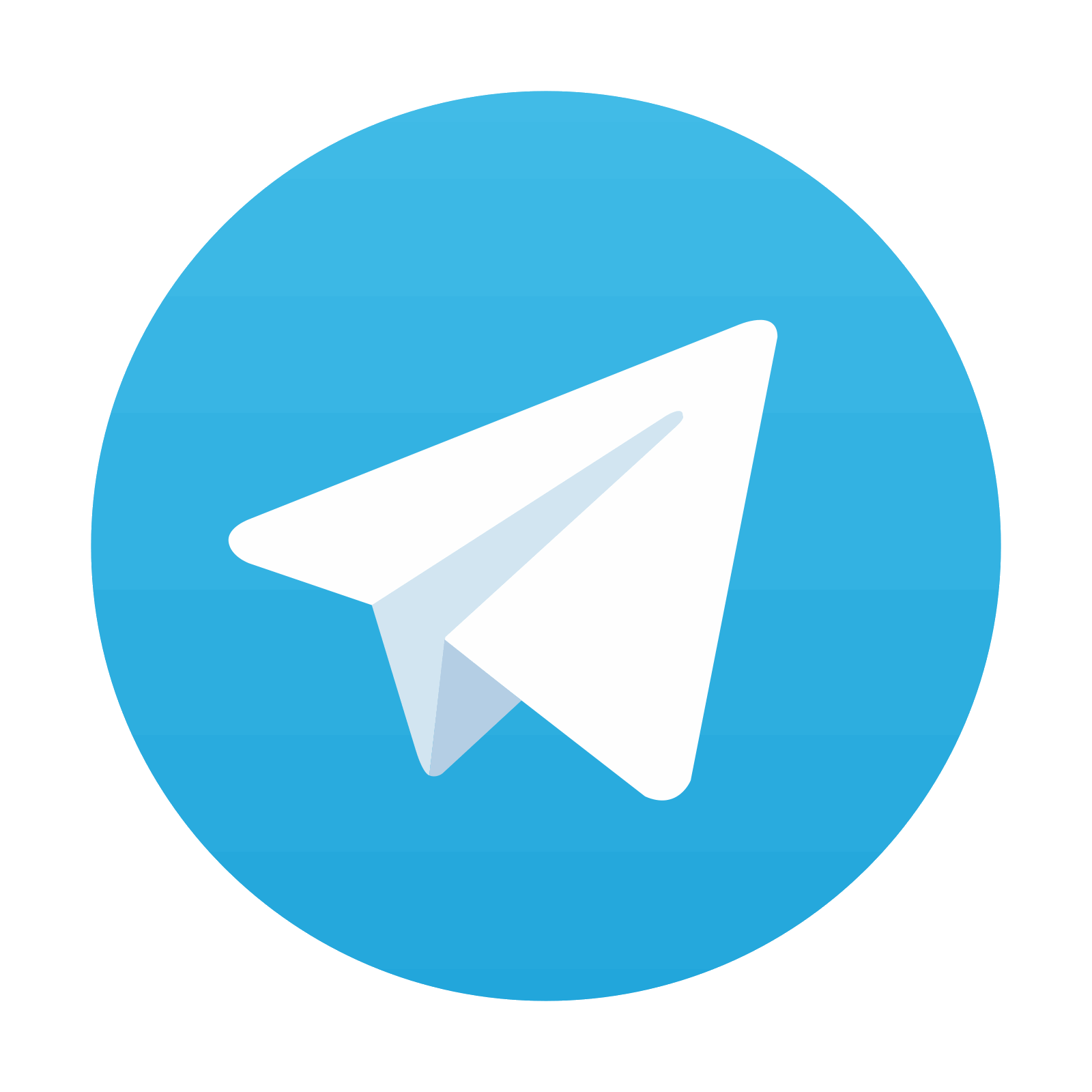
Stay updated, free articles. Join our Telegram channel
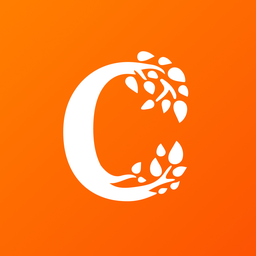
Full access? Get Clinical Tree
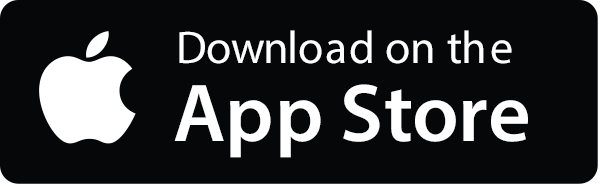
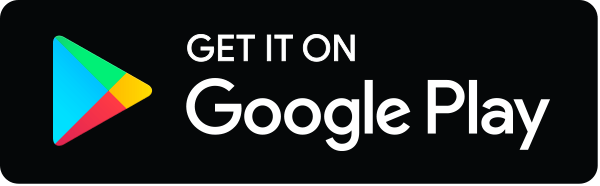