Alessio Vigani Department of Small Animals, Faculty of Veterinary Medicine, University of Zürich, Zürich, Switzerland Cardiac output is the volume of blood ejected from each of the ventricles per minute, usually reported in L/min, and is the product of the heart rate and stroke volume. Cardiac index is the cardiac output referenced to the body surface area or body weight of the patient and is expressed in L/min/m2 or L/min/kg, respectively (Table 13.1). Cardiac output is determined by five primary variables: heart rate, rhythm, preload, contractility, and afterload [1]. Cardiac output summarizes in one single value the contribution of the cardiovascular system to global oxygen delivery from the heart to the body. It, therefore, appears reasonable to monitor cardiac output in the assessment of cardiovascular insufficiency in any critical patient undergoing anesthesia. Unfortunately, no definitive studies have shown that the determination of cardiac output or its changes in response to therapy is superior to standard hemodynamic monitoring in terms of outcome [2]. In fact, there is lack of evidence of improved outcomes in acutely ill patients with use of any specific monitoring device, not only cardiac output monitors [3]. Moreover, cardiac output should not be evaluated as a sole variable in a clinical setting. For instance, if metabolic rate is increased or blood flow distribution is abnormal in septic patients, they often have a normal or even increased cardiac output and may still be in circulatory failure [4]. This raises the question: why do we measure cardiac output? There is clear evidence that persistence of a low cardiac output state has significant detrimental effects on organ perfusion and oxygenation [5]. In critically ill human patients, a low cardiac output with sustained unresponsiveness to therapy has been associated with a high mortality rate [6]. Thus, at least in some specific patients, the measurement of cardiac output may represent a supplemental aid to assess the adequacy of therapy and to guide targeted therapeutic interventions (e.g., goal‐directed fluid therapy) [2]. More specifically, in a hypotensive patient, whenever the clinical signs are difficult to interpret or the cause of hypotension is not obvious and likely multifactorial (including anesthetic drug effects), the use of cardiac output monitoring should be considered. In this regard, estimation of cardiac output is a useful tool for patient management during anesthesia. Measuring cardiac output is advantageous in monitoring anesthetic‐related hemodynamic changes as well as assessing effectiveness of resuscitation in trauma and critically ill patients [7]. Monitoring the extent of the relative changes in cardiac output, for example, following fluid resuscitation in hypovolemic patients, allows the anesthetist to assess the effectiveness of the intervention and to separate fluid responders from non‐responders [8–10]. In different scenarios, following patient trends in cardiac output rather than blood pressure, such as during dobutamine or a nitroprusside infusion, gives more meaningful information as to the hemodynamic response to therapy [11]. Importantly, the determination of absolute values of cardiac output is often less important than following trends. The assessment of relative changes in cardiac output following challenge of the cardiovascular system with fluid loading or medications is referred to as “functional cardiac output monitoring” [12]. Once it is determined that assessing cardiac output may be indicated for a given circumstance, then it is also important that its measurement is accurate enough to identify clinically relevant changes in response to therapy. An acute increase in cardiac output of 20–25% is usually accepted as a clinically relevant positive response because such a change corresponds to the limit of accuracy of current measuring techniques [13]. Table 13.1 Examples of typical cardiac output indices reported in veterinary species. The ideal cardiac output monitoring technique would provide accurate, interpretable, and reproducible measurements, be user‐friendly, and be readily available. The ideal device should also be safe for the patient, operator independent, provide a rapid response time, and be cost effective. Ultimately, the results obtained should be clinically relevant to guide therapy. To be useful in the management of hemodynamically unstable patients, the technique should allow cardiac output to be measured rapidly and repetitively [14]. At present, there is no available device that meets all these criteria. Veterinary anesthesiologists now have an increasing number of techniques and measuring devices available to assess cardiac output. Each technique has its own strengths and limitations [15,16]. As for the selection of monitoring systems for any other physiologic parameter, the following general principles can serve as guidelines to determine the most suitable cardiac output monitoring technique for use in a particular setting. No hemodynamic monitoring technique can improve outcome by itself and estimating cardiac output is not an exception. Three fundamental conditions must be met for hemodynamic monitoring to positively affect outcome: (1) the results obtained must be of clinical relevance to the patient; (2) the obtained data must be sufficiently accurate and precise to trigger a therapeutic intervention; and (3) the therapeutic intervention must improve outcome. If the information obtained is inaccurate, or interpreted or applied incorrectly, the resultant intervention is unlikely to improve the patient’s condition and may potentially be detrimental [3,13]. The choice of monitoring system depends on the patient (species and size) and often on the devices and expertise available at a specific hospital or institution. The expertise and familiarity of the operator represents a critical factor in obtaining reliable results with many cardiac output monitoring devices. A typical example of high operator dependency is represented by echocardiography compared to other automatic devices that consistently produce results with low interoperator variability [13]. As with any other monitored parameter, it is critical to integrate cardiac output data with other physiologic variables from multiple sources. The patient’s blood pressure is important complementary information. A low cardiac output in a hypotensive patient will likely indicate hypovolemia or decreased cardiac function, whereas a high cardiac output in a hypotensive patient would suggest decreased systemic vascular resistance [12]. In the clinical setting, cardiac output is estimated, not measured [16]. The only technique that allows its user to directly measure cardiac output is electromagnetic flowmetry, which requires the surgical implantation of a flow probe circumferentially on the main pulmonary artery. This technique is considered the gold standard in the research setting, but it is, for obvious reasons, impractical for clinical applications [17]. All values from bedside techniques are estimates of cardiac output based on assumptions and algorithms. Because of this estimation, comparison of results from different techniques often produces relatively poor agreement and significant bias [16]. In humans, the invasive pulmonary artery catheter (PAC) thermodilution technique is generally considered as the “reference” standard for clinical purposes but has its own limitations [8]. A measurement obtained by a less invasive technique may be preferable if it can be obtained more rapidly and easily, even if it is slightly less accurate. In veterinary medicine, an example of this is the utilization of the lithium dilution cardiac output technique as a suitable reference method in horses for comparison of other cardiac output monitoring devices [15,18–20]. Non‐invasiveness of the technique should not be the priority. Although obviously preferable, being non‐invasive is not always possible and may even be counterproductive. For instance, the real advantage of echocardiography over other cardiac output techniques is not its non‐invasiveness but the peculiarity of providing a direct evaluation of cardiac function [21,22]. A completely non‐invasive technique such as bioimpedance, despite reliability in healthy human individuals, has proved inconsistent when applied to critically ill patients or when used in other species [13,23]. The first technique for calculation of cardiac output was described by Adolph Fick in 1870. Fick described the calculation of cardiac output from the measurement of the oxygen content of arterial and mixed venous blood as well as oxygen consumption [24]. The Fick technique requires catheterization of the pulmonary artery for mixed venous blood sampling. This was first performed experimentally in live dogs in 1886 by Grehant and Quinquaud [25], and then by Zuntz and Hagemann in a horse in 1898 [26]. Its clinical use in humans did not occur for almost another 50 years. Measurement of oxygen consumption has always represented a significant challenge, and this remains a major limitation of the Fick technique [27]. For this reason, the use of estimates of oxygen consumption was introduced, and the method was referred to as the “modified Fick technique.” The Fick technique became the standard for the measurement of cardiac output until the development of dye dilution techniques. The dye dilution technique of cardiac output determination is based on the independent work of Stewart and Hamilton in the late nineteenth and early twentieth centuries [28]. The equation they derived allowed for the estimation of cardiac output by knowing the amount of an injected indicator and calculating the area under its dilution curve measured downstream. Dye dilution techniques were repeatedly shown to be as reliable as the Fick technique and of better suitability in the clinical setting [29–31]. Thus, dye dilution rapidly became the “accepted method of reference” for cardiac output measurement [32]. Thermodilution, first described by Fegler in 1954, uses heat as an indicator and relies on the same principles of dye dilution [33]. The landmark article on right heart catheterization with a balloon‐tipped catheter by Swan et al. in 1970 [34] finally set the stage for the widespread clinical use of cardiac output monitoring [35]. Since then, the accuracy of thermodilution‐based cardiac output measurements has been assessed by hundreds of studies. Interestingly, all of these studies share a common major limitation – the lack of a gold standard for comparison. Over time, PAC‐based thermodilution showed good agreement with direct Fick calculations and dye dilution techniques in a wide range of clinical conditions, and this, combined with the relative ease of insertion of PACs in humans, has made this technique the clinical “gold standard” for comparison for all new cardiac output monitors [32,36,37]. Despite the widespread use of PAC thermodilution, many studies have questioned the effectiveness and safety of this technology [38–43]. This has driven the recent interest in the development of alternative technologies that can measure cardiac output less invasively [44–46]. The development and validation of new technologies to measure cardiac output has potential applications in both veterinary clinical and research settings. Many of the techniques described in this chapter are currently in use or will eventually be suitable for use in veterinary medicine. Cardiac output can be elegantly estimated by using the Fick principle. The technique is based on the law of conservation of mass [27]. The principle states that over a given time period, the quantity of O2 or CO2 entering or leaving the lungs is equal to the quantity of the gas taken up or expelled by the blood flowing in the pulmonary circulation. In mathematical terms, the Fick principle‐derived cardiac output equals the patient’s oxygen uptake (VO2) divided by the difference between arterial and mixed venous oxygen content (CaO2 – C This relationship is true only in the absence of any cardiopulmonary shunting [47]. Determining cardiac output using the “direct Fick method” requires catheterization of both a peripheral artery and the main pulmonary artery for arterial and mixed venous blood sampling, respectively [27]. The measurement of VO2 also requires accurate collection and analysis of exhaled gases or determination via closed‐circuit techniques. The measurement of VO2 is the most problematic step of the direct Fick method that has ultimately limited its use for clinical purposes [47]. Indirect techniques based on the Fick principle (also known as the “partial rebreathing technique”) have been developed to eliminate the need for pulmonary artery catheterization and invasive blood gas sampling. The technique utilizes elimination of carbon dioxide (CO2) rather than uptake of oxygen [48] and involves intermittent periods of partial rebreathing to allow minimally invasive estimation of arterial and mixed venous CO2 content. The monitor consists of a carbon dioxide sensor, a disposable airflow sensor connected to a closed loop system, and a pulse oximeter. Arterial and mixed venous CO2 content is estimated from measurements of end‐tidal CO2 partial pressure (PETCO2) during normal breathing and rebreathing maneuvers. VCO2 is calculated from minute ventilation and its carbon dioxide content. The arterial carbon dioxide content (CaCO2) is estimated from end‐tidal carbon dioxide during periods of ventilation. Intermittent phases of partial rebreathing allow estimation of mixed venous CO2 partial pressure (P Each cardiac output determination requires about 4 min. Every 3 min, a rebreathing valve that prevents normal volumes of CO2 from being eliminated is activated, and the patient’s inhaled and exhaled gases are diverted through the loop for 50 s [48]. As a result, the elimination of CO2 drops and the concentration of CO2 in the pulmonary artery increases. It is assumed that cardiac output remains unchanged under normal (N) and rebreathing conditions (R). To compensate for the presence of shunted blood, cardiopulmonary shunting is estimated by use of pulse oximetry and FiO2 [49]. The general equation that forms the basis of this technique is: where VCO2, C Because the diffusion rate of carbon dioxide is 20 times higher than that of oxygen, it is assumed that no difference in venous CO2 (C The partial CO2 rebreathing (indirect Fick) technique for cardiac output estimation has shown sufficient reliability for clinical application in adult and pediatric human patients [50–52]; however, its accuracy in critical patients has been questioned [53]. One such device (the NICO® monitor, Novametrix Medical Systems) has been tested in veterinary species including dogs and horses, but more modern technologies are available [53–55]. The need for endotracheal intubation and mechanical ventilation limits the use of this device to the intraoperative period. Furthermore, the need for constant CO2 elimination precludes use during spontaneous breathing in small animals due to tidal volume variability [55]. In validation studies in humans and in dogs, a tidal volume of 12 mL/kg produced cardiac output determinations that correlated well with measurements obtained by both thermodilution and lithium dilution techniques [55,56]. The traditional intermittent thermodilution technique uses a bolus of ice‐cold sterile saline as an indicator that is injected into the right atrium via a PAC (Swan‐Ganz catheter). The change over time in temperature of the blood in the main pulmonary artery is then used to calculate cardiac output [36]. A saline bolus of known volume and temperature is injected into the right atrium via the proximal port of a Swan‐Ganz catheter [57,58]. The saline solution can be cooled in ice or injected at room temperature and can be administered in a volume of either 5 or 10 mL. As a general rule, higher volumes and lower temperatures produce the most accurate results. The saline mixes with the blood as it passes through the right ventricle and the pulmonary artery, thus decreasing the temperature of the blood. The blood temperature changes are detected by a thermistor at the distal end of the catheter, which lies within the main pulmonary artery, and a computer acquires the thermodilution curve over time (Fig. 13.1) [33]. The cardiac output computer then calculates flow (cardiac output from the right ventricle) using the acquired blood temperature information, and the starting temperature and volume of the injected saline bolus. The injection is normally repeated at least twice and the measurements averaged. Because cardiac output changes during the respiratory cycle, it is important to inject the saline during a consistent phase of respiration; conventionally, this is done at the end of expiration [37]. Figure 13.1 Graphical representation of the dilution curve for pulmonary artery catheter (PAC) thermodilution‐derived cardiac output. As with all of the indicator‐dilution methods, the measurement of thermodilution cardiac output is based on the Stewart–Hamilton equation: The denominator in the formula is a mathematical integration defined by the integral sign ∫, while ΔTemp is the gradient in temperature and represents the integral in the function. dt indicates that time (t) is the variable of integration. The domain of the integration is from t = 0 to t = ∞. The function is then defined as the area under the curve bounded by the graph of ΔTemp, the time axis, and the vertical lines t = 0 and x = ∞. The temperature curve does not actually return to baseline because of recirculation; the computer accounts for this in the calculation of the area under the curve by extrapolating the projected return to baseline of the temperature. Cardiac output is inversely proportional to ∫ΔTemp dt; therefore, when cardiac output is high, the indicator crosses the temperature sensor quickly, producing a small area under the ΔTemp curve. Conversely, when cardiac output is low, the bolus of cold saline diffuses in the right ventricle and pulmonary artery over a relatively long time, causing the area under the temperature versus time curve to be larger (Fig. 13.2). A modification of the original Swan‐Ganz catheter includes a proximal thermal coil to warm blood in the cranial vena cava. The changes in blood temperature are then detected at the PAC’s distal end using a thermistor. This method allows the continuous estimation of cardiac output, with the displayed values representing the average value of repeated determinations over the previous 10 min [58,59]. The use of averaged values of cardiac output corrects the inaccuracy of thermodilution associated with possible arrhythmias. The main disadvantage of this method is not being able to produce real‐time values, thus limiting its usefulness in rapidly recognizing cardiac output changes in hemodynamically unstable patients. Figure 13.2 Graphical representation of cardiac output thermodilution curves. A. Normal cardiac output. B. High cardiac output. C. Low cardiac output. Note the inverse relationship between the area under the curve (AUC) and cardiac output. Pulmonary artery catheterization allows simultaneous measurements of other hemodynamic parameters in addition to cardiac output, including pulmonary artery pressures, right‐sided and left‐sided filling pressures, and mixed venous oxygen saturation (S There are some important sources of error with thermodilution measurements that must be considered. If the volume injected is lower than that entered into the computer, the detected area under the curve (AUC) will be artificially small and the calculated cardiac output will be falsely high. Further, if the temperature of the injected saline is colder than expected (e.g., using iced rather than room temperature saline), the temperature change will be artificially large and the estimated cardiac output will be erroneously low [38]. Significant complications have been associated with the use of PACs, and several reports have described the intrinsic risk of morbidity and mortality [39–43]. The “PAC Man” study in human patients recorded complications in 10% of insertions. The most common complications were arrhythmias, heart block, rupture of the right heart or pulmonary artery, thromboembolism, pulmonary infarction, valvular damage, and endocarditis. Similar endocardial and valvular lesions were also described in horses following PAC placement [60]. Therefore, the use of the PAC should be restricted only to selected patients where the benefit of its use may substantially outweigh the risks. Current recommendations in humans limit the use of PACs to patients with severe right ventricular failure and those requiring intensive monitoring of pulmonary vascular resistance during vasodilator therapy. Moreover, the use of PACs and PAC thermodilution necessitate appropriate training as placement errors and misinterpretation of data are common. In veterinary patients, there is limited experience with clinical use of PACs and PAC thermodilution, and the technique has been largely limited to research settings [61–64]. This is mainly due to the invasiveness and the difficulty of placing and maintaining the catheter in the appropriate location. In addition, the PACs available on the market are specifically sized and designed for use in humans and, as such, are often inadequate for direct use in other species due to morphological differences. In small dogs (less than 5 kg) and cats, for example, a standard PAC designed for adult human use advanced into the main pulmonary artery would likely have the proximal port located in the jugular vein. The injection of the indicator in this location, rather than in the right atrium, will create loss and delayed mixing with significant error in the estimation of cardiac output. The opposite scenario occurs in horses where adult human PACs are too short. The proximal opening would then be located in the right ventricle, instead of its proper place in the right atrium, significantly altering the estimation of cardiac output. However, pediatric human PACS and custom‐designed PACs of appropriate size for various veterinary species have been used for research purposes. The basic principles of PAC thermodilution also apply to newer cardiac output monitoring systems that do not require the use of an actual pulmonary arterial catheter. Examples of these systems are the PiCCO® (Pulsion Medical Systems) and COstatus® (Transonic; no longer commercially available) that allow cardiac output to be estimated using central venous and arterial catheters only [65,66]. The Stewart–Hamilton equation is still the basis for estimating cardiac output using these systems. The PiCCO® system is based on transpulmonary thermodilution for cardiac output determination and requires dedicated femoral artery access for measurements. PiCCO® estimates cardiac output using central venous injections of ice‐cold intravenous fluid as an indicator and measuring the changes over time in temperature by an arterial thermistor‐tipped catheter placed in the femoral artery [65]. The COstatus® monitor is no longer manufactured but is a system that is still utilized in the scientific literature [66]. This monitor estimates cardiac output by using ultrasound technology to measure changes in the ultrasound velocity of blood following an injection of a small saline bolus (0.5–1 mL/kg) warmed to body temperature (37 °C). To obtain measurements, a roller pump circulates blood through a disposable extracorporeal arteriovenous (AV) loop interposed between the peripheral arterial catheter and the distal lumen of the central venous catheter. Two reusable sensors that measure changes in blood ultrasound velocity and blood flow in the AV loop are clamped onto the arterial and venous limbs of the loop. The venous sensor detects the injection of saline and records the time and volume of injection. The arterial sensor then measures the changes in concentration of saline in the blood as a dilution and records the indicator travel time through the cardiopulmonary system. Stroke volume is then derived from the dilution curves obtained [67]. The agreement of cardiac output values measured using transpulmonary and ultrasound indicator‐dilution techniques with those measured using PAC thermodilution was shown to be adequate for clinical use in humans [68–71]. Moreover, both technologies provide volumetric variables in addition to cardiac output, such as global end‐diastolic volume and measurements of extravascular lung water. These variables have been utilized as indicators of fluid responsiveness with promising results [72,73]. PiCCO® and COstatus® appear significantly more practical and user‐friendly than PAC thermodilution. In addition, they do not require any specific customization for use in veterinary species. COstatus® has been shown to be accurate and safe in very small patients [70]. All of these factors may allow a wider use of these systems in veterinary medicine, especially in small animals, when compared to PAC thermodilution [74,75]. The lithium dilution technique is an example of dye dilution cardiac output monitoring, based on intravascular injection of a minute amount of an isotonic lithium chloride (0.002–0.004 mmol/kg) solution used as an indicator [76]. These lithium doses are too small to exert any pharmacological or toxic effects. The lithium concentration in the blood is derived by a lithium‐selective electrode connected to a peripheral arterial catheter. An advantage of the lithium indicator‐dilution cardiac output technique is that no central venous line is necessary for the injection of the indicator, which can be given via a regular peripheral line [77]. The lithium concentration over time curve is recorded by withdrawing blood (4.5 mL/min) through a special disposable sensor, attached to the patient’s arterial line. The voltage signal across the lithium‐selective membrane is converted to a lithium concentration by a computer. Cardiac output is then calculated according to the following equation [76]:
13
Cardiac Output Measurement
Introduction
Species
Stroke volume index (mL/kg/beat)
Heart rate (beats/min)
Cardiac index (mL/min/kg)
Cat
0.90–1.0
180
160–180
Cattle
0.7–0.8
60
35–50
Dog
1.6–1.9
120
190–230
Goat
1.1–1.3
80
85–100
Horse
2.6–3.0
45
115–135
Mouse
1.5–1.8
400
600–720
Rabbit
0.4–0.6
250
100–150
Rat
1–1.2
300
300–360
Sheep
0.7–0.9
80
55–70
Swine
1.2–1.4
100
120–140
Basic principles of cardiac output monitoring
History of cardiac output measurement
Techniques of determination of cardiac output
The Fick principle
O2) [47]:
CO2), from which CO2 content (C
CO2) is calculated. As the patient rebreathes, the level of PETCO2 rises to a plateau corresponding to the partial pressure of CO2 in the blood entering the lungs (or mixed venous blood). At this equilibrium point, the CO2 elimination from the lungs approaches zero, and the partial pressure of CO2 in the end‐pulmonary capillary blood (mixed venous blood) can be assumed equal to PETCO2 [49].
CO2, and CaCO2 are CO2 consumption, mixed venous CO2 content, and arterial CO2 content, respectively.
CO2) will occur, whether under normal or rebreathing conditions. Hence, the above equation is simplified as:
Pulmonary artery catheter thermodilution
O2) [37].
Transpulmonary thermodilution and ultrasound indicator dilution
Lithium dilution
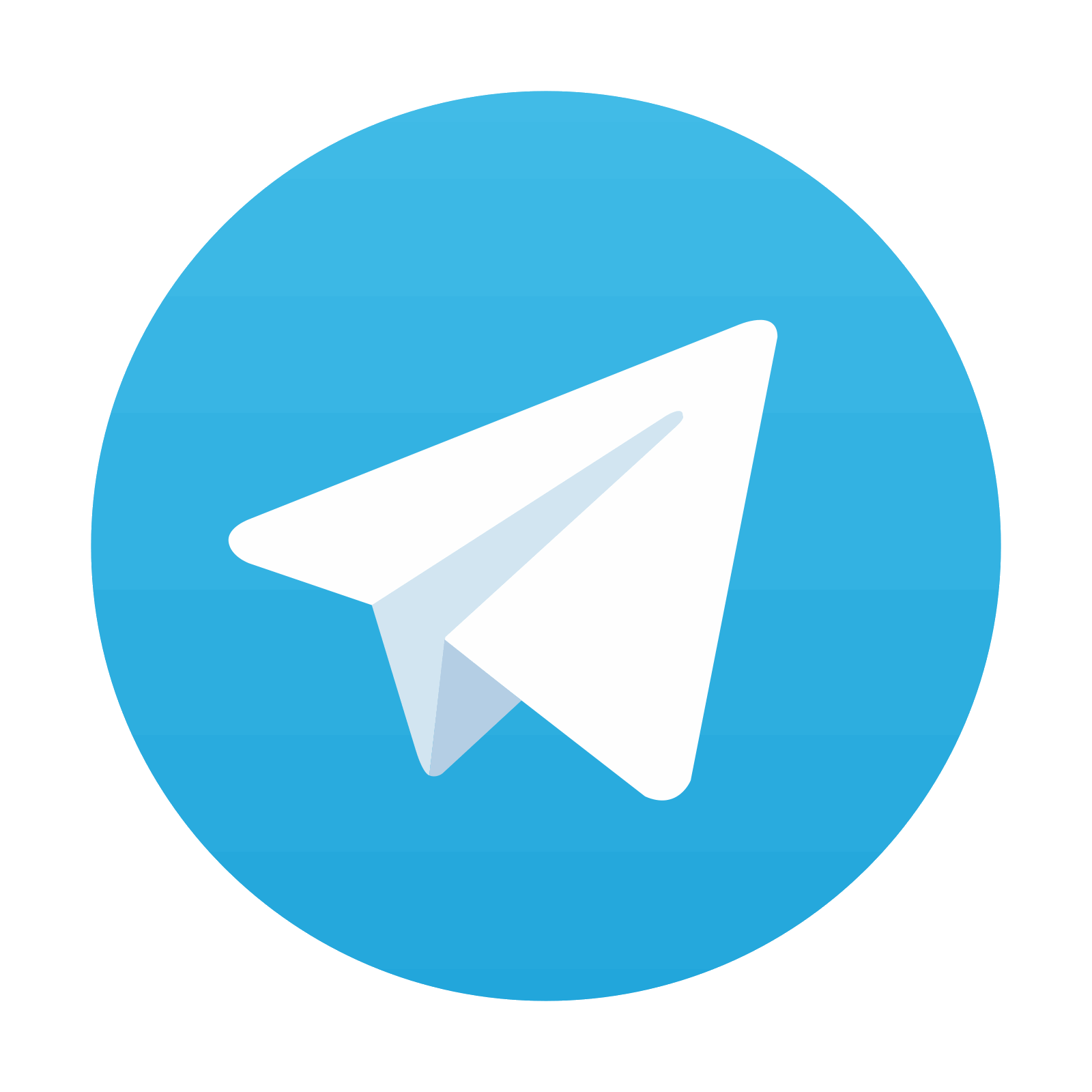
Stay updated, free articles. Join our Telegram channel
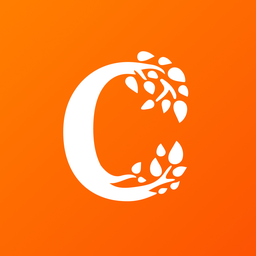
Full access? Get Clinical Tree
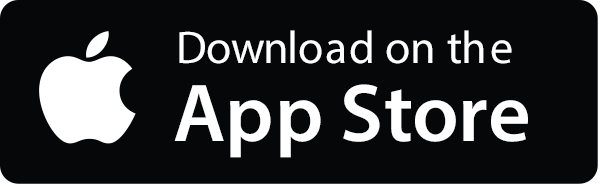
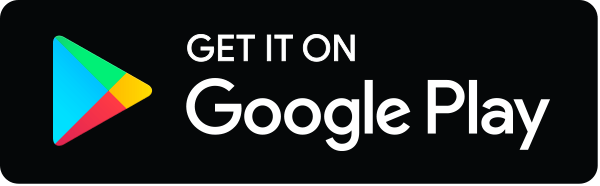