Jonathan M. Congdon Veterinary Health & Wellness Center, College of Veterinary Medicine, North Carolina State University, Raleigh, NC, 27607, USA The most critical function of the cardiovascular system is to circulate blood continuously, ensuring adequate oxygen delivery and survival of cells and tissues. The body can survive food and water deprivation far longer than it can survive oxygen deprivation and lack of perfusion; absence of oxygen delivery can trigger the complicated cascade that leads to temporary, permanent, or irreversible cell death [1]. As such, the simplest definition of cardiovascular disease is the decreased ability of this system to ensure adequate oxygen delivery for day‐to‐day survival. Nearly all anesthetic drugs compromise cardiovascular function via a single or multiple mechanism(s) and can severely compromise oxygen delivery in patients with underlying cardiac disease [2]. Cardiovascular goals during anesthesia target maintenance of oxygen delivery and homeostasis when using drugs that knowingly disturb the system. However, this goal becomes complicated in patients with underlying cardiovascular disease and increasingly more difficult when severe pathology is present. In patients with significant cardiovascular disease, the optimization of oxygen delivery requires a complete understanding of the mechanisms underlying the pathology, as well as the anesthetic drugs, patient support, and monitoring tools available. The most difficult challenge when faced with these patients is how to balance the pathophysiology of disease against the effects of anesthetic drugs and to subsequently individualize an anesthetic plan that minimizes cardiovascular compromise. It is difficult to predict all possible combinations of patient signalment and temperament, cardiovascular and comorbid conditions, clinicopathologic abnormalities, surgical procedures, and their effects on anesthetic drug choices. Thus, studies have tended to focus more on describing the specific cardiac disease or cardiac effects of specific anesthetics and less on their combinations. This approach leaves the difficult task of knowing how to choose the appropriate anesthetic plan for an individual patient. The goal of this chapter is to provide an overview of cardiovascular physiology and pathophysiology, anesthetic agents, and cardiovascular patient evaluation, monitoring, and support during anesthesia to help the clinician prepare anesthetic plans for patients with mild to significant cardiovascular disease. The mathematical definition of oxygen delivery (DO2) is the product of oxygen content (CaO2, ml O2 dl−1 blood) and cardiac output (CO, l min−1; Figure 1.1) [3]. Perfusion and the ability to deliver oxygen suffer either if the ability of the heart to eject blood (CO) is compromised or if the ability of the blood to carry oxygen (CaO2) is reduced. Although decreases in CaO2 significantly affect tissue oxygenation, the focus of this chapter is on treating reductions in CO associated with cardiac disease. It is critical to monitor blood pressure (BP) during anesthesia as it is our best, yet indirect, indicator of perfusion in veterinary medicine [4]. BP helps determine how anesthesia affects the patients’ ability to perfuse their tissues and, as such, is used as a tool to treat perfusion abnormalities. However, BP is not a component of the mathematical definition of oxygen delivery: DO2 = CO × CaO2. It is useful to assess BP to indirectly estimate changes in CO, as CO is rarely measured clinically in nonresearch patients. Systolic arterial pressure (SAP) is the peak pressure measured in the artery or arteriole during one cardiac cycle, and is determined by several variables, including stroke volume (SV, volume ejected during one ventricular contraction), velocity of left ventricular ejection, arterial resistance, and blood viscosity [5]. Diastolic arterial pressure (DAP) is the lowest arterial pressure measured during the cycle, and is affected by blood viscosity, arterial compliance, and cardiac cycle length [5]. Mean arterial pressure (MAP) is not the arithmetic mean pressure in the vessel and is always a calculated number. Various formulae exist to calculate MAP such as: MAP = DAP + 1/3 (SAP − DAP) or MAP = (SAP + [2×DAP])/3. Regarding perfusion, the most important of these values is MAP, as the total amount of time during the cardiac cycle (or during any one individual pulse) spent at SAP is very short, whereas the time spent at MAP is much longer (Figure 1.2) [6]. Autoregulation is the automatic adjustment of blood flow through a tissue regardless of the MAP driving blood through the tissue (Figure 1.3). In other words, autoregulation is the unconscious adjustment of arterial and arteriolar smooth muscle tone to maintain a constant blood flow through a tissue across a wide range of pressures. Classically, this is thought to occur between a MAP of approximately 60–160 mmHg, and is due to adaptive metabolic, myogenic, and neurogenic feedback mechanisms. Outside of this interval, tissue or organ blood flow is substantially altered, potentially resulting in reduced or nonuniform perfusion patterns [7, 8]. MAPs <60 mmHg (or SAPs <90 mmHg) have historically been considered the minimum recommended pressures in small animals associated with adequate tissue oxygen delivery [9]. However, a MAP of approximately 60 mmHg may not actually reflect adequate perfusion for several reasons. First, studies investigating autoregulation are routinely performed in nonanesthetized patients [10]. Neurogenic mechanisms for autoregulation depend on sympathetic nervous system (SNS) input. Anesthetic agents depress both the conscious and unconscious (autonomic) nervous systems. Since SNS tone is substantially reduced during anesthesia, autoregulatory mechanisms are unavoidably depressed, either partially or completely, and autoregulation is impaired. Second, if a MAP of approximately 60 mmHg is considered the minimum acceptable BP but the patient is not yet considered to be truly hypotensive, then treatments may be delayed until the patient is in a state wherein oxygen delivery is pressure‐dependent (i.e., to the left of the autoregulatory curve). As all hypotensive therapies are not instantaneously acting, there is concern that the patient may become increasingly hypotensive before treatments are efficacious. Thus, a MAP of approximately 70 mmHg (or SAP of approximately 90 mmHg) should be considered the minimum acceptable BP to build in a buffer zone so that treatments for hypotension can be applied and take effect before tissue perfusion is severely compromised, considering both altered autoregulatory mechanisms and the time‐dependent treatment effects. Figure 1.1 Determinants of oxygen delivery. Oxygen delivery (ml min−1) is the mathematical product of cardiac output (l blood min−1) and oxygen content (ml dl−1 blood). Figure 1.2 Arterial pulse pressure waveform. Mean arterial pressure (MAP) is 1/3 of the difference between systolic arterial pressure (SAP) added to the diastolic arterial pressure (DAP). MAP is considered the pressure of perfusion, as more time in the cardiac cycle is spent closer to MAP as compared to SAP. Total cycle length is 400 ms for illustration and is determined by the heart rate and other cardiovascular variables. Figure 1.3 Between MAP of approximately 60 and 160 mmHg, blood flow through a tissue capillary bed is maintained at a relatively constant flow by autoregulatory mechanisms. At MAP lower than approximately 60 mmHg and greater than approximately 160 mmHg, autoregulation of blood pressure is lost and blood flow through capillary beds becomes pressure‐dependent; tissues are either underperfused or overperfused, respectively. When considering the relationship of measured BP to the definition of oxygen delivery, one must understand the components that derive a measured BP [4]. MAP is the product of CO (l min−1) and systemic vascular resistance (SVR) (dynes s−1 cm−5). SVR is considered the degree of vasodilation (which reduces SVR) or vasoconstriction (which increases SVR) present in the systemic circulation. CO is the product of heart rate (HR, beats min−1) and SV (ml ejected per heartbeat). SV is determined by preload (the venous return during diastole preloading the ventricle before contraction/ejection), afterload (the resistance that ventricular contraction must overcome in order to eject blood), and contractility (the force of contraction of ventricular muscle, independent of preload and afterload; Figure 1.4). Increases in SVR, SV, preload, and contractility tend to increase BP, whereas increases in afterload tend to decrease SV, CO, and MAP. As MAP is a mathematical product, one cannot definitively determine if a decrease or increase in MAP is due to a decrease or increase in CO or SVR, as CO and SVR are not routinely measured in clinical patients. Choosing which mechanism for hypotension or hypertension is driving the change in pressure for a given patient requires understanding the effects of anesthetic drugs, autonomic physiology, and underlying pathophysiology, among many others. Figure 1.4 Mean arterial pressure (MAP) is the product of CO and systemic vascular resistance (SVR, the inherent tone or diameter of blood vessels). MAP is influenced by increased SVR (vasoconstriction) or decreased SVR (vasodilation). Note that MAP is not a direct component of oxygen delivery but is an indirect measurement of oxygen delivery. Cardiac output is the product of HR and SV (ml blood ejected by the heart per cardiac cycle). Stroke volume is determined by the volume of blood returning to the heart during diastole (preload), the resistance to ejection of blood during systole (afterload), and the strength of cardiac muscle contraction and relaxation (inotropy and lusitropy, respectively). Gray arrows indicate direction of change associated with hypotension (red arrow). The four mechanisms based on this algorithm are vasodilation, bradycardia, decreases in cardiac preload, and a decrease in myocardial contractility (Figure 1.4). These mechanisms of hypotension have a variety of causes (Figures 1.5–1.8) and treatments (Figure 1.9); lists within these figures are not meant to represent all causes and treatments but demonstrate common examples of those found in practice. For example, vasodilation can be treated either with (i) fluid boluses (crystalloids or colloids) to expand vascular volume to “fill” the vasodilated vasculature, or with (ii) administration of vasoconstricting agents to “offset” the vasodilation (phenylephrine, vasopressin, norepinephrine, etc.) or a positive inotrope that has vasoconstrictive properties (e.g., dopamine). Bradycardia can be treated with anticholinergics for sinus bradycardia or second‐degree atrioventricular (AV) block, or with other antiarrhythmics directed at specific bradyarrhythmias. Decreases in cardiac filling can be treated with blood volume replacement (crystalloid or colloid boluses), or with reversal or removal of obstructions or compression of the cranial and caudal vena cava. Finally, decreases in myocardial contractility from any cause can be treated either by reducing or removing the cause or with positive inotropes that improve contractility. As inhalant anesthetics are moderately to severely depressant on myocardial contractility and vasodilation (depending on dose), reducing the inhaled anesthetic dose (or requirement) of a patient can dramatically improve contractility and hypotension. Figure 1.5 Example causes of decreased heart rate via disease, complications of a procedure (e.g., ocular or vagal stimulation), or drug side effects that can be used not only to treat a cause of bradycardia from patient comorbidities or procedures but also for management before or during anesthesia. Figure 1.6 Example causes of vasodilation (decreased SVR) via disease, complications of a procedure (e.g., septic shock or anaphylaxis), or drug side effects that can be used not only to treat a cause of vasodilation but also to predict potential vasodilation from patient comorbidities or diseases for management before or during anesthesia. Figure 1.7 Example causes of decreased venous return (i.e., preload), either via disease or complications from drug side effects. Figure 1.8 Example causes of decreased contractility (or inotropy) via disease, complications of a disease, or drug side effects that can be used not only to treat a cause of decreased contractility but also to predict potential negative inotropy from patient comorbidities or anesthetics, or for management before or during anesthesia. Figure 1.9 Suggested treatment options for each underlying mechanism of hypotension are presented. Vasodilation can be treated with either volume resuscitation, vasoconstrictive agents, or reducing inhalant anesthetics. Bradycardia can be treated with anticholinergic or antiarrhythmic agents, or by treating the underlying cause of bradycardia (e.g., hyperkalemia). Decreased preload can be treated with volume boluses or correction of the inciting cause of loss of preload (e.g., obstruction to venous return or controlling hemorrhage). Decreased contractility can be treated with either minimizing the inciting cause (e.g., inhaled anesthetics) or with positive inotropic drugs (e.g., dopamine, dobutamine, etc.). It is critical to understand that these mechanism(s) and cause(s) exist not only in the anesthetized patient but also in the patient with preexisting disease or abnormal physiology (e.g., pregnancy, neonates, and geriatrics), and this approach can be used not only in the anesthetized patient but also in planning ahead for hypotension and other complications under anesthesia. Underlying cardiac disease necessitates a more extensive patient evaluation compared to noncardiac patients [11, 12]. For example, patient history should include previous cardiovascular diagnoses, medications, and any recent changes in medication dosages. Historical radiographs, electrocardiography (ECG) or Holter monitor evaluation, BP, and echocardiogram findings should be available. Patients with severe disease should be evaluated within one to two weeks of a planned anesthetic procedure. Although a complete physical examination (PE) should be performed before and on the day of anesthesia, attention must be paid to the cardiovascular and respiratory systems. The localization and characterization of heart murmurs, changes in lung sounds, increases in respiratory rate and effort, poor color and refill of mucous membranes, presence of jugular pulsations, and pulse irregularities or pulse deficits are obvious indicators of potential heart disease or changes in the patient’s cardiovascular status. A minimum database for cardiac disease should include assessments of organ function with a blood chemistry panel, electrolytes, and a complete blood count. Patients with cardiac disease should have some combination of preanesthetic ECG, BP, thoracic radiographs, and echocardiogram depending on the type of cardiac disease. Ideally, the entire workup should be completed for patients presenting with a cardiac murmur or arrhythmia and previously unrecognized cardiac disease. Previous texts [12] have established a functional classification of cardiac disease based on clinical signs to help the clinician recognize which patients may have a higher risk of anesthetic complications and for whom anesthesia should be avoided until the patient has stabilized. If the presenting complaint necessitates anesthesia, this classification alerts the clinician to the high‐risk nature of such patients for owner counseling, preanesthetic preparation, requirements for intensive patient monitoring, and patient support. Classification I comprises all nonclinical patients with preexisting cardiac disease that can be anesthetized with no preanesthetic stabilization. Classification II includes patients who have preexisting cardiac disease with mild to moderate clinical signs of disease at rest or with exercise. These patients require significant stabilization with medications and/or hospitalization before anesthesia. If anesthesia is required for a life‐saving procedure, immediate stabilization with parenteral medications before anesthesia is required. Aggressive and invasive monitoring is necessary due to their fragile nature. Classification III includes patients with ongoing, fulminant heart failure. Anesthesia is contraindicated until the patient can be stabilized. If anesthesia cannot be avoided due to a life‐saving procedure, they carry the highest risk of anesthetic complications, including severe debilitation, morbidity, and mortality. The American Society of Anesthesiologists (ASA) patient status classification scheme has been adapted by The American College of Veterinary Anesthesia and Analgesia (ACVAA; Table 1.1). The ASA patient status value is not intended to be a risk assessment; the assignment of patient status implies only the presence or absence of disease and that the clinician has evaluated the health status of the patient. The ASA physical status classification has limitations and is overly vague. However, the ASA does not (and presumably will not, as these definitions were accepted in 1963) expand on these limited definitions. Therefore, the clinician must individually determine the ASA status assignment of a patient with cardiac disease. Some authors have suggested classic types of patients who may be categorized into a physical status to help guide clinicians in the determination of ASA physical status [13]. Sedation is defined as “a state characterized by central depression accompanied by drowsiness and some degree of centrally induced relaxation. The patient is generally unaware of its surroundings but can become aroused and is responsive to noxious stimulation” [13]. General anesthesia has been described as “insensibility”; it refers to the loss of sensation to any part of the body, or to its entirety. Anesthetic induction results from drugs that depress the nervous system locally, regionally, or centrally (central nervous system; CNS) [13]. Surgical anesthesia is “the state/plane of anesthesia that provides unconsciousness, amnesia, muscular relaxation, and hypoalgesia sufficient for painless surgery” [13]. The choice between whether to simply sedate a patient for a procedure or to use general anesthesia is important to consider. The degree of CNS depression that accompanies general anesthesia also depresses autonomic reflexes, the effects of which may be avoided if sedation is an acceptable alternative for the planned procedure. Systemic sedation that is appropriate for the patient’s temperament and underlying disease in combination with locoregional analgesia may be sufficient for surgical analgesia in some cases [14–16]. Table 1.1 American Society of Anesthesiologists (ASA) physical status classification. Patient physical status adapted from the ASA physical status classification [13]. Clinical examples have been suggested by some authors; however, patient classification is highly variable and must be determined by the individual clinician. Although sedation may appear to be a universally safer option due to the avoidance of CNS/autonomic depression, sedatives such as alpha‐2‐adrenergic receptor agonists and phenothiazines may be absolutely contraindicated with some types of cardiac disease [17, 18]. Moreover, the degree of cardiovascular depression may be difficult to treat (particularly in the case of the alpha‐2‐adrenergic agonists) without reversal of the sedative with an antagonist reversal agent. In some cases, sedation may be preferred; however, general anesthesia with cardiovascular‐sparing protocols and appropriate patient monitoring and support may be the safer option. There is no single ideal anesthetic agent or anesthetic protocol for all cardiovascular disease; no one plan that will work for all patients and all procedures. All anesthetic plans should be individualized for the patient’s cardiovascular status and coexisting disease. Optimizing the plan requires a complete understanding of anesthetic drug effects and side effects, as well as pathophysiology of disease, to combine the two for optimal outcome. Premedication is an extremely important step in the process of anesthetizing patients because it provides sedation, analgesia, and a reduction in induction and maintenance drug doses [2]. As the induction and maintenance agents frequently are associated with severely depressant cardiovascular effects (albeit drug‐ and dose‐dependent in most cases), a large step toward cardiovascular stability can be provided with mild to moderate sedation with appropriate premedication. Opioids are a mainstay of premedication, induction, and maintenance of anesthesia in patients with cardiac disease, as they have minimal cardiovascular effects [19–22]. Bradycardia is the major consequence of opioid use, as opioids have minimal to no effects on cardiac contractility or vascular tone [23–25]. Bradycardia can be controlled with treatment or concomitant premedication with an anticholinergic (such as atropine or glycopyrrolate). Differences among the large number of opioids can cause confusion in choosing the most appropriate drug in this class. Generally, opioids will produce better sedation in the very young, old, or compromised patient as compared to a normally healthy adult patient. This is especially important to consider in debilitated patients with cardiac disease. Opioids are traditionally described based on their receptor target (mu‐, kappa‐, delta‐opioid receptors) and ability to exert desired clinical effects (e.g., efficacy) including sedation, analgesia, vomiting, etc. Morphine is considered the basis for comparison of all other opioids. Morphine is a full mu‐opioid receptor agonist and provides very good sedation, often perceived as the best sedating choice in this class of drugs. It is also the most likely drug to induce vomiting [26]. It is absorbed rapidly when given intramuscularly (IM) [27], and its duration is 4–6 h. Intravenous (IV) bolus administration is not recommended due to the risk of histamine release [28]. Morphine can, however, be delivered by low dose constant rate infusion (CRI) and provides significant reductions in inhaled maintenance anesthetic requirements [29]. Hydromorphone and oxymorphone have very similar profiles in small animals [30, 31]. Both are full mu‐opioid receptor agonists and provide excellent analgesia. They are moderately sedating opioids and are slightly less likely to induce vomiting compared to morphine [26]. Hydromorphone (as well as morphine) may cause panting in clinical canine patients, which may be undesirable for sedated procedures. Hydromorphone has also been reported to cause postoperative hyperthermia in cats at standard clinical doses [32]; however, the clinical relevance of this is unclear [33]. Methadone is a synthetic full mu‐opioid receptor agonist with dose‐dependent [34], mild to moderate sedative effects [35], and minimal side effects. When given alone, panting and defecation are commonly reported [34, 36, 37], though vomiting is less common when compared to morphine [26]. Methadone delivered either intravenously or epidurally significantly reduces the requirement for isoflurane in dogs [38, 39], thus reducing the dose‐dependent cardiovascular depression from isoflurane. Methadone is a superior analgesic in dogs when compared to butorphanol for postoperative analgesia following ovariohysterectomy [40]. Interestingly and different than other opioids, methadone also binds and is an antagonist at the N‐methyl‐D‐aspartate (NMDA) receptor [41]. Methadone may also be a more effective analgesic for chronic pain based on this activity [42]. Fentanyl is a synthetic full mu‐opioid receptor agonist that is 80–100 times more potent than morphine, implying that an equally effective dose is 80–100 times less than that of morphine [43]. Owing to its short duration of action (20–30 min after bolus administration), fentanyl is most useful for IV premedication, induction, or delivery by CRI [22]. Fentanyl is minimally sedating and is extremely unlikely to induce vomiting [44]. It is very well suited for use as a sole anesthetic induction agent at high doses or as part of a multidrug induction protocol. Remifentanil, sufentanil, and alfentanil are derivatives of fentanyl with similar beneficial effects regarding their analgesic efficacy and ability to profoundly decrease inhalant requirements. They all carry the same side effects of dose‐dependent respiratory depression, bradycardia, and potential hypotension. Remifentanil is an ultrashort acting synthetic fentanyl derivative with a very short elimination time due to extrahepatic elimination by plasma esterase enzymes and thus must be delivered by constant infusion. Remifentanil decreases sevoflurane minimum alveolar concentration (MAC) in dogs by 30–60% in a dose‐dependent fashion [45], and reduces isoflurane requirements in cats [46] and dogs [47]. Butorphanol is a mixed opioid receptor agonist–antagonist; it is an agonist at the kappa‐opioid receptor and an antagonist at the mu‐opioid receptor [43]. Therefore, it is only indicated for mild to weakly moderate pain as it has analgesic effects only at the kappa‐receptor; it is a very poor analgesic for moderate to severe pain [48]. Although it has a relatively short duration of analgesic action (45–90 min), it can be sedative in small animals and thus used for conscious procedures either as a sole IV sedative or in combination with other more potent sedatives, depending on the requirements. Bradycardia is less likely to occur after butorphanol administration than with full mu‐opioid agonists. It is very unlikely to cause vomiting and demonstrates a “ceiling effect” in which no further sedation or analgesia is seen beyond 0.8 mg kg−1 [49, 50]. Nalbuphine is a semi‐synthetic mixed agonist–antagonist similar to butorphanol, historically used in human labor and delivery, but is less commonly used in veterinary medicine [51]. It is related chemically to oxymorphone and has a similar potency (though not efficacy) to morphine. As a kappa‐agonist, it also appears to have a “ceiling effect” with fewer issues including respiratory depression, bradycardia, and others [52]. Epidural nalbuphine has been shown to reduce the isoflurane requirement by 26–38% in dogs [53]. Interestingly, despite reportedly similar opioid receptor effects, butorphanol may be a better sedative choice in dogs compared to nalbuphine when used alone [52], though this may not be observable when combined with dexmedetomidine/Telazol in cats [54]. Buprenorphine is unique among the common opioids in that it is a partial mu‐opioid receptor agonist. Buprenorphine has an extremely high affinity for the mu‐opioid receptor, such that it outcompetes other opioids for receptor binding, but cannot evoke a full mu‐opioid receptor response [55]. Therefore, it is not equally efficacious compared to full mu‐opioid receptor agonists. It also demonstrates a “ceiling effect” in that doses above 0.04 mg kg−1 do not provide additional analgesia or sedation. Owing to receptor binding, buprenorphine is poorly reversible to irreversible with opioid antagonists [56]. It is very unlikely to cause bradycardia and vomiting and is a relatively poor sedative. In addition to its conventional formulation, buprenorphine is available in both sustained release [57] and high‐concentration preparations [58]. Acepromazine is a common premedication and is an excellent tranquilizer in small animals. Sedation from phenothiazines is principally due to blockade of central G‐protein‐coupled, dopaminergic D2 receptors that lead to a decrease in cyclic AMP (cAMP), adenylate cyclase, and calcium conductance, and thus neuronal‐firing activity [59]. However, it is an alpha‐1‐adrenergic receptor antagonist and administration may lead to peripheral vasodilation and hypotension [60] (along with potential negative inotropic effects). Although reductions in afterload may be beneficial in some patients with cardiac disease (such as mild aortic stenosis [AS]), and animals with stable, nonclinical disease may be able to compensate for the vasodilatory effects, it may be prudent to avoid acepromazine in patients with moderate to severe cardiac disease that cannot tolerate decreases in preload and/or afterload. In addition, hypotension may lead to a compensatory increase in HR, which can increase myocardial oxygen consumption. Acepromazine may protect the myocardium from epinephrine‐ and barbiturate‐induced arrhythmias [61]; however, this benefit must be weighed against the hypotensive effects. Benzodiazepines (diazepam and midazolam) are good choices for sedation in patients with cardiac disease. They have minimal to no effects on HR, contractility, or vasomotor tone [62], and do not lead to hypotension across a wide range of doses (0.5–2.5 mg kg−1 IV). Although respiratory rate decreases, arterial blood gas values do not change appreciably [63]. The major disadvantage of benzodiazepines is that they are inconsistent sedatives in dogs [64, 65] and may be poor sedatives in cats. For example, IV premedication doses can lead to dysphoria, excitement, ataxia, arousal, and, potentially, violent aggression [64]; combinations of butorphanol and midazolam fail to provide sedation in healthy cats [65]. Although benzodiazepines decrease inhaled anesthetic requirements, this benefit can be achieved when they are combined with induction agents during the induction protocol as opposed to risking excitement when used as premedications [66, 67]. Alpha‐2‐adrenergic receptor agonists (dexmedetomidine, medetomidine, xylazine, etc.) may be relatively contraindicated in patients with cardiac disease. Alpha‐2‐adrenergic receptor agonists cause intense peripheral vasoconstriction and decrease sympathetic outflow from the CNS. The severe initial increase in SVR leads to a marked increase in BP, a significant increase in myocardial afterload, and a baroreceptor‐mediated reflex bradycardia. Some patients may demonstrate a period of vasodilation and arterial hypotension after the initial hypertension. This is commonly seen with xylazine in horses, but appears to be less common with longer‐acting agents such as dexmedetomidine [68]. The initial baroreceptor‐mediated bradycardia is exacerbated by a decrease in centrally mediated descending sympathetic tone associated with reduced synaptic norepinephrine release. Alpha‐2‐adrenergic receptor agonists can also produce bradyarrhythmias such as AV blockade and ventricular escape cardiac rhythms. At sedative doses (≥5 μg kg−1 IV), dexmedetomidine will decrease CO by 50–60%, and medetomidine at 20 μg kg−1 IV will decrease CO by at least 60% [69–71]. The increase in afterload from vasoconstriction, increase in left atrial pressure from centralization of blood volume, and decrease in CO are all mechanisms that can be detrimental to the function of a heart with underlying disease. Although alpha‐2‐adrenergic receptor agonists are extremely reliable sedatives, the cardiovascular side effects may be significant enough that the depth of sedation may be better sacrificed in the interest of cardiovascular safety. Some studies have shown promise for use of dexmedetomidine in cats with hypertrophic cardiomyopathy (HCM) [72], though further study is needed to clarify its cardiovascular effects in HCM. Alfaxalone is a neuroactive steroid‐like molecule that is capable of inducing general anesthesia through binding and interaction with the gamma aminobutyric acid (GABA) receptor [73]. Alfaxalone can be administered IV for anesthetic induction (discussed in the next section) or IM for sedation that has been reported in multiple species, including dogs, cats, and rabbits [74–78]. Typical IM dosing of alfaxalone for sedation ranges from 1 to 5 mg kg−1 IM [74, 75]. It should be combined with other sedative/analgesics [79], as alfaxalone sedation alone has higher rates of side effects including paddling, twitching and ataxia [80]. Alfaxalone doses of up to 5–8 mg kg−1 IM have been reported but carry the risk of increased respiratory depression and/or inadvertent general anesthesia. Higher doses may require oxygen supplementation, pulse oximetry monitoring [70], or possibly endotracheal intubation and ventilatory support, as higher doses may precipitate general anesthesia rather than sedation [81]. Atropine and glycopyrrolate are parasympatholytic, anticholinergic agents used to increase HR amid vagally‐mediated sinus bradycardia and AV block. Atropine has a faster onset time (1–2 min IV), shorter duration of action (20–30 min IV), and is more likely to incite tachyarrhythmias [82]. Glycopyrrolate has a longer onset time (2–4 min IV), longer duration of action (1 h IV), and may be less likely to cause tachyarrhythmias [82]. Low doses of atropine and glycopyrrolate can initially precipitate second‐degree AV block (see the following sections), which may require additional doses of anticholinergic for treatment. Anticholinergics should not be indiscriminately combined with alpha‐2‐adrenergic agonist use, as while they will prevent or treat the bradycardia associated with alpha‐2‐agonist usage, the combination of a higher heart rate with increased systemic vascular resistance typical of alpha‐2‐receptor agonists places significant burden on the heart and might potentiate cardiac arrhythmias [70]. Routine premedication with anticholinergics has fallen out of favor, as the potential side effects including decreased gastrointestinal motility, thickened respiratory secretions, and potential for tachycardias are present. Anticholinergic use should be tailored to individual patient needs. The main advantages of propofol are the rapid onset (15–20 s) and short duration of action (6–10 min of anesthesia) from an IV bolus that allow intubation and make it a suitable choice for a CRI [83]. The main mechanism of action [84] is stimulation of the GABA (the main inhibitory neurotransmitter in the CNS) receptor away from the binding site for other anesthetics such as thiopental [85]. Recoveries from propofol administration are generally smooth. However, propofol is a significant dose‐dependent vasodilator [86] and can precipitate significant hypotension at moderate bolus doses. While patients with mild cardiac disease may tolerate hypotension associated with propofol, those with more severe disease or in whom a decrease in SVR will worsen cardiac function should be cautiously induced with propofol. Alfaxalone was originally reported in 1971 as a new steroid hypnotic general anesthetic agent [87]. It is poorly water‐soluble and so was formulated in a polyethoxylated castor oil vehicle which led to adverse reactions including hyperemia in cats [88] and anaphylactoid reactions in dogs [89]. A newer formulation [90] in a beta‐cyclodextrin molecule allowed solubilization while avoiding previous side effects. Alfaxalone provides rapid and smooth anesthetic induction of anesthesia when administered by bolus. Although it should always be administered “to effect,” the manufacturer‐recommended induction dose in dogs is 1.1–2.2 mg kg−1 IV (range: 0.2–4.5 mg kg−1 IV), and in cats it is 2.3–4.0 mg kg−1 IV (range: 1.1–10.8 mg kg−1 IV), with doses depending greatly on degree of sedation from premedication [91]. Similar to propofol, alfaxalone causes dose‐dependent cardiorespiratory suppression (hypotension, apnea) in cats and dogs [92, 93]; however, clinically relevant doses show good cardiovascular stability. For example, IV alfaxalone administration increases heart rate, suggesting maintenance of the baroreceptor reflex post induction [77]. This property distinguishes alfaxalone from other induction agents and may contribute to its cardiovascular stability. A comparison of propofol (8 mg kg−1 IV, to effect) and alfaxalone (4 mg kg−1 IV, to effect) for anesthetic induction found no significant differences in physiologic variables, quality of induction, duration of intubation, or quality of recovery [94]. Alfaxalone was associated with a higher likelihood of undesirable events (e.g., excitement, paddling, twitching, apnea), though these events were not thought to be clinically serious. Dissociative anesthetics such as ketamine and tiletamine produce anesthesia by interrupting neuronal transmission, thus “dissociating” the centers responsible for consciousness and unconsciousness from the peripheral ascending inputs. The cardiovascular effects of dissociative anesthetics result from stimulation of the SNS, increasing HR, contractility, and MAP, with little change in SVR [95]. This leads to an increase in myocardial work and myocardial oxygen demand, which is compensated for by increased CO and coronary blood flow [96]. The increase in oxygen demand in patients with cardiac disease may worsen cardiac function or arrhythmias. Thus, ketamine, especially at high doses, should be used cautiously in patients where increases in myocardial contractility or work would be detrimental [97]. Etomidate is a nonopioid, nonbarbiturate sedative hypnotic drug that works similarly to propofol and barbiturates in that it enhances inhibitory GABA effects [98]. Etomidate has the distinct advantage of having minimal to no cardiovascular depression because it does not significantly change HR, contractility, afterload, or venous return [99, 100]. However, it has several drawbacks. Etomidate can cause adrenocortical suppression and has a very high osmolarity (approximately 4800 mOsm l−1) that can lead to osmolar shifts, phlebitis, pain at the injection site, red blood cell crenation, and potential hemolysis [101]. Etomidate causes a reliable but relatively slow transition to unconsciousness when compared to propofol. Etomidate has poor muscle relaxation and can stimulate myoclonus, and so should be given with a benzodiazepine or opioid such as fentanyl to facilitate a smooth induction period [99]. High‐dose, full mu‐opioid receptor agonists such as fentanyl can be extremely effective in producing general anesthesia with uncomplicated placement of an endotracheal tube. High‐dose opioids have the disadvantage of moderate to severe respiratory depression and bradycardia; however, both are easily controlled with intubation and anticholinergics, respectively. Unfortunately, transition to unconsciousness with fentanyl appears somewhat less reliable and slower compared to propofol [102]. Patients who are bright and energetic or are stimulated during the induction process by sound, touch, or pain may attempt to “override” the induction process, leading to poor induction quality. In these cases, a rescue induction agent (typically propofol for speed of onset or etomidate) can help push such a patient into unconsciousness. Quiet, dimly lit environments with little stimuli are ideal for fentanyl inductions, and fentanyl inductions usually work best in debilitated, older animals. Fentanyl should be given with a benzodiazepine for improved muscle relaxation. Inhaled anesthetics are the most chosen drugs for maintenance of anesthesia. Although injectable maintenance protocols exist and are referred to as total intravenous anesthesia (TIVA) protocols (described in the following text; Table 1.2), inhaled anesthetics provide several unique advantages. Their pharmacokinetic properties allow for careful titration of and rapid changes in anesthetic depth. Inhaled anesthetic use requires an anesthetic vaporizer and a carrier gas flow (often 100% oxygen), which supports maximal arterial blood oxygenation. The use of an anesthesia machine with associated endotracheal intubation or laryngeal mask airway placement allows for more accurate monitoring of ventilation. In addition, ventilation can also be supplemented and/or supported more easily. The ability to monitor expired gases such as carbon dioxide or expired anesthetic concentrations allows for more robust patient monitoring and support. Unfortunately, inhaled anesthetics depress cardiovascular function, leading to dose‐dependent CO and BP depression [121]. This is due to a moderate to severe dose‐dependent reduction in myocardial contractility (i.e., negative inotropy) and subsequent decreases in SV and CO [121–124]. All modern inhalant anesthetics (isoflurane, sevoflurane, desflurane) also decrease SVR (i.e., vasodilation), which can incite or predispose to hypotension. Generally, these cardiovascular effects are managed either by minimizing the dose administered or by counteracting the side effects with interventions aimed at providing cardiovascular support. Many strategies are available to reduce the minimum alveolar concentration, or MAC, of inhaled anesthetic agents required to produce lack of response to a supramaximal noxious stimulus applied to a patient 50% of the time; means of reducing MAC and thereby reducing the inhaled anesthetic dose include use of premedicants, induction agents, bolus‐ or infusion‐dose analgesics or sedatives, and local/regional anesthesia techniques. The ability to decrease the inhaled anesthetic requirements has been extensively studied (see Tables 1.3 and 1.4). The hypotensive effects of inhaled anesthetic agents can be treated by a variety of mechanisms, including optimizing HR and rhythm, judicious use of IV fluids (if not contraindicated by cardiovascular disease), increasing SVR with vasoactive medications, and directly increasing contractility with positive inotropic drugs (Figure 1.9). Table 1.2 Selected propofol and alfaxalone infusion doses. TIVA is general anesthetic maintenance using titrated or computer‐controlled infusions of anesthetic, sedative, and analgesic combinations, rather than anesthetic maintenance with inhaled anesthetics. Inhaled anesthetic use carries the disadvantages of dose‐dependent patient cardiopulmonary depression, potential risk of waste‐gas exposure to hospital staff, and the environmental impact of inhalants as greenhouse gases [134]. Inhaled anesthetics require a vaporizer for controlled dosing, an anesthesia machine for delivery, and waste anesthetic gas scavenging. Clinical circumstances may find inhalational anesthesia undesirable (e.g., head trauma, increased intracranial pressure) or impossible (e.g., nonintubated procedures, long‐term intensive care unit [ICU] ventilators without a vaporizer), and TIVA may be the best option. TIVA techniques require a delivery system such as a syringe pump, an intravenous catheter(s), and a dosing‐control schedule. Despite avoiding inhaled anesthetics, endotracheal intubation and an anesthesia machine are strongly recommended with TIVA for delivery of oxygen, ability to monitor respiratory function and end‐tidal gases (e.g., capnography), and to support or control ventilation. Table 1.3 Examples of MAC‐reducing effects of common CRIs in cats. MAC is the minimum alveolar concentration of an inhaled anesthetic (the concentration of an inhalant at the pulmonary alveolar level) that prevents purposeful responses (i.e., not a reflex response) to noxious stimuli in 50% of patients. MAC reduction is typically defined as the percent reduction in MAC dose; the lower dose of inhalant that maintains 50% response to noxious stimuli with concurrent use of an example infusion during inhaled anesthesia. MAC reduction maintains a surgical plane of anesthesia at a lower inhalant level to reduce the side effects (e.g., vasodilation and decreased contractility). μg = micrograms. Table 1.4 Examples of MAC‐reducing effects of common CRIs in dogs. MAC is the minimum alveolar concentration of an inhaled anesthetic (the concentration of an inhalant at the pulmonary alveolar level) that prevents purposeful responses (i.e., not a reflex response) to noxious stimuli in 50% of patients. MAC reduction is typically defined as the percent reduction in MAC dose; the lower dose of inhalant that maintains 50% response to noxious stimuli with concurrent use of an example infusion during inhaled anesthesia. MAC reduction maintains a surgical plane of anesthesia at a lower inhalant level to reduce the side effects (e.g., vasodilation and decreased contractility). NR = not reported; μg = micrograms. TIVA dosing can be calculated to either a CRI where a consistent dose of drug is delivered, or with a target‐controlled infusion (TCI) dose to target a consistent plasma concentration of drug. A complete description of TCI is beyond the scope of this text. However, in brief, TCI dosing requires previously determined pharmacokinetics for the drug of interest, including the species of interest and the effective plasma concentration; TCI infusions can be controlled manually or with computer programs, based on availability [135, 136]. To date, few publications are available for TCI use in clinical veterinary medicine. Thus, CRI dosing is currently the more common technique used clinically. A wide variety of TIVA techniques have been employed and, though propofol has dominated the TIVA landscape [137], alfaxalone is a suitable alternative. Selected references for TIVA with alfaxalone and propofol are presented in Table 1.2 [103–120]. Dosing for CRI propofol infusions ranges 0.2–0.6 mg kg−1 min−1 (dog) and 0.2–1.0 mg kg−1 min−1 (cat). Dosing for alfaxalone infusions ranges 0.07–0.12 mg kg−1 min−1 (dog) and 0.18 mg kg−1 min−1 (cat). One major goal of adjunctive techniques or interventions is to increase cardiovascular stability and maximize CO and BP. In practice, this can be generally summarized as applying a technique that has fewer negative cardiovascular side effects as compared to patient management without that technique. As an example, fentanyl infusions have been shown to reduce the requirement for enflurane by as much as 65% [138] and of isoflurane by approximately 50% [128] at 0.8 and 0.3 μg kg−1 min−1, respectively. As the primary cardiovascular effect of opioid infusions is bradycardia, which is easily corrected with anticholinergics, these infusions allow for decreased inspired inhalant concentrations, therefore reducing their cardiovascular compromise. This is presumed to be safer by providing improved cardiovascular stability than using higher doses of inhalants alone. Other anesthetic adjunctive techniques, including nonopioid analgesic CRIs (lidocaine and ketamine) and local and regional anesthesia (epidurals, peripheral nerve blocks, and local anesthetics), are aimed at reducing maintenance anesthetic requirements in the interest of cardiovascular stability. Local anesthetics have the distinct advantage of blocking peripheral nerve function as compared to other analgesic drug classes (opioids and nonsteroidal anti‐inflammatory drugs [NSAIDs]) that modulate the ascending nociceptive stimuli. If nociceptive stimuli are completely prevented from reaching higher centers, then, theoretically, a patient would not require general anesthesia despite painful surgery or procedures. Although this may not be practical for most procedures, it reminds us that local anesthetics are a powerful tool in preventing pain perception or ascending nociceptive information. For patients under general anesthesia, local or regional anesthesia/analgesia can dramatically reduce systemic and inhaled anesthetic drug requirements. As local anesthetics have minimal cardiovascular compromise at appropriate doses, the reduction in systemic and inhaled anesthetic drug levels can minimize or prevent the cardiovascular depressant effects of these anesthetics, leading to a more stable patient. Numerous studies have shown a significant reduction in inhaled anesthetic requirements due to application of regional anesthesia techniques, including infraorbital nerve blocks [139] and opioid‐based epidurals in dogs and cats [38, 140] as some examples. Thus, even though local anesthetics are associated with neurotoxicity and cardiotoxicity at high doses [141, 142], they are extremely valuable parts of multimodal anesthetic techniques. Newer texts focus on reviewing anatomy and approach for a large variety of locoregional anesthesia and analgesia techniques, both blindly and with ultrasound and/or or nerve stimulator guidance [143]. Much like local and regional techniques, systemically delivered analgesic infusions have the significant potential for reducing inhaled anesthetic drug doses and responsiveness to painful stimuli. If the cardiovascular side effects of the infusion(s) are not more detrimental than the inhaled anesthetic, the reduction in inhaled anesthetic dose can lead to a significant reduction in their negative consequences, such as negative inotropy, vasodilation, and respiratory depression, thus improving cardiovascular performance. As mentioned previously, IV opioid infusions are particularly beneficial in reducing inhaled anesthetic requirements (Tables 1.3 and 1.4) [46,124–133] and are relatively safe infusions, as their primary side effect is bradycardia, easily treatable with anticholinergics. In horses anesthetized with sevoflurane, an IV lidocaine bolus of 1.3 mg kg−1 followed by a CRI of 50 μg kg−1 min−1 reduced sevoflurane MAC by 27% [144]. Intravenous lidocaine infusions have been studied in dogs repeatedly for their benefits in reducing both isoflurane‐ and sevoflurane‐inhaled anesthetic concentrations. Lidocaine at 50 μg kg−1 min−1 reduced isoflurane MAC by 29% [29] and sevoflurane MAC by 22.6% [145] in dogs. In another study at 50 and 200 μg kg−1 min−1, no changes in cardiovascular parameters due to lidocaine infusion(s) were identified, and inhalant MAC was reduced by 15% and 37%, respectively [145]. NSAIDs have been, and will remain, one of the most important analgesic options in veterinary medicine. A complete review of NSAID pharmacology, indications, guidelines, and adverse effects is beyond the scope of this text and is thoroughly discussed elsewhere [23146–150]. Cyclooxygenase‐2 (COX‐2) enzyme‐selective inhibitors have been associated with increased risk of heart failure, myocardial infarction, strokes, and thromboembolic disease in humans due to their negative effects on platelet and vascular homeostasis via thromboxane A2 and prostacyclin [151–154]. Accordingly, rofecoxib (Vioxx™) and parecoxib (Celebrex™) were removed from the human market by the Food and Drug Administration (FDA) due to their increased risk of cardiovascular side effects in 2004 [155]. In veterinary medicine, emesis appears to be the most common adverse effect, with renal and hepatic adverse events found at low frequency with no cardiovascular effects reported [156, 157]. However, the use of NSAIDs is cautioned during periods of hemodynamic compromise (e.g., dehydration, hemorrhage, general anesthesia, heart failure, or renal/hepatic disease), as the kidney becomes more dependent on the vasodilatory effects of prostaglandins. Use of NSAIDs during these times could result in ischemic renal damage, and possible acute renal injury [150]. Inotropes and vasopressors are drugs aimed at improving BP and perfusion by taking advantage of SNS receptor targets. In effect, they mimic (mimesis in Greek, which means “to imitate”) the SNS’s effects on the cardiovascular system. In a normal sympathetic “fight or flight” response, epinephrine is released in response to a perceived or actual threat or injury and stimulates membrane‐bound receptors. This response includes increases in heart rate (chronotropy), strength of myocardial contraction (inotropy), speed of cardiac conduction (dromotropy), increases in SVR, and bronchodilation. While the terms “inotropes” and “vasopressors” (or simply, “pressors”) are often used interchangeably, in the strictest definition, an inotrope increases contractility, and a vasopressor increases SVR. The receptor targets for epinephrine in the SNS are alpha‐1 (α1)‐, alpha‐2 (α2)‐, beta‐1 (β1)‐, and beta‐2 (β2)‐adrenergic receptors, and each receptor has a stereotypical response to stimulation (Table 1.5) [158]. Inotropes and vasopressors target these receptors, taking advantage of their cardiovascular effects, and each drug in these classes has one or more effects at these receptors. Choosing the appropriate drug to manage hypotension is a balance of the known receptor effects of the individual drug, the suspected mechanism for hypotension, potential risks of the drug, understanding of dosing and delivery techniques, and ability to deliver them safely. There are few absolute contraindications for any drug and gaining knowledge and comfort with them can often be a matter of training, clinical experience, and personal preference. It is important to note that, with few exceptions, the half‐lives of these drugs are very short (approximately 1–3 min), and dosing of most sympathomimetic drugs is through CRI rather than by individual and/or intermittent bolus administration. Dopamine is the immediate metabolic precursor to norepinephrine and, when delivered via CRI, has a dose‐dependent positive inotropic effect. Dopamine has varied receptor effects based on the infusion dose. Between approximately 5 and 10 μg kg−1 min−1, dopamine shows primarily β1‐adrenergic receptor‐mediated increases in myocardial contractility, and occasionally β1‐mediated increases in HR. The recommended dose for improvement of CO is 7 μg kg−1 min−1 [159], although the infusion rate should be tailored within the range to each patient’s individual effect. Dopamine’s actions are unique compared to other inotropes, as higher doses (above 10 μg kg−1 min−1) activate α1‐adrenergic receptors and can increase SVR. Although this can also be beneficial for BP, an increase in SVR may in fact worsen cardiovascular performance and may be contraindicated in patients with specific cardiovascular diseases such as dilated cardiomyopathy (DCM), HCM, and regurgitant valvular disease. In addition, the higher dose of dopamine carries a higher potential for tachycardias, ventricular arrhythmias, and hypertension. It is also possible that higher doses of dopamine (or any vasoconstrictive agent), particularly when given by an inadvertent bolus (i.e., with a pump malfunction, medication‐dosing error, or flushing an IV catheter in which a dopamine CRI has been delivered), may lead to acute vasoconstriction, hypertension, and reflex bradycardia. Dobutamine is a synthetic, nonspecific β‐adrenergic agonist, activating both β1‐ and β2‐receptors, and will increase both HR and contractility like the β1‐adrenergic receptor effects of dopamine. Higher doses (>10 μg kg−1 min−1) have the potential to activate α1‐receptors, leading to increases in SVR [159]. The general recommended dose for dobutamine to achieve β1‐adrenergic effects is 1–5 μg kg−1 min−1, though doses of 1–20 μg kg−1 min−1 have been reported [160]. However, it is critically important to understand that dobutamine is also a β2‐adrenergic receptor agonist and will induce a decrease in SVR, leading to β2‐adrenergic‐receptor‐mediated vasodilation. The increase in HR and contractility may be offset by the vasodilation, and despite an increase in CO (and therefore perfusion), an increase in BP may not be observed [159]. In general, dobutamine can be advantageous in patients with DCM. Table 1.5 Inotropes and vasopressors. Effects and doses of positive inotropic and vasoconstrictive infusions, with effects at various sympathetic and nonadrenergic receptors. Epinephrine is a potent α‐ and β‐adrenergic receptor agonist leading to intense peripheral vasoconstriction and increases in HR and contractility, respectively. It dramatically increases myocardial oxygen demand and is highly arrhythmogenic. It can be challenging to discriminate effects (i.e., β‐adrenergic effects without α‐adrenergic effects) with bolus‐dose epinephrine, though dose recommendations exist to target β‐adrenergic effects (0.01 mg kg−1 IV) and avoid α‐adrenergic effects (0.1 mg kg−1 IV) [161]. In cats, dose‐dependent effects of an epinephrine infusion occur between 0.125 and 2.0 μg kg−1 min−1 [162], though complete understanding of dose‐dependent effects is lacking. With the availability of other, better‐understood and lower‐risk sympathomimetic drugs for management of hypotension, epinephrine may be a questionable choice for a routine inotropic agent, particularly due to the increase in oxygen demand and potential for arrhythmias. Ultimately and ideally, epinephrine should be limited to use for cardiopulmonary cerebral resuscitation (CPCR) or cardiopulmonary bypass. Ephedrine is a noncatecholamine sympathomimetic that acts both directly and indirectly (by causing norepinephrine release) at adrenergic receptors, though the effects are not as strong [163]. Ephedrine is one of the few inotropic/vasopressive agents that can be delivered by bolus injection, rather than by infusion, as the half‐life for activity is longer than other sympathomimetic drugs. Typical bolus dose is 0.02–0.05 mg kg−1 IV . Ephedrine bolus leads to increases in BP, cardiac index, and oxygen delivery in dogs anesthetized with isoflurane [165]. Onset time is rapid, and the duration of the increase in BP is shorter than that of the increase in CO. Unfortunately, repeated doses of ephedrine have diminishing returns, as norepinephrine stores are rapidly depleted [165]. As such, it is useful for short‐term treatment of hypotension [166]. Vasopressin (antidiuretic hormone, ADH) is an exception to the sympathomimetics, as it is a nonadrenergic hormone that participates in endogenous BP control and can be delivered exogenously for management of hypotension. Vasopressin acts via the vasopressin‐1 (V1) endothelial receptor, increasing SVR and BP, but has no effect on HR, myocardial contractility, or CO [161]. Indirectly, the increase in SVR can lead to a decrease in CO and bradycardia at higher doses [7]. Actions at the vasopressin‐2 (V2) receptor are responsible for the renal effects [163]. Since it is not a catecholamine, it is not arrhythmogenic, a significant advantage over other drugs in this group. Vasopressin has been shown to be comparable to phenylephrine for the treatment of hypotension in an endotoxic shock model [164]. Although intentionally titrated vasoconstriction can be an important strategy for treatment of refractory hypotension, high levels of SVR may potentially decrease CO and oxygen delivery, particularly in patients with heart disease (e.g., DCM) for which increases in afterload can be severely detrimental [167]. Norepinephrine is the major endogenous SNS neurotransmitter [168], and has both α‐ and β‐adrenergic effects, although, in practice, the α1‐adrenergic receptor effect predominates, leading to its use primarily as a vasoconstrictor. At very low doses (approximately 0.025 μg kg−1 min−1), there is evidence that β1 effects predominate, which can be beneficial in increasing HR and CO [169]. At higher doses in dogs (0.1–0.8 μg kg−1 min−1), norepinephrine has shown dose‐dependent increases in SAP, MAP, DAP, and resultant decreases in HR [170]. Phenylephrine is a pure α1‐adrenergic receptor agonist with no β2‐receptor activity, which leads to dose‐dependent increases in SVR, and carries the benefits and drawbacks of pure vasoconstrictors as described previously [168]. Phenylephrine has been evaluated both in healthy cats [171] and for treatment of hypotension in cats with naturally existing hypertrophic obstructive cardiomyopathy (HOCM) [172]. Phenylephrine increases SVR and arterial BP, but does not increase HR, cardiac index, or calculated oxygen delivery; for treatment of isoflurane‐induced hypotension via an increase in CO, dopamine would be a superior choice to phenylephrine, as dopamine increased CO and phenylephrine did not. However, if increases in SVR and BP are desired without increases in HR, phenylephrine remains a clinically useful option. As decreases in cardiovascular function and CO are inevitable effects of anesthetics, fluid therapy is recommended to maintain perfusion despite cardiovascular depression. Patients who present with compensated heart disease with no overt clinical signs may tolerate typical rates of IV fluids (balanced electrolyte solutions) during anesthesia, usually in the range of 3–5 ml kg−1 h−1. Patients with evidence of noncompensated cardiovascular disease are often at risk for failure due to poor cardiac function or the cascade of neurohormonal mechanisms that leads to an increase in circulating blood volume such as activation of the renin–aldosterone–angiotensin system (RAAS) and increased secretion of ADH. Patients with a history of heart failure and/or chronic volume overload (mitral, tricuspid, and aortic valve insufficiency, left‐to‐right shunts including patent ductus arteriosus [PDA], and ventricular septal defects [VSDs]) may be less likely to tolerate high fluid rates during surgery; lower fluid rates should be used in these patients. Usually, 2–3 ml kg−1 h−1 is sufficient to meet maintenance metabolic needs, but not increasing blood volume and risk precipitating heart failure. Furosemide may be used for its diuretic effects if the patient receives an excessive amount of IV crystalloid solution. The administration of synthetic colloids (i.e., hetastarch, pentastarch, dextran, and hemoglobin glutamer‐200) is often avoided in patients with cardiac disease, as colloids can sustain plasma volume for significantly longer periods and are more difficult to treat/reverse with diuretics. Most anesthetic premedicants and induction agents are respiratory depressants; the most significant of which are the opioids, propofol, alfaxalone, and inhaled anesthetic agents. Ketamine is considered a minimal respiratory depressant as is etomidate [173]. The onset of respiratory depression can be very rapid, which can result in patient desaturation and cyanosis. The alveolar partial pressure of oxygen (PAO2) is predicted by the alveolar gas equation (Table 1.6). The following alveolar gas equations represent examples of differing PaCO2 levels during normoxia (inspired oxygen = 21%): and hyperoxemia (inspired oxygen = 40%): where FIO2 refers to the inspired fraction of oxygen, Patm is the atmospheric pressure (in mmHg), PH2O is the partial pressure of water vapor (in mmHg), and PaCO2 is the arterial partial pressure of carbon dioxide (in mmHg). In animals that are ventilating normally with a PaCO2 of 40 mmHg (Table 1.6, Eq. (1.2)), the PAO2 is nearly 100 mmHg. This pressure represents the alveolar pressure of oxygen able to diffuse down the oxygen concentration gradient into pulmonary arterial blood. As patients hypoventilate, PaCO2 increases (in this example, to 60 mmHg), which decreases the PAO2 and results in clinical hypoxemia when PAO2 is less than 80 mmHg (Table 1.6, Eq. (1.3)). When providing supplemental oxygen via a tight‐fitting face mask (estimated to be a FIO2 of approximately 40%), PAO2 is subsequently increased (Table 1.6, Eq. (1.4)), which can blunt the effects of hypoxemia due to hypoventilation (Table 1.6, Eq. (1.5)). Thus, preoxygenation can be a critical component of maintaining a high PAO2 and arterial partial pressure of oxygen (PaO2) subsequent to anesthetic‐related respiratory depression from premedication through the induction process. The general recommendation is to provide oxygen via a tight‐fitting face mask for a minimum of 3 min before anesthetic induction [174]. This can be easily performed, as monitoring equipment (ECG, noninvasive blood pressure [NIBP], capnometry) is placed before induction of anesthesia. Blood pressure is the most reliable clinical indicator of perfusion, despite the disadvantage that it is not a clear indicator of CO. BP is a standard monitoring tool for all anesthetized patients and has been the standard of care in humans for decades. The ACVAA guidelines on small animal patient monitoring recommend measurement of BP as part of basic patient care during anesthesia [175]. Methods of arterial BP monitoring include both invasive and noninvasive techniques. Noninvasive methods include automated oscillometric BP monitors and manual Doppler BP monitoring. Invasive (or direct) BP monitoring involves the placement of a catheter into a peripheral artery with connection to a fluid‐filled pressure transducer system. All techniques have both advantages and disadvantages regarding the ease of placement, frequency and speed of measurement, invasive nature, and technical skill required for measurement and accuracy of measurement. Table 1.6 Alveolar and arterial oxygen partial pressure calculations, based on PaCO2 and the alveolar gas equation. Alveolar partial pressure of oxygen is calculated using the alveolar gas equation: PAO2 = FiO2 (Patm − PH2O) − PaCO2/0.8. PAO2 is the alveolar partial pressure of oxygen. PaO2 is the arterial partial pressure of oxygen, measured with arterial blood gas analysis. FiO2 is the fraction of inspired oxygen (21–100%). Patm is the atmospheric barometric pressure specific to the elevation at or above sea level, assumed in the example to be at sea level, where Patm is 7–60 mmHg. PH2O is the vapor pressure of water, which varies slightly by patient temperature generally assumed to be approximately 47 mmHg. PaCO2 is the patient’s current arterial pressure of carbon dioxide, measured on arterial blood gas analysis. PaCO2 divided by 0.8 is respiratory quotient, which is the ratio of CO2 molecules produced for O2 molecules consumed in mitochondrial ATP synthesis. The normal alveolar to arterial gradient of oxygen is <10–15 mmHg in room air, and the PaO2 values calculated in Table 1.5 assume a 105‐mmHg difference between alveolar and arterial partial pressures. Refer to Eqs. (1.2)–(1.5) in the body of the text for values. Invasive blood pressure (IBP) monitoring is the gold standard with which all other forms of BP measurement are compared [176–182]. Direct monitoring is the most accurate BP measurement and offers additional benefits of being a continuous, second‐to‐second monitor for SAP, DAP, and MAP. Acute changes in the patient’s hemodynamic status can be appreciated rapidly, and alterations in the arterial pressure waveform can also provide information about patient status. The placement of an indwelling arterial catheter also allows for arterial blood gas sampling. IBP monitoring has significant drawbacks, including the skill required to place arterial catheters in potentially hypotensive, unstable patients, the requirement for a multiparameter patient monitor with the capability of connecting to a fluid‐filled transducer system, the understanding of what causes error in the transducer system, and the need for an ability to troubleshooting of the system [183]. Despite these complexities, IBP monitoring is a mainstay of advanced cardiovascular monitoring. NIBP monitoring includes both automated oscillometric monitoring devices and Doppler ultrasound BP monitoring [184]. Oscillometry uses an automated cuff that is inflated above SAP, occluding arterial blood flow. As cuff pressure is reduced, the arterial pulse begins to generate oscillations in the arterial wall that are transmitted to the cuff. These oscillations increase and then decrease in amplitude as cuff pressure is reduced, and eventually the oscillations are eliminated as blood flow becomes laminar. Although technology and calculation algorithms vary between oscillometric devices, generally, the onset of oscillations represents the SAP, the maximal oscillation amplitude represents MAP, and the cessation of oscillations is DAP. Oscillometry carries the advantage of automation and ease of use. However, oscillometric devices are fraught with error, including inappropriate cuff size. The cuff should be approximately 40% of limb circumference; oversized cuffs lead to inappropriately low readings, and inappropriately small cuffs lead to falsely elevated measurements [184]. Other issues with oscillometric devices include motion artifacts and interference from high HRs or cardiac arrhythmias [185]. Studies comparing the accuracy of oscillometric BP to direct BP found limited agreement with MAP and DAP in anesthetized dogs with 67% and 95% of readings within 10 and 20 mmHg of invasive pressure values, respectively [184]. Another study found poor correlation such that a 25‐mmHg bias was identified between invasive and oscillometric pressure in anesthetized cats [177]. Oscillometric BP devices also carry the disadvantages of slow performance compared to continuous arterial catheters. Doppler BP devices use a BP cuff that is manually inflated with a sphygmomanometer above SAP. A Doppler crystal is placed over a peripheral artery, and blood flow is audibly demonstrated with appropriate Doppler sound. When inflation of the cuff occludes flow, the Doppler signal is lost. As the cuff is manually deflated, blood flow begins to pass through the cuff and is again audible via the Doppler crystal. This is generally interpreted as the peak pressure or SAP. Blood pressure measured with the aid of a Doppler crystal can be checked manually more frequently than oscillometric devices and are simple to use. Furthermore, a Doppler crystal placed over a peripheral artery provides the anesthetist with an audible signal for blood flow, which can be a strong comfort for those moments in which the anesthetist’s attention cannot be on the patient or patient monitor. Doppler crystal placement also allows for a second assessment of BP should an arterial catheter fail. Disadvantages of Doppler crystals include that they are somewhat fragile, require more skill for placement to obtain an audible signal, and may not accurately predict SAP. For example, multiple studies have evaluated the assessment of SAP with Doppler NIBP compared to IBP [182, 183, 186]. In cats, poor agreement was found between invasive SAP and Doppler BPs, such that the Doppler underestimated SAP by approximately 14 mmHg [168, 169, 175] to 25 mmHg [182, 183]. Doppler BP measurement was not recommended when accuracy is desired. However, in rabbits, direct SAP was found to have good agreement with Doppler BP [185]. Despite the potential inaccuracy of NIBP compared to IBP, NIBP can help identify relative changes, which may help the anesthetist choose intervention options. ECG allows analysis of the cardiac rhythm. Understanding the components of the cardiac rhythm and how it relates to mechanical function of the heart helps the anesthetist to analyze the rhythm for changes that would indicate abnormalities. These abnormalities might imply that there is asynchrony in mechanical heart function and rhythm correction may improve mechanical function, CO, and perfusion. Although ECG monitoring does not “prove the patient is alive,” as there can be dissociation between the electrical and mechanical activity (termed pulseless electrical activity, PEA), it is nevertheless a basic requirement of anesthetized patient monitoring. Saturation of hemoglobin in arterial blood is an important component of the oxygen content (CaO2) equation: where the constant 1.3 is the amount of oxygen (ml at 1 atm) bound by 1 g of hemoglobin, [Hb] is the hemoglobin concentration, SaO2 is the hemoglobin saturation with O2, the constant 0.003 is the amount of O2 dissolved in plasma, and PaO2 is the arterial partial pressure of O2. As most of the O2 is carried in the hemoglobin molecule, the degree to which hemoglobin is saturated with oxygenated blood is a critical variable in oxygen delivery. The pulse oximeter is a simple tool that estimates the oxygen saturation of arterial blood (SaO2 ~ SpO2). Hemoglobin saturation of approximately 90% is correlated with a PaO2 of approximately 60 mmHg, well into the hypoxic range. Therefore, a hemoglobin saturation of >93–94% is required to ensure normoxia. Many variables can interfere with the ability of the pulse oximeter to provide an accurate arterial saturation (Table 1.7) [187]. Hypothermia has varying effects based on the amount of body temperature loss [2]. Essentially, all patients will become hypothermic to some degree because of premedication and induction of anesthesia, unless active heat support is provided. Causes of hypothermia include, but are not limited to, opioid‐, phenothiazine‐, and alpha‐2‐adrenergic‐receptor agonist‐mediated changes in thermoregulation, high surface area to body mass ratio, high cold‐compressed O2 flow rates, open body cavities, cold surfaces, room‐temperature IV fluids, cold scrub solutions, and body cavity lavage (especially orogastric lavage with fluids below body temperature). Mechanisms for heat loss include evaporation, conduction, convection, and heat loss from respiration and radiant heat loss [186]. Anesthetized patients also have decreased heat production due to central depression and inability to shiver in response to hypothermia. It is far easier to prevent than it is to treat hypothermia, as the peripheral vasoconstrictive effects of hypothermia make external rewarming difficult and inefficient. Consideration of these variables for an individual patient or procedure will help the anesthetist generate a robust heat loss prevention or rewarming plan. Table 1.7 Pulse oximetry (SpO2): sources of error. The physiologic effects of hypothermia vary with severity and include catecholamine release, decreased cerebral metabolic rate of O2 consumption and intracranial pressure, and altered electroencephalogram and arterial blood gas results [188, 189]. At moderate levels, hypothermia decreases inhalant requirements, reduces concentrations of inhalant required to produce apnea, decreases CO and BP, and increases SVR. In addition, moderate hypothermia results in bradycardia, prolonged clotting times, decreased drug metabolism, prolonged nerve conduction and muscle contraction, and a left shift of the oxygen–hemoglobin dissociation curve (which favors hemoglobin loading) [190]. With these profound physiologic changes, it is clear that body temperature should be controlled in any anesthetized patient and especially in one who may have preexisting cardiovascular compromise, as they may lack the reserves to compensate. Capnometry is the assessment of exhaled CO2 as an indicator of ventilatory adequacy. Capnometry refers to the measurement of end‐exhalation (end‐tidal) CO2; a capnograph provides a visual waveform display of the measurement of CO2 over time. However, the term capnography is often used to imply all these components. Hypoventilation is defined by an increase in the PaCO2 with a subsequent increase in end‐tidal CO2 levels. Hypoventilation leads to respiratory acidosis and should be avoided in patients with cardiac disease, as ideal cardiac function occurs at normal blood pH; acute respiratory acidosis has been shown to increase HR and CO but decrease myocardial contractility and SVR [191]. Hypercapnia increases catecholamine release [192, 193] and can result in tachyarrhythmias from the combination of acidosis, electrolyte changes due to acidosis, and CO2‐mediated increases in catecholamine release [194, 195]. It is possible to roughly estimate the change in pH on the basis of the increase in PaCO2; for every approximately 10 mmHg acute increase in PaCO2, arterial pH will decrease by approximately 0.1 pH unit [196]. Assisted or controlled ventilation can be helpful in maintaining normoventilation, to optimize oxygenation (SpO2 or PaO2) and improve inhaled anesthetic depth. Mechanical ventilation can be provided either with a controlled mechanical ventilator or with intermittent assisted manual ventilation (“bagging”). It is nearly impossible to provide the same consistency, tidal volume, respiratory rate, peak inspiratory pressure, and duration of inspiration with manual ventilation as compared to mechanical ventilation, and the use of mechanical ventilators is strongly recommended to maintain this consistency and allow the anesthetist to attend to other tasks. However, mechanical ventilation can and very often will decrease BP by one of three mechanisms [197]. First, tidal volume via mechanical ventilation is very likely to be larger than that of a spontaneously taken breath. Therefore, mechanical ventilation represents an increase in anesthetic delivery via a larger number of volatile inhaled anesthetic molecules delivered within a larger tidal volume. Inhaled anesthetics subsequently cause dose‐dependent decreases in BP, mediated by decreases in myocardial contractility and SVR. Second, increases in intrathoracic pressure during positive pressure ventilation decrease venous return (i.e., preload), leading to reduced SV, CO, and BP. The effects on CO are more pronounced with more frequent respiratory rates, longer inspiratory phase duration, and larger tidal volumes [198]. Finally, high PaCO2 can increase sympathetic tone and improve BP, an effect that is reduced when patients are ventilated to a normal or even low end‐tidal CO2 [199, 200]. Arterial blood gas analysis is a useful direct measurement of PaO2 and PaCO2 to evaluate pulmonary function and quality of ventilation. Serial monitoring of arterial blood gases helps the anesthetist to better direct ventilatory adjustments to maintain oxygenation and normal CO2 levels. The availability of arterial catheterization allows for continuous IBP monitoring, as well as serial blood sampling for clinical pathology. Normal values for arterial blood gas values are presented in Table 1.6. Central venous pressure is the intraluminal pressure measured in the intrathoracic vena cava immediately outside the right atrium [201, 202]. Historically, it has been used as an indicator of right ventricular preload and overall patient intravascular volume status [203], as well as in assessment of right heart function and critical patient care monitoring [204]. However, right ventricular preload is truly defined by the difference between the intracardiac (i.e., right ventricular) and extracardiac transmural pressure gradient [195]. A variety of factors can reduce preload but lead to an increase in measured CVP, which may lead the clinician to erroneously interpret an increase in preload. These include changes in intrathoracic pressure (changes in stage of ventilation, pleural effusion, and abdominal hypertension) and blood volume or cardiac arrhythmias. Decreases in right ventricular compliance also can increase CVP, as end‐diastolic filling pressures can be elevated with a “stiff” ventricle, pericardial disease, or pericardial tamponade. Ultimately, CVP represents the balance between volume return to the heart and cardiac function. There are many excellent, in‐depth published reviews of CVP monitoring [201–204]. Although CVP monitoring requires a complete understanding of these variables and the ability to trend values over time, it can be a valuable tool in monitoring patients under anesthesia in select cases. Arterial PPV is a dynamic measurement and is defined as the difference between the maximum and minimum arterial pulse pressure during one mechanically ventilated breath [205]. While oscillometric techniques of measurement are under development, PPV typically requires arterial catheterization and specialized monitoring equipment. PPV was originally manually calculated, and expressed as percentage of the ratio of the difference between the maximal and minimal values of pulse pressure over the mean of these two values and expressed as a percentage [206]: PPV is an accurate predictor of fluid responsiveness in critically ill human patients [207–209] and in dogs [210], and is superior to CVP as a predictor of fluid responsiveness during open‐abdomen surgical procedures in humans [211] and to pneumoperitoneum in dogs [212]. While PPV variation requires more study in veterinary medicine, it has potential to provide more continuous and dynamic monitoring of patient volume status. Readers are referred to additional texts for more complete information on equipment and tools to monitor fluid responsiveness as well as other cardiac parameters [2, 213]. A common misconception is that most heart murmurs do not definitively require complete cardiac evaluation before anesthesia is planned, and that all patients with heart disease must be managed similarly when anesthetized. As with any anesthetic patient, patients with underlying cardiac disease should be evaluated with a complete history (detailing both the long‐ and short‐term changes in underlying disease), PE, and minimum database of blood work/urinalysis relevant for their signalment. In addition, patients with cardiac disease should be evaluated with thoracic radiographs, ECG, BP, and echocardiogram, which are aimed at not only documenting presence of disease, but also assessing the severity of disease and possible response to previous treatments. As one of the main goals of perianesthetic management is maintenance of homeostasis, especially perfusion and oxygen delivery, preanesthetic cardiac evaluation should include assessments for cardiac pump function. Valvular heart disease accounts for over 50% of congenital heart disease in dogs; chronic AV valvular disease is the most common cardiac disease in dogs, whereas mitral valve insufficiency is in the top three causes of cardiac disease in cats [214]. The prevalence of valvular disease in small animals necessitates a complete understanding of these comorbidities and how they affect and dictate the perianesthetic plan. Degenerative mitral valve disease is the most common cardiac disease in dogs, found in as much as 30% of the geriatric canine population [215]. dMVD is also referred to as myxomatous mitral valve degeneration, endocardiosis, degenerative valvular disease, and myxomatous degeneration; all terms describe the same constellation of pathophysiologic and clinical signs. In 2009, the American College of Veterinary Internal Medicine published a consensus for the diagnosis and treatment of myxomatous mitral valve disease in dogs, and the guidelines were updated in 2019 [216]. In this consensus, myxomatous mitral valve disease was categorized into stages A, B (B1, B2), C, and D: Stage A Stage B Stage C Stage D dMVD is grossly seen as an idiopathic development of nodular irregularities on the free edge of mitral valve leaflets. These nodules consist of deposition of mucopolysaccharides in the layers of the valve leaflet, which can increase in size and number over time. Pathophysiology of dMVD may also include distortion of the chordae tendineae such that they lengthen and/or thicken [217]. When valve changes are severe, this leads to curling of the valve leaflet and subsequent AV valve incompetence. The valve may degenerate to the point at which valve leaflets can prolapse into the left atrium. When valvular changes are sufficient that leaflets do not oppose one another during ventricular systole, it results in regurgitant flow of blood into the left atrium. Mitral valve regurgitation may be trivial or severe, and volume of regurgitation is dictated by the size of the space between the valve leaflets, the pressure gradient between ventricle and atria, and the duration of systole [218]. Mitral regurgitation leads to a volume overload on the left atrium, as pulmonary venous return is complemented by regurgitant flow. CO suffers as regurgitant flow increases, and the body compensates with renal, neurohormonal, and cardiac remodeling (left ventricular eccentric hypertrophy). High atrial pressures due to volume overload lead to atrial dilation, as well as increases in pulmonary vein pressures and congestion of pulmonary venous flow, which will eventually lead to pulmonary edema. CHF is the result of chronic volume overload to the left atrium and pulmonary veins with eventual failure of adaptive mechanisms. Dogs may present with lethargy, cough, exercise intolerance, weight loss, respiratory difficulty, or collapse [219]. Patients may also present with other complaints, and a murmur may be auscultated in a patient with no previous history of cardiac disease. It is these patients who often require a more cautious approach to evaluation and anesthesia planning; a lack of clinical signs may provide a false sense of security regarding potential anesthetic complications. The classic heart murmur for dMVD is a holosystolic murmur, loudest over the left apex of the heart. The intensity of the murmur tends to be consistent over the duration of systole, with no increase or decrease in the loudness of the murmur. Often, the second heart sound is inaudible. The murmur intensity is not correlated with the severity of regurgitant flow, but, in general, louder murmurs indicate worse regurgitation. Prolapse of the mitral valve may result in a mid‐systolic click. Anesthetic management can cover the spectrum from patients with fully compensated, nonclinical disease to those at high risk for heart failure. No consensus exists regarding the management of either end of this spectrum. Patients with stable, compensated disease with no left atrial enlargement and no clinical signs of pulmonary edema or heart failure generally do not require intensive management. As a portion of left ventricular ejection regurgitates into the left atrium, CO is compromised, and the patient with dMVD is thought to compensate for this with increases in HR [215]. Normal to high‐normal HRs are recommended for any signalment. Therefore, it is optimal to consider anticholinergics in the anesthesia plan, particularly if opioids are to be administered. Opioids are considered extremely safe for a patient with dMVD, as the cardiovascular effects are primarily limited to bradycardia, which is easily treated or prevented with the use of anticholinergics. Hypothermia should be avoided by using supplemental heat support to avoid hypothermia‐related bradycardia. Stable patients should tolerate inductions with ketamine and diazepam/midazolam. Alternatively, they should tolerate the dose‐dependent vasodilation with propofol or alfaxalone inductions; however, the induction agent dose should be minimized using preanesthetic sedatives and/or combining induction agents with benzodiazepines and/or opioids during induction. The severe negative inotropic effects and mild to moderate vasodilation associated with inhaled anesthetics can be minimized by additional use of local, regional, or systemic sedatives and analgesics. Opioids are well suited for this purpose. Alpha‐2‐adrenergic receptor agonists should be used with great caution due to the increase in afterload and the potential for increased regurgitant flow, as well as severe decreases in HR and CO. Unstable patients, such as those at significant risk for onset of heart failure or with a previous history of heart failure, those with arrhythmias, or those with preexisting cardiovascular compromise, must be handled with extreme caution. Pre‐induction stabilization of heart failure, hypotension, and arrhythmias must be attempted. Complete cardiac evaluation (PE, thoracic radiographs, ECG, BP, and echocardiogram) is optimal. Anesthetic management includes all efforts made to minimize or mitigate the cardiovascular compromise due to inhaled anesthetics and reliance on balanced anesthesia. As stated previously, alpha‐2‐adrenergic receptor agonists are not recommended in most cases. Sedation with opioids and benzodiazepines is useful, as they have minimal effects on cardiovascular function; opioid‐mediated bradycardia can be minimized or prevented with anticholinergics. Although optimal sedation is ideal, oftentimes, good sedation must be sacrificed in the name of cardiovascular stability, as induction of anesthesia is approached. Midazolam followed quickly by etomidate is a good induction choice because both have minimal to no cardiovascular side effects. Fentanyl and midazolam may also be used for induction, provided opioid‐associated bradycardias (and respiratory depression) are controlled and the patient is sufficiently sedated beforehand or is quite compromised. Alternatively, some patients may require induction with propofol despite the risk of dose‐dependent vasodilation and hypotension. In these cases, alfaxalone may be a better choice for induction in unstable patients, as it has greater cardiovascular stability when compared to propofol [94, 115, 118]. In these situations, reducing the dose of propofol with pre‐induction sedation and/or combining propofol with one (i.e., midazolam) or two (i.e., midazolam/fentanyl) additional induction drugs can minimize propofol dosage. Inhaled anesthetic concentration should similarly be minimized with additional local, regional, or systemic analgesics and sedatives. Monitoring in patients with severe mitral valve disease should include either Doppler NIBP or IBP monitoring. Mitral valve stenosis is a rare finding in dogs, with only 12 reported cases in a 10‐year period [220] and 12 diagnosed cases in a cohort of 1779 patient records [221]. Stenotic lesions may involve the valve annulus, leaflets, chordae tendineae, or papillary muscles and present as a valvular or supravalvular lesion [222]. The heart murmur associated with MVS is a mid‐diastolic low‐frequency murmur and possibly a split‐second heart sound (S2). The stenotic lesion creates a pressure gradient across the valve and leads to an increase in left atrial pressure, which is transmitted to the pulmonary vasculature and can lead to pulmonary edema with severe stenosis [214]. Diagnosis of MVS may be made only when a patient presents with left heart failure and the defect is identified with echocardiography. The treatment for MVS is frequently medical, as surgical options are extremely high risk and should only be considered when all alternatives have been exhausted. The goal of medical intervention is to manage signs of left‐sided heart failure and to decrease left atrial pressure and signs of left heart failure with diuretics and angiotensin‐converting enzyme (ACE) inhibitors. Sodium restriction is recommended. Additional treatment may be required for supraventricular arrhythmias such as atrial fibrillation or supraventricular tachycardia (SVT). Anesthetic management largely depends on the severity of clinical presentation [223, 224]. The anesthetic goal is to prevent any situation in which CO is significantly impaired or the patient is put at risk for the development of pulmonary edema. With MVS, CO can be decreased by multiple mechanisms. For example, as MVS worsens in severity, ventricular filling depends increasingly on diastolic filling time and right atrial pressure. Tachycardia or tachyarrhythmias will decrease diastolic filling time and worsen ventricular filling and subsequently CO. Loss of association between atrial depolarization or the atrial kick boosting end‐diastolic volume (10–30% of end‐diastolic volume) and ventricular contraction/ejection will worsen CO. Therefore, arrhythmias affecting AV coordination should be treated rapidly in these cases. Atrial fibrillation and SVT can develop while anesthetized and the ECG should be evaluated before and through anesthetic induction. Acute vasodilation and decreases in atrial preload may worsen ventricular filling, as the normal response to acute hypotension is tachycardia. Finally, the pressure overload to the pulmonary vasculature from MVS can precipitate pulmonary edema [225]. Avoidance of increases in blood volume that can precipitate CHF is strongly recommended. Patients with mild MVS can likely be managed with any anesthetic plan except for high dosages of ketamine and tiletamine. Both dissociative agents can increase catecholamine release, which increases sympathetic tone, potentially leading to tachycardia and increases in myocardial contractility. If diastolic filling time decreases significantly, CO can drop precipitously. Similarly, tachycardia from patient stress, anxiety, and pain can decrease CO. ACE inhibitors should be withheld for 24 h to reduce the potential for hypotension (see the section titled “Systemic Hypertension”). Moderate to profound preanesthetic sedation is optimal to prevent tachycardia. Opioids and benzodiazepines are attractive options, as they do not significantly decrease HR, contractility, or vascular tone. While some opioids are good sedatives, benzodiazepines are inconsistent sedatives in small animals and can precipitate mild dysphoria or excitement in dogs, and undesirable behavioral changes including potential aggression in cats [65, 67]. Anticholinergic agents are controversial in that they can precipitate tachycardia. However, anticholinergics can be indicated if there is a preexisting bradycardia or second‐degree AV block. Patients with mild disease can likely tolerate the vasodilation associated with propofol for induction, but combination with an opioid (propofol–fentanyl) or benzodiazepine (propofol–midazolam) is recommended to reduce the total dosage of propofol. As mentioned in the earlier text, alfaxalone may provide increased cardiovascular stability compared to propofol. If patients have severe disease with significant cardiovascular compromise, anesthetic induction can be achieved with etomidate or fentanyl in combination with a benzodiazepine. Patient monitoring with MVS also depends on their degree of disease and anticipated complications. Patients with mild disease can be monitored as for any patient. Patients with advanced disease may require IBP monitoring, arterial blood gas assessment to evaluate pulmonary function, and CVP/PPV measurement. Patients with severe disease may warrant referral to specialty centers for consultations with veterinary cardiologists and anesthesiologists for management. Tricuspid valve stenosis as an independent finding is rare in small animals, and tricuspid valve incompetence is far more often due to tricuspid valve dysplasia or is the result of underlying cardiac disease. Like MVS, it may result from abnormalities of the annulus, valve leaflets, or papillary muscles. Labradors or breeds at risk for AV valve disease such as Newfoundlands and Bull Terriers may be at higher risk [167]. Should TVS be identified as an isolated finding, recommendations for anesthetic management are the same as MVS. Given the rarity of isolated TVS, other causes for tricuspid valve incompetence must be investigated in patients who have suspicion of tricuspid valve disease. Aortic stenosis is the most common congenital cardiac disease of large breed dogs such as Boxers, Great Danes, Rottweilers, Golden Retrievers, German Shepherds, English Bulldogs, and Bouvier des Flandres, and has been described as heritable in Newfoundlands [214]. AS can be due to valvular, supravalvular, and subvalvular lesions (SAS); however, SAS is the most common finding in dogs representing more than 95% of lesions identified. The site of SAS is the left ventricular outflow tract (LVOT), which comprises the membranous and muscular portions of the basilar interventricular septum, the craniolateral left ventricular free wall, and the anterior mitral valve leaflet. Aortic stenosis can be described as fixed or dynamic. Fixed SAS is due to an anatomic abnormality creating the stenotic lesion; the severity of the obstruction does not change with rate or velocity of flow through the area. Fixed SAS has been graded in cadavers depending on the anatomic findings. For example, Grade 1 has minor changes (endothelial nodules) in the subaortic endocardial surface, Grade 2 has a narrow fibrous band around part of the LVOT, and Grade 3 has a complete band of tissue surrounding the entire LVOT [226]. Dynamic SAS is an obstruction in the LVOT that changes based on the flow rate through the subaortic outflow tract. Increases in HR or cardiac contractility lead to a decrease in intraluminal pressure (based on the Bernoulli principle) and an increase in the degree of LVOT obstruction (LVOTO). Dynamic SAS is most identified in HCM and can be referred to as hypertrophic obstructive cardiomyopathy (HOCM). The principal hemodynamic consequence of outflow tract obstruction is an increase in resistance to systolic ejection of blood from the ventricle, thereby decreasing flow through the outflow tract, increasing pressure across the stenosis, or both [227]. Left ventricular pressure is increased and results in compensatory concentric hypertrophy to maintain left ventricular output. Blood ejection through the stenotic area results in turbulence during systole and a resultant systolic murmur, typically described as an ejection murmur that increases and then decreases (crescendo–decrescendo) [228]. SAS and LVOTO along with the left ventricular hypertrophy typically do not lead to left‐sided heart failure. However, left ventricular hypertrophy, a decrease in capillary density, and increased wall tension predispose to myocardial ischemia. Patients who develop this pathophysiology are at risk for syncope, ventricular arrhythmias, and sudden death, although it is unclear which of these is the definitive cause of death. Another possible explanation for sudden death is exercise‐induced increases in left ventricular pressure (in addition to pathologically high resting left ventricular pressure) and activation of ventricular mechanoreceptors that lead to vasodilation and bradycardia; the Bezold–Jarisch reflex [224]. Aortic valve leaflet damage from high‐velocity regurgitant jet flow can predispose the valves to bacterial endocarditis associated with bacterial shower from surgical or dental procedures or from concurrent noncardiac infection‐causing bacteremia. Prophylactic antibiotics are recommended for all anesthetized procedures to minimize the risk of endocarditis. Anesthetic management in patients with SAS can become very complicated and requires intensive monitoring and antiarrhythmic treatments to maintain normal ventricular filling and optimal CO. Patients should remain in a normal sinus rhythm without sinus tachycardia or bradycardia, and one should be prepared to treat ventricular ectopy or atrial fibrillation. For example, left ventricular CO is dependent on the organization of atrial and ventricular contraction. Thus, AV blocks or atrial fibrillation will lead to loss of the atrial kick and reduction of left ventricular end‐diastolic volume. Sinus tachycardia prevents diastolic filling time and should be avoided. Sinus bradycardia leads to poor CO and hypotension, subsequently leading to poor coronary and myocardial perfusion in a thickened left ventricle. Thus, HR should be kept in the normal range to prevent decreased tissue perfusion. Ventricular premature complexes (VPCs) cause contraction before complete ventricular filling and lead to a decrease in CO. Prompt treatment of ventricular ectopy is important, as the presence of ventricular rhythms can be a risk factor for sudden death [229]. Premedication in patients with moderate to severe AS should provide adequate sedation/analgesia to prevent anxiety, pain, or stress‐related tachyarrhythmias. Anticholinergic agents are indicated to prevent bradycardia and AV blocks. The administration of acepromazine is controversial due to long‐acting vasodilation but may be tolerated in the minimally affected patient if anxiety is present and tranquilization is required. Anesthetic drugs that should be used extremely cautiously (if at all) in AS include high‐dose dissociative anesthetics (ketamine and tiletamine) and alpha‐2‐adrenergic receptor agonists (xylazine, medetomidine, and dexmedetomidine). High‐dose anticholinergic agents are controversial in that it is difficult to predict the maximum HR a patient may develop in response to a typical dose of anticholinergic. It is worth considering titrating lower doses of anticholinergic agents for patients developing sinus bradycardia and assessing the response to low doses, rather than risk inducing tachyarrhythmias with standard or high doses of atropine or glycopyrrolate. Anesthetic induction can be accomplished with propofol, alfaxalone, etomidate, or opioid–benzodiazepine combinations. Pulmonic stenosis is rarely identified in cats but is the third most common cardiac defect identified in dogs [230]. Pulmonic valvular stenosis is the most common abnormality, with supravalvular and subvalvular stenosis being less common. Pulmonic stenosis is commonly identified as a sole lesion in dogs but can be identified in combination with other cardiac abnormalities such as tetralogy of Fallot (TOF). It is heritable in Beagles and Keeshonds, and a variety of breeds have increased risk for PS, including English Bulldogs, Cocker Spaniels, Mastiffs, Samoyeds, Miniature Schnauzers, Chihuahuas, and Chow Chows [230]. Pulmonic stenosis is due to variable congenital deformations and fusion of pulmonic valve leaflets. The degree of anatomical change and severity of pulmonary outflow tract obstruction are graded as either Grade 1 with minimal to mild fusion and trivial to mild outflow obstruction or Grade 2 that has moderate to severe valve deformation and fusion, with severe outflow obstruction [231]. Severe obstruction leads to poststenotic main pulmonary artery dilation in response to the turbulent blood flow downstream from the affected valve. The primary pathophysiologic hemodynamic effect of PS is an increase in resistance to right ventricular systolic ejection, leading to increased right ventricular pressure. This pressure overload leads to concentric hypertrophy of the right ventricle as a compensatory response, thereby returning right ventricular output to normal or near normal. The degree of pressure overload is related to the pressure gradient across the pulmonic valve and is correlated with disease severity. Right ventricular hypertrophy decreases right ventricular compliance, reduces ventricular filling, and leads to an increase in right atrial pressure. This mechanism also underlies the tricuspid regurgitation in patients with tricuspid dysplasia (whether preexisting or because of change in right ventricular size), which can result in right heart failure, jugular distension/pulsations, ascites, and pleural effusion. Definitive anesthetic recommendations for mild PS are lacking, but patients with clinically insignificant PS can likely be anesthetized with any technique. Intravenous crystalloid administration during anesthesia should be limited (2–5 ml kg−1 h−1), and synthetic colloids should be avoided, as volume overload and right heart failure could be a concern, particularly in patients with concurrent tricuspid dysplasia. Patients with severe PS should be treated cautiously, and anesthetic management should be designed to minimize cardiovascular depression. Alpha‐2‐adrenergic receptor agonists are contraindicated due to the significant increase in right atrial pressure. Acepromazine is controversial due to the long lasting, irreversible vasodilation, hypotension, and reduced right atrial preload. However, opioids for patient analgesia and sedation as well as anticholinergics to prevent or treat opioid‐associated bradycardia are recommended. Induction agents that preserve cardiac function and CO are preferred; combinations of etomidate or fentanyl with benzodiazepines have minimal cardiovascular depression, provided patients do not become bradycardic. Dissociative anesthetics are controversial and may be contraindicated in severe PS due to increases in SVR. Inhaled anesthetic doses should be minimized by opioid infusions to minimize vasodilation and significant decreases in contractility. Local and regional anesthetic techniques should be employed to reduce systemic and inhaled anesthetic requirements. Monitoring should consist of standard ECG, SpO2, temperature, and capnography, as well as IBP management to allow observation in minute‐to‐minute changes in patient status. Congenital cardiac defects can be grouped according to their pathophysiologic mechanisms [232]. The most common examples include those with left‐to‐right shunting of blood and volume overload (PDA and VSD), pressure overload (PS and SAS), and those which present for cyanosis (TOF, PDA, and VSD with shunt reversal [right‐to‐left]). Patent ductus arteriosus is one of the most common congenital abnormalities identified in dogs but is rare in cats. Predisposed breeds include the Chihuahua, Bichon Frise, Collie, Cocker Spaniel, Keeshond, Maltese, Miniature Poodle, Pomeranian, and Yorkshire Terrier, among others [233]. The defect is genetic in the Miniature Poodle. The ductus arteriosus is a fetal structure that allows shunting of blood (80–90% of total flow) from the pulmonary artery to the aorta necessary to avoid blood flow through the high vascular resistance of the fetal lungs. Increases in oxygen tension in the ductus with neonatal ventilation should lead to constriction of the ductus and closure within the first week of life [234]. A PDA is the persistence of this fetal structure in the neonatal and juvenile patient. Morphology of a PDA can vary from a diverticular, funnel‐shaped structure to a cylindrical tube between the descending aorta and main pulmonary artery [235]. Blood flow for a left‐to‐right shunt is determined by the diameter in the shunt and the pressure gradient between the aorta and pulmonary artery [236]. Left‐to‐right shunting of blood occurs continuously in patients with PDA throughout the cardiac cycle because of higher systemic BP as compared to pulmonary BP and is a function of relative resistances in the aorta as compared to pulmonary vascular resistance. This leads to constant volume overload of the left ventricle, as shunt flow is added to normal pulmonary venous return. The volume overload leads to atrial and ventricular dilation and ventricular hypertrophy. When pulmonary vascular resistance increases and exceeds SVR, shunt flow will reverse and become right‐to‐left, leading to clinical cyanosis, as shunt flow does not circulate through the lungs [234]. Due to the risk of shunt reversal, cyanosis, development of heart failure, arrhythmias, and other complications, it is recommended that individuals intimately familiar with the pathophysiology of disease and the surgical/interventional procedures required to ligate/occlude PDA shunts anesthetize patients with PDA; evaluation and treatment by veterinary specialists trained to safely handle these patients are strongly recommended. Anesthetic factors to consider are primarily directed at the patient’s age and size. Neonatal or juvenile physiology leads to a variety of unique pharmacokinetic and pharmacodynamic alterations (see Chapter 19). For example, the cardiovascular system has lower myocardial contractile mass, low cardiac reserve, and high cardiac index; CO is HR‐dependent primarily due to poor vasomotor control [237]. The ability to increase contractility or vasoconstrict may be reduced, and treatments aimed at increasing contractility or providing vasoconstriction may be reduced or ineffective. The respiratory system is very compliant with high elastic forces, leading to increased airway resistance. Respiratory rates and minute ventilation are greater than in the adult. The hepatic and renal systems are immature and drug effects may be pronounced or prolonged in these patients. Young patients have high body weight to surface area ratios and will lose body heat rapidly. Thus, heat support is critical in these patients. High body water content may lead to lower than adult packed cell volume (PCV) and albumin levels. Blood glucose regulation may be impaired; patient fasting should be shorter than adults, and supplemental dextrose may be warranted [237]. Patient size may present special challenges because patients are usually quite small at presentation for PDA ligation or coil placement, and patient size has been identified as a risk factor for anesthetic‐related mortality. Placement of monitoring equipment may be difficult, and the ability to access the patient during surgery may be limited. Venous catheterization may be challenging, and access tubing should be confirmed and clearly labeled if the patient cannot be clearly visualized. Anticipated surgical or procedural complications can include hemorrhage, as well as complications from thoracotomy (pain, hypoventilation, hypoxia, ventilation/perfusion imbalance, etc.). Interventional catheterization carries the risk of hemorrhage, migration of the occlusive device into the pulmonary artery, and failure to occlude the PDA with conversion to thoracotomy. Anesthetic drug selection is generally dictated by the unique neonatal/juvenile physiology; short‐acting, reversible drugs are recommended. For example, a combination of opioids, benzodiazepines, and anticholinergics is frequently used for sedation, analgesia, and HR support. Examples for premedication may include hydromorphone, oxymorphone, methadone, or morphine with or without atropine. In very young patients, additional sedation may be achieved with midazolam or diazepam. Anticholinergics are recommended, as CO is HR‐dependent, and bradycardia can lead to significant hypotension. Acepromazine is not recommended, as juvenile patients are relatively more vasodilated than adults, have difficulties in increasing cardiac contractility and SVR, and diastolic runoff through the PDA leads to very low DAPs. Induction agents including ketamine, etomidate, and fentanyl/benzodiazepine are recommended. Propofol is not recommended as a sole induction agent because of the dose‐dependent vasodilation, though alfaxalone may provide improved cardiovascular stability and maintenance of baroreceptor reflexes [94]. Maintenance anesthetic requirements can be reduced by concurrent infusions of opioids and/or lidocaine. IBP monitoring is recommended, although Doppler BP measurement is a reasonable alternative, given the difficulty of arterial catheter placement in these patients. Regional anesthesia including intercostal or intrapleural nerve blocks can be considered as a part of a balanced analgesic plan for patients undergoing thoracotomy. Intercostal nerve blocks are performed by injection of local anesthetic along the caudal margin of the ribs, dorsal to the length of the incision. Injection of two to three intercostal spaces cranial and caudal to the incision location is recommended for lateral thoracotomy [238]. Total dose of lidocaine or bupivacaine is recommended to not exceed 2 mg kg−1, as the highest plasma concentration of local anesthetics is seen after intercostal nerve blocks, suggesting significant drug uptake from intercostal injection [239]. Postoperative intrapleural analgesia can also be obtained by direct intrapleural infiltration of local anesthetic, again, not exceeding the 2 mg kg−1 maximum dose. Typically, bupivacaine is chosen for these regional techniques due to the longer duration of action compared to lidocaine. Intraoperative glucose monitoring is recommended and supplemental dextrose at 1.25–2.5% in maintenance IV fluids can be considered as needed. Anesthetists should be aware and prepared for observing the Branham reflex, the decrease in heart rate, and increase in blood pressure immediately after PDA ligation [240]. Additional complications including hemorrhage, arrhythmias, hypothermia, and others are treated as needed. Should an Amplatzer ductal occluder be the treatment option of choice, the anesthetist should be aware of the risk (3% of cases) of device migration from the PDA into the main pulmonary artery and lungs [241]. Thus, anesthetic recovery is a critical time for PDA occluder placement, as a poor or rough recovery may dislodge the device in the PDA. Reducing the risk for dysphoria from benzodiazepines or opioids may require carefully titrated reversal with flumazenil and/or naloxone, respectively. Butorphanol may also be used to reverse mu‐opioid agonist effects while still providing some analgesia/sedation through kappa‐opioid receptors. Similarly, titrating down inhalant doses toward the end of the anesthetic event may reduce the risk of emergence delirium. Vital signs including temperature, HR, and BP should continue. Postprocedural sedation with injectable acepromazine, benzodiazepines (dependent on patient temperament), or very low doses of alpha‐2‐adrenergic receptor agonists may be required. All care must be aimed at a smooth recovery to prevent device dislodgement. Tetralogy of Fallot is a common congenital anatomic malformation, which can cause cyanosis, polycythemia, stunted growth, exercise intolerance, collapse, and seizures [242]. Predisposed breeds include the English Bulldog, Keeshond, Miniature Poodle, and Schnauzer, as well as other dog breeds and cats. TOF is a combination of four anatomic derangements as follows: (i) VSD, (ii) dextrorotation with an overriding aorta, (iii) PS, and (iv) right ventricular hypertrophy due to obstruction in the right ventricular outflow tract (RVOT) [243]. Hemodynamic alterations are dependent on the degree of shunt through the VSD and the consequences of the PS. If PS is mild and resistance to RVOT flow is mild, then right ventricular pressures should be lower than left ventricular pressures and flow should be left‐to‐right [230, 243]. If PS is severe and represents significant outflow obstruction, then elevated right ventricular pressures can shunt blood right‐to‐left and lead to clinical cyanosis. Clinical cyanosis results in erythropoietin release and secondary polycythemia. Polycythemia (PCV >70–75%) can lead to increased blood viscosity and poor perfusion due to sludging of blood flow [244]; patients may seizure because of polycythemia. The primary consideration for anesthesia in a patient with TOF is maintenance of normal systemic BPs to prevent reduction in left ventricular pressure. Decreases in left ventricular pressure can lead to shunt reversal (i.e., right‐to‐left) if right ventricular pressures are higher than left ventricular pressures. Right ventricular desaturated blood can subsequently enter the systemic circulation, resulting in cyanosis and decreased oxygen delivery. Premedication, induction, and maintenance anesthetic agents should be selected to prevent systemic hypotension as much as possible. Due to their vasodilatory effects, avoiding or minimizing dosages of propofol and inhaled anesthetics is recommended. Alfaxalone can be considered in place of propofol for potential improved cardiovascular stability. Opioids are a mainstay of anesthetic management used to reduce inhaled anesthetic requirements. BP should be supported, and hypotension rapidly treated to prevent further right‐to‐left shunting; this may include positive inotropic agents such as dopamine or dobutamine, or vasopressors such as phenylephrine or norepinephrine. IBP monitoring is recommended for patients with significant cyanosis or right‐to‐left shunting for both gold‐standard monitoring of systemic BP and arterial blood gas sampling in the event of desaturation. Preoxygenation and postoperative oxygen supplementation are strongly recommended. Ventricular septal defects represent the failure of complete development of the membranous or muscular interventricular septum. They are more likely seen in Keeshond and English Bulldogs but have been identified in many dog breeds [245]. The incidence in cats is unknown, but the prevalence in both dogs and cats is low. VSDs can vary in size and pathophysiology, and clinical presentation depends on the degree and direction of shunting. Simple VSDs show left‐to‐right shunting in both phases of the cardiac cycle, and volume of flow is dependent on the shunt diameter [245]. Small‐to‐medium size defects exhibit resistance to flow across the VSD, which typically minimizes the increase in right ventricular volume and does not result in increases in pulmonary circulation or pulmonary pressures. Large VSDs that do not cause resistance to flow across the VSD lead to pulmonary overcirculation and pulmonary hypertension (PHT). PHT can then increase right ventricular pressure and, if higher than left ventricular pressure, may lead to right‐to‐left shunting and clinical cyanosis. Increased pulmonary blood flow leading to increased left ventricular preload can lead to left ventricular hypertrophy and pulmonary edema because of the inability of the left ventricle to eject the increased pulmonary venous return. Similar to TOF, anesthetic management is directed at preventing right‐to‐left shunting by maintenance of systemic BP. Anesthetic plans should be designed to minimally impact BP, and rapid support for hypotension should be available with positive inotropic agents (dopamine and dobutamine) or vasopressors (phenylephrine and norepinephrine) if needed. Monitoring and interventions for patients are like those for TOF. The importance of a normal cardiac rhythm cannot be overstated. The essential function of the cardiovascular system is to provide tissues with oxygen and nutrients while removing the waste products of metabolism. More than any other, the one essential micronutrient that the body cannot survive without is oxygen. As previously discussed, oxygen delivery is the product of CO (l min−1) and CaO2 (ml O2 dl‐1 blood). CaO2 is the sum of oxygen bound by saturated hemoglobin and that found dissolved in blood (PaO2). Cardiac output is the product of HR and SV (ml blood ejected per heartbeat). To maximize CO and optimize oxygen delivery, the contraction and relaxation of the heart must be sufficiently coordinated to allow diastolic ventricular filling and systolic ejection of blood. Cardiac arrhythmias, by definition, are disorganizations of the coordinated electrophysiologic and mechanical function of the heart and can rapidly lead to life‐threatening reductions in CO and perfusion [246]. Identification and treatment of arrhythmias are critical components of patient management before and during anesthetic events, as well as into the recovery period. The behavior of electrical impulses and of the cardiac rhythm is determined by the shape of the action potential. The ECG is the electrical representation of the summation of all cardiac vectors measured in standard Lead I, II, or III configurations at the limb electrodes placed on the patient, graphed in voltage versus time. Changes in the shape of the cardiac action potential or ECG are determined by shifts of ions, particularly sodium, potassium, and calcium across the cardiac myocyte cell membrane. The movement of ions is determined by cell surface receptors and the electrochemical gradients of these ions across the membrane, and is extensively reviewed elsewhere [246]. The cardiac action potential is described in four phases, labeled as Phase 0 through 4, during the progression of the cardiac cycle (Figure 1.10) [246]. Phase 4 represents the resting phase and is described by the resting membrane potential (RMP), the voltage measured across the myocyte cell membrane during the unstimulated state. RMP varies by the type of myocyte; specialized myocytes such as the sinoatrial (SA) nodal cells (Figure 1.10) and AV nodal cells have a different RMP as compared to a nonspecialized working cardiac myocyte. The transmembrane RMP measured in the generic cardiac myocyte is −90 mV but can vary from −50 to −90 mV depending on the type of cardiac myocyte. In Phase 4, the cell membrane is relatively permeable to potassium (inwardly rectifying potassium current, IK1) and impermeable to sodium and calcium; therefore, the RMP is determined mostly by potassium, as it moves out of the cell along the electrical and chemical gradients. The term “resting state” is somewhat misleading, as the RMP is also an active process due to the action of the basolateral Na+‐K+‐adenosine triphosphatase (ATPase) pump, which actively moves sodium out of the cell against the concentration gradient. Phase 0 is characterized by myocyte cell membrane depolarization due to the rapid influx of sodium through rapidly opening voltage‐gated sodium channels, down the electrochemical and concentration gradients such that the transmembrane potential reaches a positive value of approximately +30 mV [247]. The polarity reversal to positive in the cell membrane potential opens the L‐type calcium channels, allowing the onset of inward calcium conductance that becomes important through Phase 2. The slope of Phase 0 represents the speed of depolarization of a single myocyte, and as conduction of the action potential through one myocyte dictates conduction to adjacent myocytes and spread of the action potential, the slope of Phase 0 determines conduction velocity through the heart. Pathologic states that slow sodium influx during Phase 0 reduce the speed of conduction of single myocytes through the heart and can be the origin of cardiac arrhythmias or reentry circuits. Phase 1 results from the return of the RMP toward neutral due to the inactivation of Phase 0 voltage‐gated sodium currents, the onset of inward calcium movement through L‐type calcium channels, as well as transient inwardly rectifying potassium currents via voltage‐gated potassium channels (I‐to). Phase 2 is the sustained depolarization of the cardiac myocyte termed “the plateau phase,” which is a unique feature in electrically excitable tissues. The plateau is the balance of the inward calcium movement via L‐type calcium channels and the outward potassium movement through a sodium/potassium exchanger current. Figure 1.10 Representative myocardial action potential tracings of a ventricular myocyte (top) and SA nodal myocyte (bottom). Phase 4: resting membrane potential. Phase 0: rapid depolarization. Phase 1: initial repolarization. Phase 2: plateau phase. Phase 3: repolarization. Phase 3 is the final repolarization of the cardiac myocyte and is primarily due to the increase in outward potassium conductance across the cell membrane via multiple slow, rapid, and delayed rectifier currents. At the same time, conductance of sodium and calcium decreases, allowing overall net movement of positive charge out of the cell and reestablishment of RMP at −50 to −90 mV. Another mechanism of arrhythmia generation is the reduction in overall potassium outward movement during Phase 3 in the failing heart, leading to events such as early afterdepolarizations. After the end of Phase 3, the cardiac myocyte enters a refractory period, wherein further stimulation cannot result in the generation of an action potential. The refractory period allows the heart to relax during diastole and the ventricles to reach an appropriate end‐diastolic volume such that when the refractory period ends, and the heart is again able to contract, a normal volume of blood is ejected, and SV/CO is maintained. The refractory period prevents cardiac tetany and depolarization of one myocyte from the adjacent one. This appropriately propagates the action potential in one direction, rather than allowing the passage of an action potential between two adjacent myocytes. The duration of the refractory period is roughly that of the action potential, such that the myocyte cannot be restimulated until the end of Phase 3. The refractory period can be divided into an (early) absolute refractory period, wherein no degree of stimulation can lead to depolarization, and a (later) relative refractory period, wherein a higher‐than‐normal stimulus has the potential to depolarize the myocyte. Cardiac arrhythmias can be classified based on the electrophysiologic mechanism underlying the generation of the abnormal rhythm. These mechanisms include disorders of impulse generation, impulse conduction, and combined disorders. Specific arrhythmias of these classes are separated into sinus, supraventricular, and ventricular origin arrhythmias (Table 1.8) [243]. The normal ECG waveform is generated from the coordinated conduction of the action potential from the SA node across the atria to the AV node, through the AV node to the bundle of His, and into the ventricular Purkinje system. The conduction is subsequently carried rapidly through the ventricles, leading to coordinated muscular ventricular contraction. Ventricular repolarization is the terminal event of a single ECG complex. Atrial depolarization is seen as the P wave. P wave amplitude and duration can vary with changes in body position relative to electrode position, vagal tone, and with arrhythmias or cardiac disease. Action potential conduction through the AV node is represented by the P–R interval. Shortened P–R intervals can be due to accessory atrial pathways increasing rate of atrial conduction. P–R interval prolongation is the classic finding for first‐degree AV block. Atrial repolarization occurs during ventricular depolarization and is not seen on the ECG waveform. The QRS complex is formed from ventricular depolarization (ventricular septum, left and right ventricular free walls; Figure 1.11). The S–T segment is the time between the end of ventricular depolarization and beginning of ventricular repolarization. Both S–T segment elevation and depression can be abnormal findings; elevation can be due to myocardial hypoxia, pericardial effusion, or digoxin toxicity (cats), and S–T segment depression can be due to hypoxia, hyperkalemia or hypokalemia, infarction, or digoxin toxicity [248]. Ventricular repolarization occurs after the QRS complex and is represented by the T wave. Table 1.8 Cardiac arrhythmia classification by mechanism. Clinical evaluation of the ECG during anesthesia is typically performed in a Lead II arrangement. Each lead arrangement has a positive and a negative electrode, described by Einthoven’s triangle (Figure 1.12). The principal cardiac vector during normal sinus rhythm is from the SA node to the left ventricular free wall. Lead II ECG (negative right arm to positive left leg) measures parallel to this vector, typically resulting in the largest amplitude ECG waveform. Figure 1.11 Representative Lead II ECG tracing to identify named peaks. The P wave represents atrial depolarization. The P–R interval represents AV nodal delay in conduction. The QRS complex represents ventricular depolarization. The T wave represents ventricular repolarization. The R–R interval determines overall heart rate and is evaluated for regularity during ECG assessment. Figure 1.12 Einthoven’s triangle illustrates electrical measurement vectors and polarity through the heart based on lead selection. A Lead II ECG shows the highest‐amplitude ECG tracings as Lead II parallels electrical potentials from the SA node to the AV node and through the His–Purkinje system toward the ventricular myocardium. For this reason, Lead II is the most common ECG tracing used during general anesthesia. Evaluation of the ECG for arrhythmias requires a systematic approach to ensure arriving at an accurate diagnosis, and it is a common mistake to interpret the ECG and diagnose an arrhythmia with an “at‐a‐glance” approach. The following variables should be evaluated to identify ECG abnormalities. Heart rate will be averaged by the patient monitor over a specific duration of time, typically 6–10 s. Although this may be sufficient for some arrhythmias, manual calculation of the HR, whether an average over time or a calculated instantaneous rate (HR between two consecutive complexes), is recommended, as a patient monitor can incorrectly calculate HR with irregular rhythms or very tall/short complexes. Heart rate should never be taken from the ECG due to such inaccuracies. Heart rate analysis allows classification of tachyarrhythmias and bradyarrhythmias, which are species dependent. The anesthetist must ensure that there is a Q‐R‐S present for every P wave and a P wave for every Q‐R‐S complex. P waves not followed by a Q‐R‐S are typical of second‐ and third‐degree AV block. In small animals, the P wave has a positive deflection. Rounded P waves indicate an abnormality in the SA node, and P waves of different or variable morphology may represent ectopic atrial contractions. An absence of P waves is seen with hyperkalemia, atrial standstill, atrial fibrillation, or P waves lost within a dissociated Q‐R‐S complex (third‐degree AV block). Inverted P waves (negative in Lead II) indicate that the P wave origin is near the AV node and travel toward the Lead II negative electrode (right arm). Rhythm regularity is assessed by measuring the R‐to‐R interval between two successive Q‐R‐S complexes, most easily accomplished with calipers, though the edge of a piece of paper with markings or a ruler may suffice. A regular rhythm is one in which the R‐to‐R interval is consistent. Regular rhythms with a consistent R‐to‐R interval include normal sinus rhythm, sinus tachycardia, sinus bradycardia, SVT, and ventricular tachycardia. Irregularity can be described as “regularly irregular” where there is irregularity with a pattern (e.g., AV blocks, sinus arrhythmias, and wandering pacemakers), or as “irregularly irregular” where there is no pattern to the rhythm (e.g., atrial fibrillation). Usually, the faster the rhythm, the more difficult it is to detect regularity or irregularity. Printing ECG strips at faster paper speeds (e.g., 50 mm s−1) can assist in unmasking irregularity if difficult to determine compared to slower paper speeds (e.g., 12.5 or 25 mm s−1). Q‐R‐S complex morphology can aid in identification of supraventricular or ventricular origin waveforms. Ventricular origin ectopic complexes rarely conduct through the Purkinje system, and therefore the wave of depolarization must spread cell to cell. Cell‐to‐cell conduction is far slower (approximately 1 m s−1) compared to conduction rates of the Purkinje system (approximately 100 m s−1), and therefore the ECG trace of an ectopic ventricular complex is “wide and bizarre”. Narrow‐complex Q‐R‐S morphology is consistent with supraventricular origin complexes that pass through the AV node and spread via the Purkinje fibers and lead to extremely rapid (and therefore narrow) Q‐R‐S complexes. Periodicity is the frequency of an arrhythmia and is described as either a sustained, incessant abnormality or nonsustained, paroxysmal rhythm. The term paroxysm is often reserved for an arrhythmia that converts from totally normal to totally abnormal between two Q‐R‐S complexes, for example, the sudden, acute paroxysm of SVT or ventricular tachycardia. Sinus arrhythmia is the most seen regularly irregular sinus rhythm during the respiratory cycle, wherein HR increases with inspiration and decreases with expiration [248]. This is due to changes in underlying vagal tone through the respiratory cycle and the effect of changing vagal tone on HR. It is common in the canine but is abnormal in the feline. If required, treatment is directed at increasing sympathetic tone or normalizing HR if bradycardic (usually with an anticholinergic agent). Owing to the effects of premedication and induction agents and subsequent effects on parasympathetic tone, sinus arrhythmia can be a normal finding while anesthetized. A wandering pacemaker is a sinus rhythm with variation in the origin of the P wave within the SA node and is likely due to variable vagal tone to the SA node [249]. It is seen as a cyclic variation in P wave configuration amid a normal sinus rhythm. The P wave may occasionally be isoelectric and therefore undetected on the ECG trace. Sinus arrest is the failure of the SA node to produce a depolarization and subsequent P–Q‐R‐S–T complex due to severely depressed automaticity of the SA node [250]. For a diagnosis of sinus arrest, the R‐to‐R interval of the period of arrest must be a minimum of twice the R‐to‐R interval of the underlying sinus rhythm. However, pauses of 5–12 s are not impossible and can be terminated by an escape ventricular complex, junctional escape complex, or sinus complex. Sinus arrest can result in clinical signs of weakness or syncope. Possible causes of sinus arrest include carotid sinus or ocular stimulation, SA nodal fibrosis, drug effects (digoxin, β‐adrenergic blockers), or hyperexcitability of the vagus nerve (“vagotonia”) with intrathoracic or cervical mass manipulation. Treatment of sinus arrest can include termination of the stimulating cause and attempts at anticholinergic therapy; however, if severe, mechanical pacemaker implantation may be required. Sinus bradycardia is a normal sinus rhythm of lower‐than‐normal expected rate. Assessment of normal HR varies with species and breeds. It may be a normal finding in a very calm, athletic, or sleeping patient, but can also be a consequence of drug therapy (e.g., opioids, alpha‐2‐adrenergic receptor agonists, propofol, β‐adrenergic blockers, calcium channel blockers, and digoxin), pathophysiologic (e.g., hypothyroidism and hypothermia), or because of cardiac disease (e.g., sick sinus syndrome [SSS]) or elevations in vagal tone. Treatment for sinus bradycardia is recommended if there are signs of reduced perfusion, CO, or BP. If no contraindications exist, a bradycardic animal that is hypotensive should have the HR increased with anticholinergic therapy to improve CO and BP before other treatments to improve BP are attempted. Sinus tachycardia is a sinus rhythm more than the normal range, typically HR >160 beats min−1 in dogs and >200–220 beats min−1 in cats [251]. It can be a normal physiologic response to pain, stress, or anxiety, due to drug overdoses of anticholinergics, catecholamines, or their derivatives (i.e., positive inotropes such as dopamine and dobutamine), or thyroid over‐supplementation. Pathologic sinus tachycardia can be seen with pain, hyperthyroidism, fever, shock, CHF, and early stages of hypoxia (hypoxic, ischemic, hypemic, and/or histotoxic). Treatment of sinus tachycardia before or during anesthesia requires ruling out possible causes of tachycardia and treating as needed. Light anesthetic planes have been associated with sudden onsets of tachycardia and are due to increases in catecholamines as the patient mounts a physiologic response to noxious stimuli. Improvement of anesthetic depth or administration of analgesics typically resolves tachycardia due to this etiology. Rarely, β‐adrenergic blockers may be required to treat a pathologic sinus tachycardia; however, identification and treatment of underlying causes should be the focus of treatment. Atrial premature complexes are ectopic foci of depolarization in the atria, which lead to premature atrial contractions [247]. Atrial contraction has been described as providing an atrial kick, increasing end‐diastolic volume by 10–30% [252]. Loss of coordination and timing of the atrial contraction prevents this increase in end‐diastolic volume and leads to a decrease in CO if sufficiently frequent. APCs are most frequently caused by cardiac disease, most commonly atrial enlargement secondary to AV valvular disease, valvular dysplasia, and PDA, but can also be seen with metabolic, neoplastic, and/or inflammatory diseases that affect the atria [253]. Any cause of atrial volume overload may lead to atrial enlargement and development of APCs. APCs may also precede worsening atrial arrhythmias such as atrial tachycardia, atrial flutter, or SVT. The presence of APCs typically does not warrant specific treatment, provided overall perfusion is adequate, but when identified should alert the anesthetist to the possibility of occult cardiac disease and may suggest the need for further cardiac evaluation (thoracic radiographs, BP measurements, and/or echocardiogram) or the possibility that APCs could degrade into atrial tachycardia or atrial fibrillation. Atrial flutter is an atrial tachyarrhythmia (>300 beats min−1) in which P waves are replaced by a “sawtooth pattern” of atrial depolarization, referred to as flutter or “f” waves [254]. Flutter wave conduction to the ventricles is variable, so that there may be a 4:1 ratio of atrial “f” waves to ventricular complexes or a 1:1 ratio that is difficult to differentiate from atrial tachycardia. Causes of atrial flutter are the same as those for other atrial tachyarrhythmias, particularly those causing atrial enlargement. Reentry rhythms can underlie atrial flutter, as can feline restrictive or hypertrophic cardiomyopathy, and ruptured chordae tendineae. Treatment of atrial flutter is not well described in veterinary medicine but is aimed at slowing ventricular rate. Options include diltiazem or digoxin administration, direct current cardioversion, or precordial thump in an emergency. Atrial fibrillation is a common rhythm in dogs and tends to be a sustained rhythm, although paroxysms have been described [255]. It is the classic “irregularly irregular” rhythm noted on auscultation, pulse palpation, or ECG analysis. There is complete loss of P waves, replaced by a chaotic isoelectric line of “f” waves and irregular R‐to‐R intervals. It is characterized mechanically by complete lack of coordinated atrial activity. Loss of all atrial coordination prevents the atrial kick in most if not all cardiac cycles and leads to significantly reduced CO when combined with the high ventricular rates. Atrial fibrillation is often the rhythm associated with DCM of large breed dogs, severe atrial enlargement of any cause, and cases with severe mitral regurgitation; “lone” atrial fibrillation is seen in giant breed dogs that have atrial fibrillation but no structural cardiac disease. Treatment of atrial fibrillation focuses on medical therapy for rate control and includes digoxin, β‐adrenergic blockers, and calcium channel blockers. Slowing of ventricular rate is important to prevent the development of heart failure and to extend diastolic filling time and improve CO. However, long‐term conversion from atrial fibrillation to a sinus rhythm is often not possible with severe underlying cardiac disease. In cases with minimal underlying structural cardiac disease (typically those with lone atrial fibrillation and atrial enlargement only), cardioversion to a sinus rhythm may be considered. The decision to attempt cardioversion (medically or with electric cardioversion) is controversial, and there are no clear criteria for attempting cardioversion. Atrioventricular junctional tachycardia is due to the presence of an ectopic focus of depolarization in the AV node. Intrinsic automaticity rate of the AV node is 40–60 beats min−1. Therefore, an AV nodal tachycardia has only to be faster than this rate to be termed a tachycardia. The underlying mechanism is most commonly a reentry circuit. As the depolarization occurs in the AV node, the ventricular portion of the complex tends to be narrow. A noted variation in this rhythm is the presence of inverted P waves that can be seen before, during, or after the Q‐R‐S complex. It may not be possible to distinguish this rhythm from an atrial tachycardia at very high HRs, so the term SVT can be used to identify either rhythm. Treatment is aimed at breaking the reentry circuit with a calcium channel blocker (e.g., diltiazem) to reduce calcium entry into the myocyte and therefore reduce HR. Ventricular premature complexes (VPCs or PVCs) are due to ectopic depolarization foci located in the ventricular myocardium. They occur before the next expected Q‐R‐S complex based on the underlying R‐to‐R interval. Depolarization spreads cell to cell, resulting in a wide Q‐R‐S complex. Unifocal VPCs are individual wide Q‐R‐S complexes with the same morphology, indicating they come from the same focus of depolarization [256]. Multifocal VPCs have differing morphology and can be positive with different morphology, negative with different morphology, or both. Couplets (two consecutive wide complexes) and triplets are terms used to describe multiple VPCs occurring consecutively. A rhythm of alternating VPCs and sinus‐origin beats is referred to as ventricular bigeminy. There is often a compensatory pause after the VPC, due to the refractory period of the VPC. When the VPC does not affect the R‐to‐R interval of the underlying sinus rhythm, it is referred to as an “interpolated VPC.” R‐on‐T phenomenon occurs when the VPC occurs on the T wave of the previous sinus beat and can predispose to development of ventricular fibrillation (Vfib) [257]. The first line VPC treatment is a class 1b antiarrhythmic such as IV lidocaine or, less commonly, amiodarone. Recommended IV lidocaine doses range from 1 to 2 mg kg−1 in dogs or 0.25–1.0 mg kg−1 (up to 4 mg) for cats. Oral sotalol has also been recommended in cats but is not an option during anesthesia. Other therapies for ventricular arrhythmias have largely been unproven in the cat [252]. Mexiletine is an alternative for long‐term management, as it is only available as an oral preparation. Class 1b antiarrhythmic drugs are believed to shorten the refractory period and terminate reentry rhythms by this mechanism. Triggers for treatment include multifocal VPCs (as more of the heart is presumed to be diseased/affected, and degradation to a worsening rhythm is likely), runs of couplets/triplets/ventricular tachycardia, R‐on‐T phenomenon, or any ventricular rhythm that has hemodynamic consequences. In cases of refractory life‐threatening ventricular arrhythmias or shock‐resistant ventricular fibrillation, amiodarone is recommended. Causes of VPCs include hypoxia, cardiac disease (myocarditis, arrhythmogenic right ventricular cardiomyopathy [ARVC], neoplasia, trauma, and structural cardiac disease), splenic/hepatic neoplasia, gastric dilatation volvulus syndrome, acidosis, pain, and catecholamine or sympathomimetic therapy. Treatment of VPCs and ventricular rhythms must include evaluation, monitoring, and treatment of these mechanisms in addition to treatment for the arrhythmia itself. Ventricular tachycardia is defined as a ventricular rhythm greater than 160–180 beats min−1 in dogs, whether paroxysmal or sustained [256]. Idioventricular rhythm is the ventricular escape rhythm seen with loss of supraventricular input such as in complete (third‐degree) AV block, typically a pulse rate of 40–60 beats min−1 in dogs and 60–80 beats min−1 in cats. The most appropriate term for a complete ventricular rhythm with rates between 60 and 160 beats min−1 (in dogs) is an accelerated idioventricular rhythm; a ventricular rhythm that is not quite tachycardic. The major hemodynamic difference between accelerated idioventricular rhythm and ventricular tachycardia is the decrease in diastolic filling time as HR increases past 160–180 beats min−1, as well as the decrease in CO that results. Causes of ventricular tachycardia are the same as for VPCs, and the same considerations apply for treatment. Ventricular fibrillation is a chaotic organization of coarsely wandering electrical potentials of variable duration and amplitude with no P–Q‐R‐S–T organization. It is a nonperfusing rhythm, creating no mechanical activity in the heart, and CO is near zero. Ventricular fibrillation is a terminal rhythm and can result from severe ventricular tachycardia, severe systemic or cardiac disease, or cardiac surgery. The only treatment with a reasonable chance of converting Vfib to a perfusing rhythm is electrical defibrillation. However, the ability to convert to a sinus rhythm is often temporary, and fibrillation frequently recurs in minutes to hours. Sinoatrial block is failure of a normally generated SA nodal‐action potential to appropriately conduct to the atria and lead to atrial depolarization [253]. Sinoatrial block differs from sinus arrest in that SA block is a failure of conduction, while sinus arrest is failure of the SA node to depolarize (failure of impulse generation). It can be difficult to distinguish between them with routine Lead II ECG analysis in anesthetized patients. First‐degree SA block is a prolonged period from SA nodal firing and atrial depolarization. This is undetectable on ECG, as SA nodal firing is not recorded. Second‐degree SA block is identified by a pause after a sinus beat or beats, wherein the duration of the pause is an exact multiple of that of the underlying normal sinus rhythm P‐to‐P interval. SA block appears otherwise identical to sinus arrest. Sinus block can be the result of atrial disease (enlargement, fibrosis, cardiomyopathy, and neoplasia), drug toxicity (β‐adrenergic and calcium channel blockers), or potentially SSS. SA nodal blocks typically do not require treatment. However, if severe bradycardia develops, treatment should be considered, as they may be responsive to atropine/glycopyrrolate. If the rhythm fails to respond to anticholinergics and the patient is clinical for the arrhythmia, transcutaneous or transvenous cardiac pacing may be required. Atrial standstill is failure of normally generated SA nodal potentials to depolarize the atria. The ECG appears as a flat line with no P waves. Atrial standstill can be due to diseased atrial myocardium that is unable to depolarize normally, or, more commonly, electrolyte disturbances such as hyperkalemia, where elevated serum potassium levels are sufficiently high to prevent atrial depolarization [251]. Common causes of hyperkalemia include urinary obstruction, renal failure, uroabdomen, and hypoadrenocorticism. At moderate to severe serum potassium levels, the SA node and ventricular myocardium maintain their ability to depolarize, albeit slowly, but no P waves are seen on the ECG; slowed and widened Q‐R‐S complexes can be seen. Elevations in serum potassium may not be correlated to the severity of arrhythmias; alterations in the ECG can be seen at severe hyperkalemia, and classic changes in the ECG waveform can be seen with low serum potassium levels. However, typically as potassium increases, the T waves become “tall and tented,” P waves flatten, and the P‐to‐R interval increases in duration, progressing to atrial standstill followed by widening of the Q‐R‐S complex until the ECG appears as a sine wave. Ventricular arrhythmias may also present at any time. Treatment for hyperkalemia is focused on identification and treatment of the underlying cause [258]. Immediate stabilization of the hyperkalemic patient involves decreasing serum potassium levels and treating underlying acid–base disturbances to move potassium intracellularly. These mechanisms are critically important in reducing serum potassium levels in a patient who requires general anesthesia to treat the underlying disease. Calcium gluconate (50–100 mg kg−1 as a slow 5 min IV bolus) or calcium chloride (10 mg kg−1 as a similar bolus) can be given to counteract the electrochemical effects of hyperkalemia on resting membrane hyperpolarization and rapidly treat the ECG side effects of hyperkalemia. Improvements in ECG can be seen within minutes of administration and can last between 30 and 60 min, allowing time for other treatments to reduce the hyperkalemia. Calcium given too rapidly can cause bradycardia and worsen the rhythm, and so should be given slowly while monitoring the patient with an ECG placed. Another strategy for decreasing serum potassium in the hyperkalemic patient is to cotransport potassium with glucose into cells under the influence of insulin. Recommendations for insulin/dextrose therapy are 0.25 units kg−1 of regular insulin IV given with 1–2 g of dextrose (diluted 3:1 with saline) for every full unit of insulin administered. Serial glucose monitoring is recommended, and patients may require a dextrose infusion (1.25–2.5%) to prevent hypoglycemia. In humans, onset time of insulin/dextrose therapy is 20 min, with a duration of 30–60 min [258]. Finally, sodium bicarbonate can be used to buffer an underlying acidosis and reverse the shift of transmembrane antiport of hydrogen ions and potassium, thus moving potassium back into cells. Once venous or arterial blood gas analysis is complete (pH, base excess, bicarbonate, and PCO2), total bicarbonate deficit can be estimated with the formula: total deficit = 0.3 × base excess × body weight (in kilograms). If bicarbonate therapy is appropriate, it is recommended to replace no more than 1/3–1/2 of the deficit. Administration of a larger dose of bicarbonate risks overcorrection and development of an alkalosis. One of the more significant buffering mechanisms for bicarbonate therapy is the generation of CO2 based on the carbonic anhydrase equation:
1
Cardiovascular Disease
Introduction
Cardiovascular Physiology
Tissue Perfusion and Oxygen Delivery
Blood Pressure and Cardiac Output
Mean Arterial Pressure and Autoregulation
Hypotension
Relationship Between Mean Arterial Pressure and Cardiac Output
Preanesthetic Patient Assessment
Functional Classification of Cardiac Disease
Sedation Versus General Anesthesia
Category
Physical status
Clinical examples
I
Normal, healthy patients
No detectable disease; patients presenting for ovariohysterectomy or castration
II
Patients with mild, systemic disease
Skin tumor, fracture without shock, uncomplicated hernia, cryptorchidectomy, localized infection, or compensated disease
III
Patients with severe, systemic disease fever, dehydration, anemia, cachexia, or moderate hypovolemia
IV
Patients with severe, systemic disease that is a constant threat to life
Uremia, toxemia, severe dehydration and hypovolemia, cardiac decompensation, emaciation, or high fever
V
Moribund patients that are not expected to survive 24 h
Extreme shock, terminal malignancy or infection, severe trauma
Anesthetic and Analgesic Agents
Premedicants
Opioids
Full Mu‐Opioid Receptor Agonists
Morphine
Hydromorphone/Oxymorphone
Methadone
Fentanyl
Remifentanil, Sufentanil, and Alfentanil
Mixed Opioid Agonist–Antagonist Receptor Drugs
Butorphanol
Nalbuphine
Partial Mu‐Opioid Receptor Agonists
Buprenorphine
Phenothiazines
Benzodiazepines
Alpha‐2‐Adrenergic Receptor Agonists
Alfaxalone
Anticholinergics
Induction Agents
Propofol
Alfaxalone
Dissociative Anesthetics
Etomidate
High‐Dose Opioids
Anesthetic Maintenance
Inhaled Anesthetics
Propofol (dogs)
Induction dose IV
CRI dose
Bustamante et al. [103]
0.65–5 mg kg−1
0.25 ± 4.7 mg kg−1 min−1
Park et al. [104]
0.2–0.4 mg kg−1 min−1
Kennedy and Smith [105]
4.0 ± 1.0 mg kg−1 (with ketamine 1:1)
0.3 ± 0.1 mg kg−1 min−1
5.3 ± 1.0 mg kg−1
0.6 ± 0.1 mg kg−1 min−1
Mohamadnia et al. [106]
8 mg kg−1
0.3 mg kg−1 min−1
Tsai et al. [107]
1.5–2.0 mg kg−1
Raisis et al. [108]
To effect
0.4 mg kg−1 min−1
Murrell et al. [109]
0.33 mg kg−1 min−1
Hughes and Nolan [110]
4 mg kg−1
0.2–0.4 mg kg−1 min−1
Smith et al. [111]
8.68 ± 0.57 mg kg−1
0.68 ± 0.13 mg kg−1 min−1
6.13 ± 0.67 mg kg−1
0.49 ± 0.16 mg kg−1 min−1
4.78 ± 0.39 mg kg−1
0.26 ± 0.05 mg kg−1 min−1
Alfaxalone (dogs)
Induction dose IV
CRI dose
Hunt et al. [112]
1.4 mg kg−1
99 μg kg−1 min−1
Bennell et al. [113]
1.5 mg kg−1
Dehuisser et al. [114]
2 mg kg−1
150 μg kg−1 min−1
Liao et al. [115]
1.5 mg kg−1
268 μg kg−1 min−1
2.1 mg kg−1
310 μg kg−1 min−1
0.62 mg kg−1
83 μg kg−1 min−1
0.98 mg kg−1
87 μg kg−1 min−1
Conde Ruiz et al. [116]
3 mg kg−1
200 μg kg−1 min−1
Herbert et al. [117]
1.5 ± 0.57 mg kg−1
0.11 (0.07–0.33) mg kg−1 min−1
1.2 (0–6.3) mg kg−1
0.08 (0.06–0.19) mg kg−1 min−1
Suarez et al. [118]
Propofol = 5.8 ± 0.30 mg kg−1
Propofol = 0.37 ± 0.09 mg kg−1 min−1
Alfaxalone = 1.9 ± 0.07 mg kg−1
Alfaxalone = 0.11 ± 0.01 mg kg−1 min−1
Alfaxalone (cats)
Induction dose IV
CRI dose
Beths et al. [119]
1.7 (0.7–3.0) mg kg−1
0.18 (0.06–0.25) mg kg−1 min−1
Schwarz et al. [120]
2.57 ± 0.41 mg kg−1
0.19–0.022 mg kg−1 min−1
Total Intravenous Anesthesia
Study
Loading dose (mg kg−1)
CRI dose (μg kg−1 min−1)
Inhalant
MAC (%)
MAC reduction
MAC reduction percentage
Remifentanil
Brosnan et al. [125]
None
0.0625–16
Isoflurane
1.94 ± 0.8
None
None
Ferreira et al. [46]
None
0.25
Isoflurane
1.66 ± 0.08
1.27 ± 0.13
−23% ± 7.9%
None
0.5
Isoflurane
1.66 ± 0.08
1.16 ± 0.17
−29.8% ± 8.3%
None
1.0
Isoflurane
1.66 ± 0.08
1.22 ± 0.15
−26% ± 9.4%
Ketamine
Pascoe et al. [126]
2.0
23
Isoflurane
1.51 ± 0.23
0.84 ± 0.33
−45% ± 17%
2.0
46
Isoflurane
1.51 ± 0.23
0.57 ± 0.35
−63% ± 18%
16.0
115
Isoflurane
1.51 ± 0.23
0.41 ± 0.35
−75% ± 17%
Study
Loading dose (mg kg−1)
CRI dose (μg kg−1 min−1)
Inhalant
MAC (%)
MAC reduction
MAC reduction percentage
Fentanyl
Ueyama [127]
0.005
0.15
Isoflurane
1.42 ± 0.08
0.93 ± 0.04
−35%
Hellyer [128]
0.01
0.3
Isoflurane
1.8 ± 0.21
0.85 ± 0.14
−53%
Remifentanil
Michelsen [129]
None
1.0
Enflurane
2.1 ± 0.2
NR
−63% ± 10.4%
Allweiler [130]
None
0.1
Isoflurane
1.28 ± 0.13
0.78 ± 0.17
−40%
None
0.25
Isoflurane
1.28 ± 0.13
0.65 ± 0.16
−50%
Monteiro et al. [47]
None
0.15
Isoflurane
1.24 ± 0.18
NR
−43% ± 10%
None
0.3
Isoflurane
1.24 ± 0.18
NR
−59% ± 10%
None
0.6
Isoflurane
1.24 ± 0.18
NR
−66% ± 9%
None
0.9
Isoflurane
1.24 ± 0.18
NR
−71% ± 9%
Ketamine
Muir et al. [29]
None
10
Isoflurane
1.38 ± 0.08
1.3 ± 0.07
−25%
Queiroz‐Castro [131]
1.0
25
Isoflurane
1.06 ± 0.02
0.73 ± 0.04
−28.7% ± 3.7%
Doherty [132]
1.5
50
Isoflurane
1.11 ± 0.05
0.56 ± 0.04
−49.6%
Love [133]
0.5
6.25
Sevoflurane
2.62 ± 0.21
2.61 ± 0.22
−0.4% ± 4%
1.0
12.5
Sevoflurane
2.62 ± 0.21
2.06 ± 0.22
−22% ± 4%
Anesthetic Adjuncts
Local and Regional Anesthesia and Analgesia
Systemic Analgesic Infusions
Nonsteroidal Anti‐Inflammatory Drugs
Sympathomimetic Drugs: Inotropes and Vasopressors
Dopamine
Dobutamine
Sympathetic nervous system receptor activation
Alpha‐1
Alpha‐2
Beta‐1
Beta‐2
Dopamine (D1)
Vasopressin (V1)
Dose
Effect
Vasoconstriction bradycardia
Vasoconstriction
Inotropy chronotropy
Vasodilation bronchodilation
Vasodilation
Vasoconstriction
Drug
Dopamine
+++ (High dose)
+
+++ (Low dose)
+ (Low dose)
+++
−
low: 5–10 μg kg−1 min−1 high: 10–20 μg kg−1 min−1
Dobutamine
−
−
+++
++
−
−
1–10 μg kg−1 min−1
Epinephrine
+++
+++
++
++
−
−
Bolus: 0.01–0.1 mg kg−1 CRI: 0.01–1.0 mg kg−1 min−1
Isoproterenol
−
−
+++
+++
−
−
0.01–0.1 mg kg−1 min−1
Norepinephrine
+++
−
++
+++
−
−
0.05–2.0 μg kg−1 min−1
Phenylephrine
+++
−
−
−
−
−
Bolus: 1–5 μg kg−1 CRI: 0.5–3.0 μg kg−1 min−1
Vasopressin
−
−
−
−
−
+++
Bolus: 0.1–0.6 mg kg−1 CRI: 1–4 mU kg−1 min−1
Epinephrine
Ephedrine
Vasopressin
Norepinephrine
Phenylephrine
Patient Monitoring and Support
Fluid Therapy
Patient Preoxygenation
Blood Pressure (BP)
PaCO2 (mmHg)
30
40
60
90
FiO2 (%)
PAO2
PaO2
PAO2
PaO2
PAO2
PaO2
PAO2
PaO2
21
112.2
101.0
99.7
89.8
74.7
67.3
49.7
44.8
30
176.4
159.8
163.9
147.5
138.9
125.0
113.9
102.5
40
247.7
222.9
235.2
211.7
210.2
189.2
185.2
166.7
100
675.5
608.0
663.0
596.7
638.0
574.2
613.0
551.7
Invasive Blood Pressure
Noninvasive Blood Pressure
Electrocardiography
Pulse Oximetry
Core Body Temperature
Motion artifact
Tissue thickness
Tissue hypoperfusion/hypotension
Vasoconstriction
Hypothermia
Tissue pigment
Methemoglobinemia (SpO2 values approach 85% when MetHgb is approximately 30%)
Carboxyhemoglobinemia (SpO2 values falsely elevated)
Intravenous dye injections (indocyanine green, methylene blue)
Ambient light at 660 and 920 nm wavelength(s)
Severe anemia (Hb <5 g dl−1)
Capnometry and Ventilation
Arterial Blood Gases
Central Venous Pressure (CVP)
Arterial Pulse Pressure Variation (PPV)
Anesthetic and Pharmacologic Recommendations for Specific Cardiac Diseases
General Preanesthetic Evaluation
Valvular Heart Disease
Introduction
Degenerative Mitral Valve Disease (dMVD)
Incidence and Pathophysiology
Physical Examination Findings
Anesthetic Management
Mitral Valve Stenosis (MVS)
Incidence and Pathophysiology
Anesthetic Management
Tricuspid Valve Stenosis (TVS)
Incidence and Pathophysiology
Anesthetic Management
Aortic Stenosis
Incidence and Pathophysiology
Anesthetic Management
Pulmonic Stenosis (PS)
Incidence and Pathophysiology
Anesthetic Management
Congenital Cardiac Disease
Patent Ductus Arteriosus
Incidence and Pathophysiology
Anesthetic Management
Tetralogy of Fallot
Incidence and Pathophysiology
Anesthetic Management
Ventricular Septal Defect
Incidence and Pathophysiology
Anesthetic Management
Abnormalities of Cardiac Conduction and Cardiac Rhythm
Electrophysiology of the Conduction System
Mechanisms Eliciting Cardiac Arrhythmias
Normal Sinus Impulse Formation
Normal sinus impulse formation
• Normal sinus rhythm
• Sinus arrhythmia
• Wandering sinus pacemaker
• Disturbances of sinus impulse formation
• Sinus arrest
• Sinus bradycardia
• Sinus tachycardia
Disturbances of supraventricular impulse formation
• Atrial premature complexes
• Atrial tachycardia
• Atrial flutter
• Atrial fibrillation
• Atrioventricular junctional rhythm
Disturbances of ventricular impulse formation
• Ventricular premature complexes
• Ventricular tachycardia
• Ventricular asystole
• Ventricular fibrillation
Disturbances of impulse conduction
• Sinoatrial block
• Persistent atrial standstill (“silent” atrium)
• Atrial standstill (hyperkalemia)
• Ventricular pre‐excitation
• Atrioventricular (AV) block
Evaluation of the ECG Rhythm
Heart Rate
P–Q‐R‐S Relationship
R‐to‐R Interval
Q‐R‐S Morphology
Periodicity
Specific Arrhythmias
Sinus Arrhythmia
Wandering Sinus Pacemaker
Sinus Arrest
Sinus Bradycardia
Sinus Tachycardia
Atrial Premature Complexes (APCs)
Atrial Flutter
Atrial Fibrillation
Atrioventricular Junctional Tachycardia
Ventricular Premature Complexes
Ventricular Tachycardia
Ventricular Fibrillation
Sinoatrial Block (SA Block)
Persistent Atrial Standstill
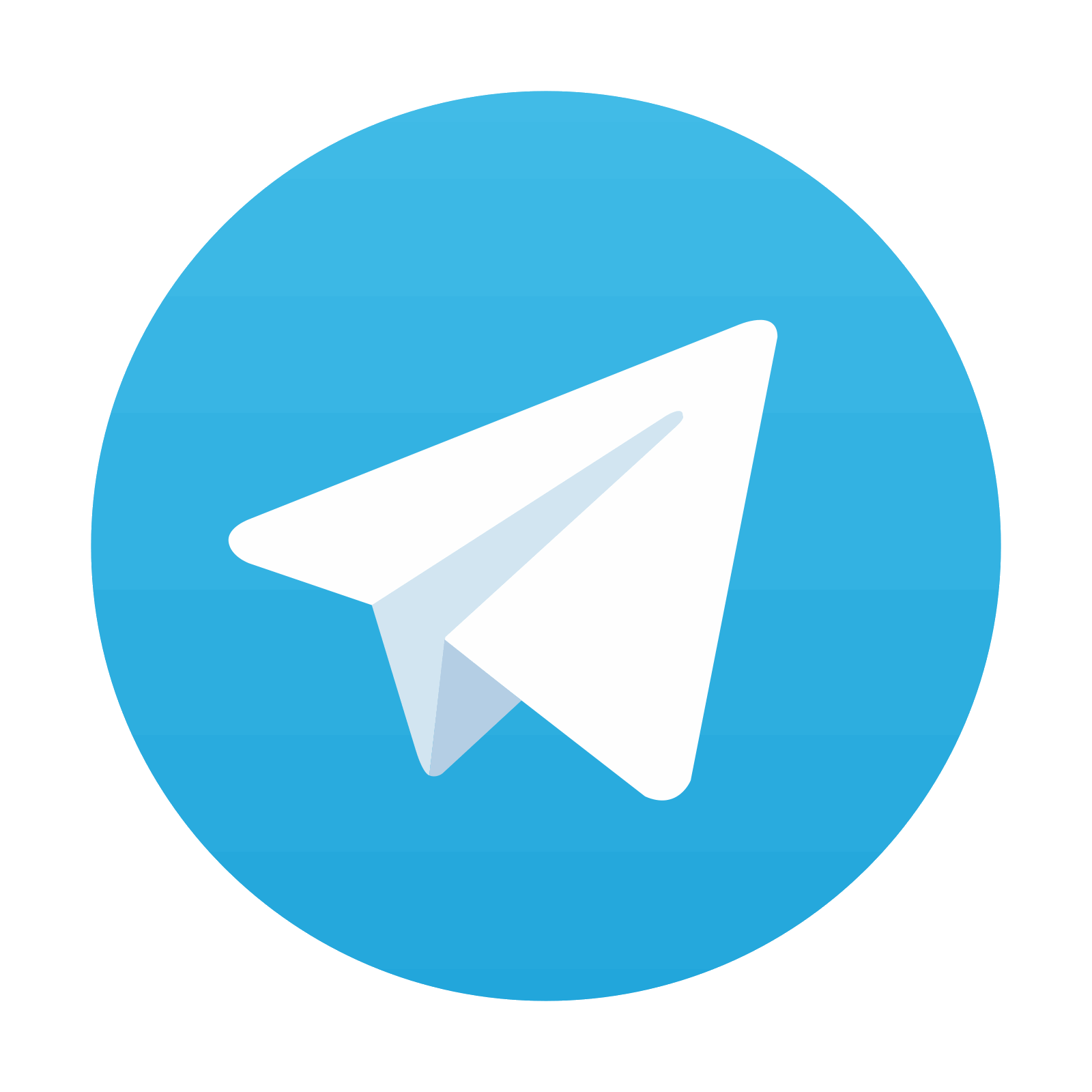
Stay updated, free articles. Join our Telegram channel
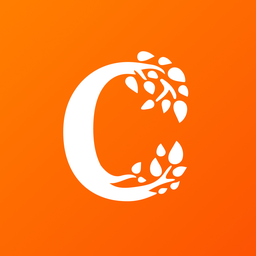
Full access? Get Clinical Tree
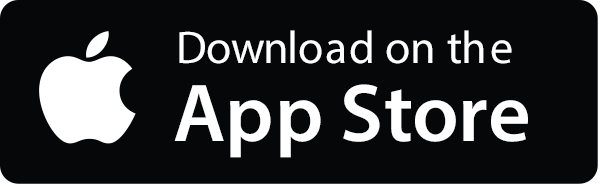
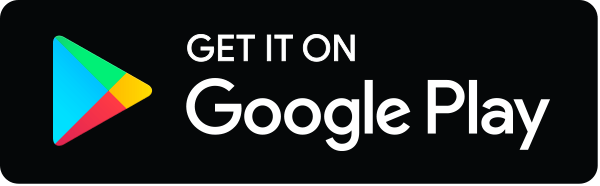