© The Author(s) 2016
Arpan Bhagat, Giorgia Caruso, Maria Micali and Salvatore ParisiFoods of Non-Animal OriginSpringerBriefs in Molecular Science10.1007/978-3-319-25649-8_33. Chemistry and Technology of Ready-to-Eat Vegetable Foods
(1)
Palermo, Italy
(2)
Palermo, Italy
Abstract
Ready-to-eat vegetable food refers to minimally processed fruits and vegetables, which have undergone treatments of mild intensity, without the alteration sensorial characteristics such as freshness. This type of product is ready for consumption: in recent years, it has emerged as a growing reality as it responds to consumers’ needs by offering new services (convenience food). Given the direct consumption, the producer must associate a high quality of the product. This food, normally fresh and without added preservatives, is exposed to chemical and microbiological alterations; as a result, it is surely associated with a reduced shelf life. Even if these products receive some degree of minimal technological processing before market distribution, the used processing technology may be not sufficient, in most cases, with reference to microbiological stability and the complete removal of pathogens. Numerous techniques are currently been used in order to reduce microbiological and chemical spoilage, including chlorine washing, irradiation and modified atmosphere packaging. This chapter concerns recent updates about correlated technologies, including new recyclable trays, and correlated chemical and physical modifications of ready-to-eat packed products: the ‘respiration’ of vegetables, colorimetric modifications and other sensorial alterations.
Keywords
Enzymatic browningEthyleneLactic acid bacteriaMicrobial spoilageModified atmosphere packagingpHReady-to-eat vegetable foodRespirationShelf lifeWater activityAbbreviations
AEW
Acidic electrolysed water
CO2
Carbon dioxide
ClO2
Chlorine dioxide
DNA
Deoxyribonucleic acid
EHEC
Enterohaemorrhagic
HPP
High pressure processing
H2O2
Hydrogen peroxide
LAB
Lactic acid bacteria
MAP
Modified atmosphere packaging
N2
Nitrogen
O2
Oxygen
ppm
Parts per million
POD
Peroxidase
PAL
Phenylalanine ammonia lyase
PPO
Polyphenoloxidase
RTE
Ready-to-eat
RNA
Ribonucleic acid
UV
Ultraviolet light
Aw
Water activity
3.1 Introduction
In recent years, the demand for minimally processed and ready-to-eat (RTE) fresh food products has increased dramatically in developed countries. The main reason is substantially correlated with the offer of a suitable choice for contemporary lifestyles: RTE products provide incorporated services (convenience food) to consumers. Moreover, the awareness of benefits of a diet rich in fruits and vegetables has simultaneously risen with clinical investigations and the epidemiological research. In particular, recent studies have associated the consumption of vegetable foods to a reduced risk of cardiovascular, chronic and neurological diseases, as well as some kinds of cancer (Ragaert et al. 2004; Su and Arab 2006). As a matter of fact, RTE foods contain high levels of micronutrients, fibres and antioxidants, including carotenoids and flavonoids.
Minimally processed vegetable foods are fruits and vegetables which have undergone treatments of mild intensity with the aim of increasing their functionality. On the other hand, these processing techniques do not alter sensorial features, such as freshness, and the expected nutritional quality (Allende et al. 2006). The initial quality of produce before processing has high relevance when speaking of the final RTE product. In fact, vegetables are in a raw state and ready for consumption. Consequently, these foods require very special attention because of their peculiar physiological, enzymatic and respiratory features. In addition, the problem of microbiological risks for consumers’ health has to be considered.
3.2 Shelf Life and Processing
Generally, vegetable foods are known to be among the most perishable edible products. In fact, they display a high water activity (Aw) together with a neutral to slightly acidic pH value and higher carbohydrate contents with respect to proteins (Ramos et al. 2013).
In addition, minimally processed vegetable foods differ from traditional and intact products both for their physiology and their handling and storage requirements. As a matter of fact, processing procedures include very often cutting, slicing, shredding, dicing, peeling, washing and other procedures; these steps can affect the final storage life (Siddiqui et al. 2011). Washing water serves to reduce microbial contaminations because of the presence of sanitising agents such as chlorine (Sect. 3.5.1) and other chemicals (Gurnari 2015a, b).
First of all, some fruits and vegetables require the peeling step because of the necessity of removing inedible parts. Subsequently, chopping operations are required with the aim of facilitating prompt consumption. The disruption of tissues and cells integrity caused by processing can decrease shelf life.
In fact, wounded tissues undergo enhanced deterioration; as a result, derived products have a very short shelf life: 4–7 days, depending on the initial quality, the initial microbial load and the used processing technology (Watada and Qi 1999). However, various factors can influence the extent of disruption and senescence during cutting process: in particular, the size of vegetable pieces, the sharpness of cutting blades and mechanical properties of the product have to be carefully studied (Siddiqui et al. 2011).
3.3 Chemical and Biochemical Mechanisms of Spoilage
Minimally processed fruits and vegetables have different physiological rates if compared with intact products: their metabolism is accelerated similarly to the observed situation of stressed plant tissues. Even minimal processing can lead to an increase in respiration, ethylene production, water loss, microbiological replication, as well as enzymatic browning, formation of volatiles, loss of chlorophyll and lipid oxidation (Toivonen and DeEll 2002). These modifications influence directly the appearance of the final product; unfortunately, the consumer’ approach is first focused on the estimation of appearance, colour and texture (Wismer 2009).
Ethylene has been reported to increase in minimally processed vegetable foods even if this phenomenon is dependent on intrinsic factors (i.e. climacteric vs. non-climacteric produce). Temperature has also an effect on the induction of ethylene production: for instance, it has been found in cantaloupes stored at very low temperatures. In this situation, the suppression wound-induced ethylene has been recognised (Madrid and Cantwell 1993). Generally, ethylene increases ripening, senescence and textural modifications by means of the stimulation of enzymatic activity; enzymes can be peroxidase (POD) and polyphenoloxidase (PPO) as well as phenolic compounds (Saltveit 1999). The initiation of wound ethylene response starts usually within 1 hour; the maximum rate is achieved between 6 to 12 h (Abeles et al. 1992).
In turn, ethylene stimulates the respiration rate: consequently, a notable enhancement of the tricarboxylic acid cycle, the electron transport chain and starch breakdown can be observed. In fact, post-harvest vegetables are living tissues similar to normal vegetables; therefore, these tissues utilise reserve energy during ageing. For instance, respiration rates have been reported to increase in baby carrots by two–threefolds after peeling and slicing (Simõ et al. 2011). In agreement, tissues with high respiration rates and low energy reserve have a shorter shelf life (Eskin 1990). However, the augmented respiration is not only due to the enhancement of aerobic respiration: the role of α-oxidation of long-chain fatty acids with the production of carbon dioxide (CO2) has been also proposed as synergic cause (Rolle and Chism 1987).
Moreover, minimally processed products are more susceptible to water loss because peeling and cutting operations expose interior tissues. As a consequence, the peridermal tissue—which acts as a protection against excessive transpiration—is removed and surface-to-volume ratios are forced to increase (Toivonen and DeEll 2002). The decrease in water leads to a loss of turgor, reducing the firmness of the products and hence the consumer’s acceptance.
Another factor correlated with the consumer’s evaluation of vegetable foods is enzymatic browning. This phenomenon is primarily caused by
(a)
Cell disruption, which activates metabolic pathways, ultimately leading to the synthesis of enzymes and substrates, and by
(b)
Loss of cellular compartmentation, which brings cell units together.
Phenylalanine ammonia lyase (PAL) is one of the key enzymes in phenylpropanoid metabolism and is wound induced. As a matter of fact, PAL produces various phenolic compounds, which are then oxidised in reactions involving POD and PPO (Barry-Ryan and O’Beirne 1998). POD, widespread in plant cells, is iron-porphyrin organic catalyst with a notable role in browning through two possible routes. The first of these mechanisms involves the formation of hydrogen peroxide (H2O2) during the oxidation of phenolic compounds, whereas the second reaction route utilises quinonic forms as substrates (Richard-Forget and Gauillard 1997).
PPO is a tetramer that contains four atoms of copper per molecule and catalyses the hydroxylation of monophenols to o-diphenols. PPO can also further catalyse the oxidation of o-diphenols with the consequent production of o-quinones. As a result, quinones can react with non-enzymatic reactions with other quinones, amino acids or proteins. The result is a melanin pigment, responsible for the well-known black to brown colour. Another enzyme involved in senescence is lipoxygenase, an iron-containing enzyme that catalyses the oxidation of polyunsaturated fatty acids in lipids containing a cis–cis-1,4-pentadiene structure (Lamikanra 2002). Therefore, lipoxygenase generates free radicals with the ability of provoking further membrane rupture; the structural lipidic membrane is degraded. In addition, lipoxygenase is responsible for production of certain volatiles: involved biochemical pathways are usually triggered by cell damage.
As a matter of fact, plants produce secondary metabolites in response to wounding: these secondary compounds may affect dramatically the perceived odour. Each vegetable species is believed to synthesise its own characteristic volatile pattern (Pichersky et al. 2006), even if phenylpropanoid and polyketide phenolics, aldehydes, alcohols and terpenoids are the main compounds. Sulphur-containing compounds may also accumulate during time as a result of the loss of cellular compartmentation. Enzymes such as cysteine sulfoxide lyase can oxidise various substrates and convert these compounds into sulphur-containing molecules which may be responsible for off-odours. Peculiar examples can be methanethiol, dimethyl disulfide and allyl isothiocyanate in cut cabbage tissues (Chin and Lindsay 1993; Dan et al. 1997).
Furthermore, discoloration can also occur with a general loss of green colour, due to chlorophyll degradation. Two enzymes are considered responsible for chlorophyll breakdown: chlorophyllase and magnesium dechelatase. Two alternative alternative pathways have been reported at present, both resulting in the formation of a common product: pheophorbide a, an olive-brown compound, which is the precursor of the colourless product in a reaction mediated by pheophorbide a oxygenase (Toivonen and Brummell 2008).
Finally, the residential microbial flora also affects the quality of vegetable products through spoilage and/or with possible risks for consumers’ health. Processing operations can provide further opportunities for microbial contaminations; in addition, they can also cause leaking of small molecular weight compounds and cellular fluids from damaged tissues. In fact, microbial growth is usually higher in fresh-cut products with respect to the whole product. As a result, spoilage may occur: peculiar signs are loss of texture, brown colours, production of off-odours and soft rot.
3.4 Microbiological Quality
Vegetable food possess a natural saprophyte microflora deriving from soil, water, insects and consisting of bacteria, yeasts, moulds that find favourable pH and Aw conditions. As a consequence, microbial flora tends to increase during all post-harvesting stages.
The number and species of microorganisms can vary depending on the type of produce and growing conditions; however, normal counts usually range from 103 to 109 colony forming units/g, with a general predominance of Gram-negative bacteria in vegetables, and of yeasts and moulds in fruits (Oliveira et al. 2010). Even biofilms may occur in vegetable leaves, mainly composed of environmental species which may act either preventing adhesion to plant surfaces by other bacteria. Alternatively, pathogens may be embedded in their matrix, hence decreasing the efficacy of sanitising treatments.
The dominant microflora in vegetables is composed of Pseudomonas, generally up to 50–90 % (Arvanitoyannis and Stratakos 2010). The most abundant species appear to be Pseudomonas fluorescens, P. putida and P. cepacia, whose role as spoilage microorganisms is notable. As a matter of fact, they can synthetise enzymes—also under refrigeration conditions—such as pectinases, cellulases, glycoside hydrolases and lipoxygenase, in addition to well-recognised proteolytic and lipolytic activities (Heard 2002). Pectic substances are very abundant in vegetable cell walls. Chemically, these compounds are linear chains of α-(1–4)-linked d-galacturonic acid, with carboxyl groups either esterified (pectin) or non-esterified (pectic acid) with methanol.
Pectic substances are used by many microorganisms as energy source, resulting in enzymatic liquefaction of these compounds and consequently in tissue softening (Chen 2002). Involved enzymes are pectinases: these compounds exist in a wide variety of forms and are classified according to the reaction. In detail, should the mechanism of action involve β-elimination or hydrolysis, two categories would be considered: pectinesterases and depolymerising enzymes. Pectinesterases catalyse a de-esterification reaction of pectin resulting in pectate and methanol, whereas the second type of enzymes is able to cleave the pectinic chain, thereby releasing shorter portions (Sakai et al. 1993).
A peculiar enzyme, cellulose, catalyses the decomposition of cellulose, specifically by hydrolysis of the 1, 4-β-d-glucosidic bond. Basically, cellulases break down the cellulose molecule into monosaccharides such as glucose, or shorter chain of oligosaccharides. These enzymes are used by bacteria with the aim of obtaining short soluble sugars as food resources: they are divided into three general major types, based on the type of catalysed reaction:
Endocellulases, which cleave internal bonds at random sites, thus creating new chain ends
Exocellulases or cellobiohydrolases, which cleave two to four monomers from one end of the chain, producing cellobiose and/or glucose
Cellobiases or β–glucosidases, which can hydrolyse exocellulase products into single monosaccharides (Singh and Hayashi 1995).
Enterobacteriaceae are well represented: generally, the most reported life forms are Enterobacter, Pantoea and Serratia. With the notable exception of Erwinia carotovora, a well-known plant pathogen, these bacteria are environmental microbes, encompassing a wide variety of ecological niches (Caponigro et al. 2010). Their role in the spoilage process has not been so well examined until now: consequently, more research would be needed at present.
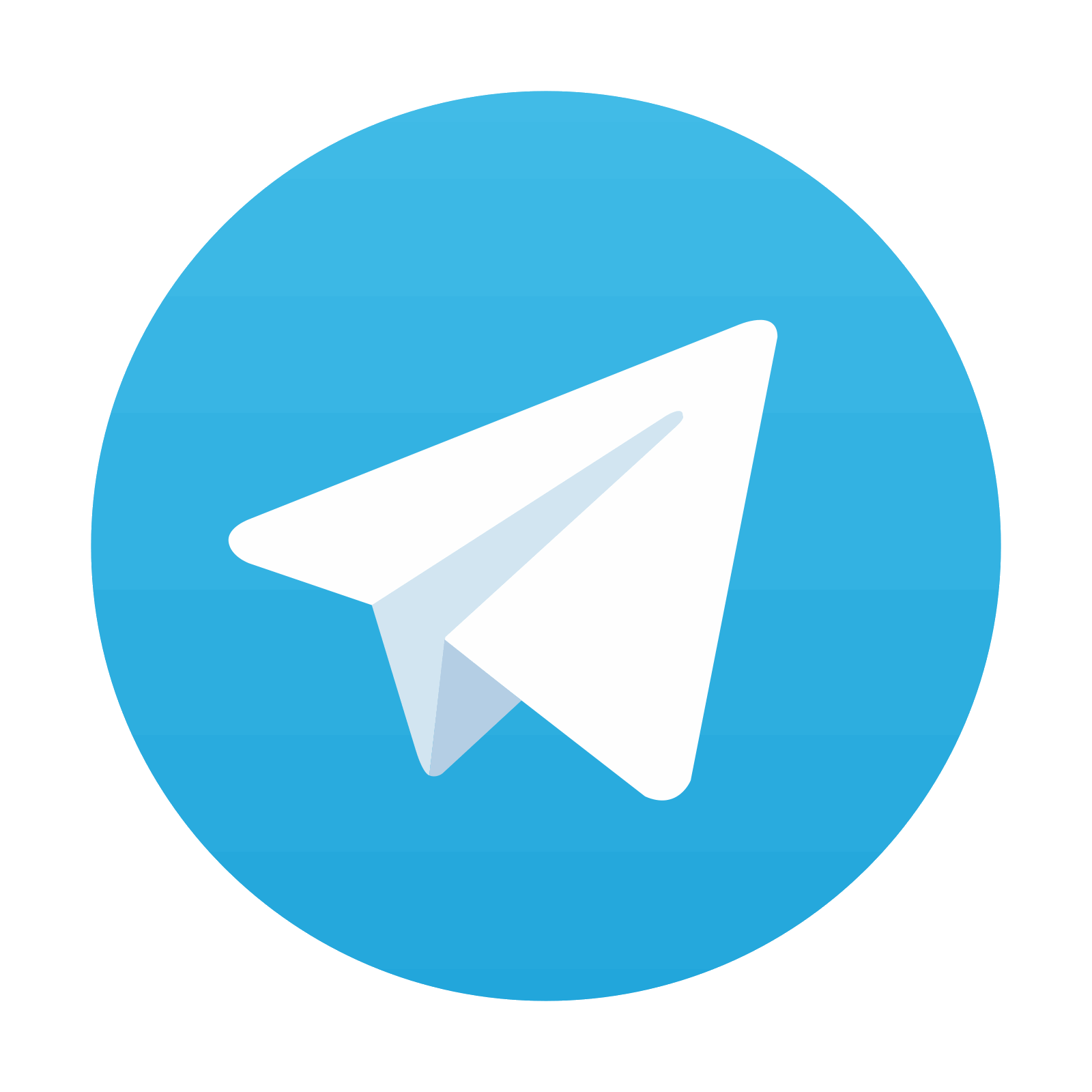
Stay updated, free articles. Join our Telegram channel
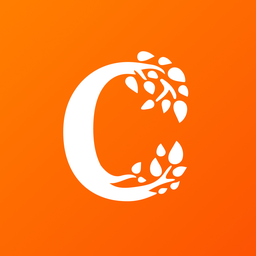
Full access? Get Clinical Tree
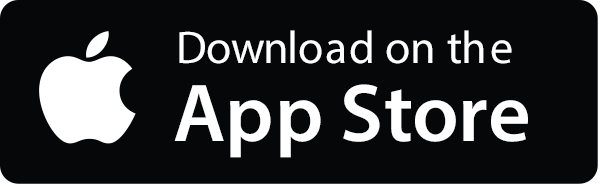
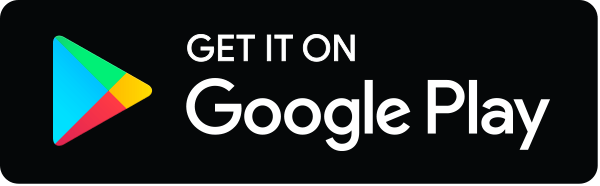