Raphaël Vézina Audette1, Alexander C.S. Thomson2, Ciara A. Barr3, Luis Campoy4, Matt Read5 and Santiago Peralta4 1 Portland Veterinary Emergency and Specialty Care, Portland, Maine, USA 2 City University of Hong Kong Veterinary Medical Centre, Hong Kong SAR, China 3 Department of Clinical Sciences and Advanced Medicine, School of Veterinary Medicine, University of Pennsylvania, Philadelphia, Pennsylvania, USA 4 Department of Clinical Sciences, College of Veterinary Medicine, Cornell University, Ithaca, New York, USA 5 MedVet Columbus, Worthington, Ohio, USA Local and regional (locoregional) anesthetic and analgesic techniques are a cornerstone of modern veterinary anesthetic and analgesic protocols. However, the relevance of locoregional anesthesia extends beyond the perioperative setting to include applications in interventional pain management, both acute and chronic. Due to their unique mechanism of action targeting voltage‐gated sodium channels along nerve fibers, local anesthetics interrupt transmission of neural impulses along axons and contribute significantly to decreasing nociceptive traffic along the pain pathways in both the peripheral and central nervous systems. The benefits of incorporating local or regional blocks into analgesic protocols are many, ranging from profound surgical anesthesia and analgesia, inhibition of central sensitization, a decreased reliance on systemically administered opioids and their associated adverse effects, lowering of inhaled anesthetic requirements for maintenance of a desired surgical plane of anesthesia and, ultimately, a potential for better surgical outcomes with a significant decrease in patient morbidity and mortality. Locoregional anesthetic techniques range in complexity, from simple local infiltration techniques needing minimal equipment and training (e.g., ventral midline infiltration or “line blocks,” ring blocks, and testicular blocks), to more complex ultrasound‐guided regional anesthesia techniques requiring advanced training and mastery of applied functional musculoskeletal and neuroanatomy. Ultrasound guidance has recently been described for a wide variety of peripheral nerve and fascial plane block techniques, allowing for a more precise and targeted delivery of local anesthetic solutions and decreased risks of nerve injury or vascular puncture. In some techniques, ultrasound guidance may be used in conjunction with peripheral neurostimulation to provide further confirmation that targeted structures are properly identified. In veterinary medicine, species‐specific anatomic studies are needed to determine the relevance and safety of various locoregional anesthetic techniques. Extrapolating from descriptions of blocks described in human medical textbooks, or even from one animal species to another, may lead to treatment failures since the comparative neuroanatomy often contains important differences. Preliminary cadaveric studies assessing distribution of injectate and staining of target nerve fibers must be followed by well‐designed, blinded, randomized, controlled clinical trials to evaluate analgesic efficacy. The past decade has seen exponential growth in the number of publications on locoregional anesthesia in veterinary medicine. The low cost of local anesthetics and the positive impact of these techniques on patient welfare make locoregional anesthesia a vital component of many balanced anesthetic protocols. Topical anesthesia is the oldest form of locoregional anesthesia and refers to the direct application of a local anesthetic agent to skin or mucosa to produce superficial desensitization. Topical anesthesia is still commonly used in companion animals; proparacaine and tetracaine are used for short‐term corneal desensitization, and atomized lidocaine is routinely used to desensitize the arytenoids prior to tracheal intubation, particularly in cats [1,2]. However, application of local anesthetics to intact skin is complicated by the presence of the stratum corneum, which limits absorption. Skin preparations of local anesthetics may contain higher concentrations and permeation enhancers such as eucalyptol or propylene glycol [3]. Application of heat, ultrasonic waves, and electrical energy has also been reported to increase absorption of local anesthetics across the skin [3]. In companion animals, a eutectic mixture of lidocaine and prilocaine (EMLA™ cream) is commonly used for venipuncture and superficial skin procedures. The combination of local anesthetics reduces the melting point of each, permitting a higher local anesthetic concentration in the liquid phase. The efficacy and depth of desensitization are dependent on contact time; placing an occlusive dressing over the application site is often recommended to enhance absorption. The use of EMLA™ cream has been reported to significantly reduce stress in cats when applied 30 min prior to jugular venipuncture [4]. In dogs, application of EMLA™ cream 60 min before IV catheter placement reduced behavioral reactions without affecting catheter placement success rate [5]. Transdermal lidocaine patches have been evaluated in dogs, cats, and horses [6–11]. Human studies have demonstrated an analgesic effect and reduced postoperative opioid consumption in patients with lidocaine patches applied [12,13]. However, extrapolation of human data to animals is tenuous due to differences in skin composition that may affect drug absorption and controlled clinical trials have not yet demonstrated a significant analgesic benefit in veterinary patients [8]. Lidocaine patches appear to be well tolerated in dogs and cats with few side effects, low systemic absorption, and a low risk of systemic toxicity [6,9]. It is important to note, however, that the systemic absorption of lidocaine from patches increases in the presence of surgical wounds compared to intact skin [14]. Local infiltration is the deposition of local anesthetic into and around a surgical site, rather than targeting specific neural structures. It may be particularly useful when a regional nerve block is difficult or contraindicated, or when the required equipment for more advanced techniques is not available. However, local infiltration may result in incomplete blockade of sensory fibers supplying the target site due to imprecision and the inhibitory effect of tissue inflammation on local anesthetic efficacy. As a result, clinical studies evaluating the analgesic efficacy of incisional and peri‐incisional blocks in companion animals have demonstrated mixed results [15–19]. Local infiltration is most commonly delivered by injection, but multi‐fenestrated catheters (i.e., “soaker catheters”) may be implanted for repeated or continuous infiltration of a surgical site. In humans, local anesthetic wound infiltration has been associated with a reduction in postoperative opioid requirements, reduced postoperative nausea and vomiting, and a reduced length of hospital stay [20]. A retrospective study in dogs identified catheter disconnection, seroma formation, and local anesthetic systemic toxicity (LAST) as potential complications associated with infiltration catheters but did not find an increased incidence of surgical site infection compared to historical controls [21]. However, controversy remains about the effect of high concentrations of local anesthetics on wound healing. This effect may vary by the specific local anesthetic used, as well as the stage of wound healing. For example, some studies have indicated no effect of lidocaine on wound healing, while levobupivacaine may have a beneficial effect in the early phase and a detrimental effect thereafter [22]. The clinical implications of these studies are unclear in companion animals but, in general, local infiltration appears to be well tolerated with a low incidence of adverse effects. Tumescent anesthesia is a type of local infiltration first developed for liposuction in humans. A large volume of dilute local anesthetic, combined with epinephrine and sodium bicarbonate, is infused into the subcutaneous tissues by a blunt‐ended, multi‐fenestrated Klein cannula [23]. Though high total doses of local anesthetic may be used (up to 55 mg/kg lidocaine in obese humans), the addition of a vasoconstrictor to the tumescent solution limits systemic absorption and reduces the risk of LAST [24]. Tumescent anesthesia has been reported in dogs and cats undergoing mastectomy [25–27]. Dogs receiving 15 mL/kg of a tumescent solution containing 0.16% lidocaine with epinephrine showed no difference in physiologic parameters from dogs receiving an epidural and intercostal nerve block; neither group required rescue analgesia for at least 6 h after surgery [27]. Similarly, tumescent anesthesia consisting of 15 mL/kg of 0.32% lidocaine with epinephrine was effective and well tolerated in a study of 12 cats [26]. The total lidocaine doses reported for tumescent anesthesia were 24 mg/kg in dogs and 48 mg/kg in cats. Intravenous regional anesthesia (IVRA), also known as a Bier block, involves administration of local anesthetic into a peripheral vein, where it is distributed to the tissues served by the local venous drainage. Once the target vein has been catheterized, the limb is elevated, and a tight bandage is placed to exsanguinate the area. A tourniquet is applied proximal to the bandage and the area to be anesthetized and the bandage is removed. Lidocaine 0.5% (0.6 mL/kg) is then injected into the catheter, resulting in desensitization of tissues distal to the tourniquet. IVRA can be used for short (< 90 min) procedures of the distal extremities, particularly when minimal hemorrhage is desirable. In one canine study, IVRA with lidocaine was found to provide equivalent intraoperative analgesia to a brachial plexus block for carpal arthrodesis [28]; however, IVRA is better suited to surgeries of shorter duration, such as digit amputation. Application of the tourniquet itself may be a source of pain, particularly ischemic pain transmitted by sympathetic fibers in the adventitia of major arteries [29]. In addition, once the tourniquet is released, transient increases in local anesthetic plasma concentrations can result in signs of systemic toxicity. Strategies to minimize or prevent these complications include limiting the time the tourniquet is in place, releasing the tourniquet slowly at the end of the procedure, and avoiding the use of cardiotoxic local anesthetics such as bupivacaine for this technique. Neuraxial anesthesia dates back to 1885, when neurologist James Corning injected 26 mg of cocaine into the space between two vertebrae of a young dog, resulting in anesthesia of the pelvic limbs lasting 4 h [30]. Further refinement of this experiment led to the development of techniques for administering anesthetic agents into the epidural or subarachnoid spaces. These techniques remain in wide use today in both human and veterinary anesthesia. Epidural anesthesia and analgesia refer to administration of anesthetic agents into the space immediately superficial to the dura mater of the spinal cord. This space is normally occupied by loose connective tissue, adipose tissue, blood vessels, and lymphatics. Subarachnoid anesthesia, also known as spinal or intrathecal anesthesia, refers to administration of drug into the subarachnoid space, between the arachnoid membrane and the pia mater. Unlike the epidural space, the subarachnoid space contains cerebrospinal fluid. Both epidural and subarachnoid anesthesia work by bathing the spinal nerve roots in anesthetic as they leave the spinal cord, inhibiting neural transmission. The magnitude and duration of analgesia are dependent on the physical and chemical characteristics of the anesthetics used, as well as their concentration. However, the mechanism of drug distribution differs between epidural and spinal anesthesia. Whereas the distribution of anesthesia from an epidural injection predominantly depends on the injection site and the volume injected, the distribution of a spinal injection is also dependent on patient positioning and the baricity of the injectate. Baricity refers to the density of the solution compared to the density of cerebrospinal fluid. While commonly performed in veterinary medicine, neuraxial anesthesia requires operator skill and is reliant on somewhat subjective criteria for determining correct placement of the needle, which may vary based on conformation and body condition. As a result, epidural failure rates of 12–30% have been estimated in companion animals [31]. A variety of techniques have been investigated for improving epidural success rate, including use of a nerve stimulator and ultrasound guidance [32–34]. Nerve blocks involve the administration of a local anesthetic adjacent to a peripheral nerve to block conduction. This results in sensory and/or motor deficits, depending on the nerve fiber composition, in the targeted anatomic region due to loss of both afferent and efferent nerve function. A variety of techniques may be used to identify the location of the target nerve(s), including palpation, anatomical landmarks, nerve stimulators, or ultrasound guidance. Although regional anesthesia may be provided utilizing any of these techniques, ultrasound guidance has been shown to improve the anatomical accuracy and success rate of a variety of nerve blocks in both human and veterinary medicine [35–38]. Fascial plane blocks are a relatively new subset of regional anesthetic techniques that involve deposition of a local anesthetic solution in between two layers of fascia, relying on spread of injectate volume within a fascial plane that contains nerves, rather than identification of and injection around discrete nerves [39]. The mechanism by which fascial plane blocks provide analgesia is still not well understood as studies in human medicine have found significant variability in the distribution of analgesia, sometimes even at sites distant from the expected distribution [40]. While most evidence clearly supports an analgesic benefit of fascial plane blocks, these results may indicate additional mechanisms of action are at play, such as systemic absorption of large doses of local anesthetic [41]. When successful, nerve blocks provide complete analgesia by preventing transmission of nociceptive signals to the central nervous system. Due to their superior analgesic effect, regional anesthetic techniques are associated with a greater reduction in the surgical stress response and improved postoperative analgesia when compared to opioid‐based protocols [42–45]. A variety of excellent texts and other tools are available for teaching and learning regional anesthetic techniques in veterinary patients. Nerve impulses are carried by axons (often referred to as fibers) which are long, thin projections of neurons whose cell bodies reside in the sensory/dorsal root ganglia (afferent neurons) or brainstem/spinal cord (efferent neurons). Axons vary in diameter and myelination based on their function and required conduction speed. For example, the Aδ fibers responsible for fast, sharp pain are myelinated and much larger in diameter than the unmyelinated C fibers responsible for slow, aching pain. Each axon is surrounded by a loose, elastic connective tissue structure called the endoneurium, which consists mostly of collagen. Axons are bundled together in fascicles, which are surrounded by a more robust connective tissue sheath called the perineurium. Fascicles divide and converge with each other every few millimeters along the length of the nerve [46]. The outer sheath of the nerve, a continuation of the dura mater containing the nerve’s blood supply, is the epineurium. The relevance of this microanatomy to locoregional anesthesia has been the subject of much research and debate. Intraneural, extrafascicular injection of local anesthetic is associated with extensive longitudinal spread along the nerve without significant nerve damage [47–49]. However, intrafascicular injection of volumes as low as 0.05 mL have been associated with fascicular rupture, demyelination, and nerve damage in rodent models [50], and larger volumes injected proximally may cause inadvertent spinal anesthesia [47]. Though intraneural, extrafascicular injection may result in profound, long‐lasting nerve block, it is not recommended due to the risk of inadvertent intrafascicular injection, as well as the risk of disrupting epineurial blood vessels and compromising nerve perfusion. Nerves are loosely mobile and contain elastic elements, so they can be stretched without damaging the individual axons. They are loosely connected to surrounding structures by a connective tissue meshwork called the paraneurium. Blunt instruments, such as nerve block needles, will often push nerves to the side rather than perforating them. When performing a peripheral nerve block, it is preferable to avoid unnatural positioning that stretches the nerve, as this may increase the risk of inadvertent nerve puncture. A more in‐depth discussion of local anesthetics is presented in Chapter 29. In short, local anesthetics are amphipathic molecules that exert their effects through use‐dependent blockade of voltage‐gated sodium channels. The sodium channels NaV1.7, NaV1.8, and NaV1.9 are implicated in propagating action potentials along nociceptive nerve axons [51], although local anesthetics do not bind exclusively to these subtypes. The relative affinity of local anesthetics for off‐target receptors, such as cardiac ion channels, accounts for the differences in their pharmacologic and toxic effects [52]. Local anesthetics are weak bases with pKa values greater than 7.4, meaning they are partially ionized at physiologic pH. Because uncharged local anesthetic molecules must cross the lipid membrane to access their intracellular binding sites on sodium ion channels, their onset of action is influenced by pKa. For example, local anesthetics with a high pKa, such as bupivacaine (pKa = 8.1), have a longer onset time than those with a low pKa, such as lidocaine (pKa = 7.8). Molecular weight, concentration, and lipid solubility also contribute to local anesthetic onset time and potency. High concentration, lipid solubility, and low molecular weight facilitate diffusion across cell membranes, decreasing onset time [52]. Duration of local anesthetic effect is determined by several factors, including protein binding, site of injection, vasoactivity, and regional vascularity. Drugs with a long duration of action often have a long onset time. Mixing different local anesthetics together to produce both short onset time and long duration has occasionally been advocated, particularly with dental nerve blocks. However, this practice results in unpredictable blockade, as the concentration and pH of each local anesthetic in the mixture are altered. Most literature evaluating local anesthetic mixtures has demonstrated a reduction in block duration without an appreciable improvement in onset time, and this practice is therefore of little value and is not recommended [53,54]. Differential blockade describes the phenomenon that different types of nerve fibers demonstrate variable susceptibility to local anesthetics. This was first demonstrated by Gasser and Erlanger in 1929, who found that sensory fibers were more easily depressed by cocaine than motor fibers [55]. While this effect was initially attributed to the smaller size of sensory fibers, subsequent research demonstrated that A fibers are more susceptible to local anesthetic block than C fibers, seemingly at odds with clinical observations [56,57]. In practice, sympathetic fibers are most susceptible to blockade, followed by pain, touch/proprioception, and finally motor fibers. This indicates that several factors besides fiber diameter contribute to local anesthetic susceptibility, including the position of the fibers within the nerve (mantle effect), myelination, and density of ion channels in the axonal membrane. Small, unmyelinated nerve fibers may have a shorter critical length of local anesthetic exposure required to produce conduction block [58]. In addition, some local anesthetics, such as bupivacaine and ropivacaine, exhibit intrinsic differential blocking properties at low concentrations, preferentially producing sensory blockade [59,60]. Adjunctive medications may be added to local anesthetic to potentiate or prolong sensory blockade or reduce the total dose or concentration of local anesthetic required for locoregional anesthetic techniques. High total doses and concentrations of local anesthetics are associated with increased incidence of nerve block‐associated complications and neurotoxicity, so multimodal perineural analgesia is desirable [61]. A wide variety of different drugs, such as opioids, adrenergic agonists, corticosteroids, buffers, ketamine, and hyaluronidase have been studied as local anesthetic adjuvants. Opioid receptors are synthesized in the dorsal root ganglia and transported peripherally along the axons of afferent nerves, especially after inflammation or tissue injury [62]. These peripheral opioid receptors may serve as analgesic targets for locoregional anesthetic techniques since their activation decreases the excitability of nociceptor terminals. However, not all opioids are equally effective in prolonging the duration of peripheral nerve blocks. Lipid solubility and ionization state likely influence the ability of a particular opioid to penetrate the nerve sheaths and access opioid receptors at the axonal membrane [63]. Buprenorphine, a highly lipid‐soluble opioid, has been demonstrated to significantly prolong the effect of peripheral nerve blocks in humans [64–66]; however, opioids with lower lipid solubility, such as morphine, or a higher degree of ionization, such as fentanyl, are less likely to be effective. The synergistic effect of buprenorphine may also be attributable to its local anesthetic effects, as it produces use‐dependent blockade of voltage‐gated sodium ion channels [67]. Epinephrine has a long history as an additive to local anesthetics. Its vasoconstrictive effect counteracts the vasodilation produced by many local anesthetics, therefore decreasing systemic absorption and prolonging the duration of blockade. It may also contribute directly to analgesia through activation of α2‐adrenergic receptors in the axons of peripheral nerves. Epinephrine is commonly added in a concentration of 2.5–5 μg/mL. In combination with local anesthetics, epinephrine may reduce blood flow to peripheral nerves, although this effect varies with the dose and specific local anesthetic used [68]. It should be used with caution in extremities as, in rare cases, local vasoconstriction may impair perfusion in areas with limited collateral circulation [69]. α2‐Adrenergic receptor agonists are commonly added to local anesthetics. While α2‐adrenergic receptor agonists may also produce local vasoconstriction, plasma concentrations of local anesthetic are similar with and without the addition of an α2‐adrenergic receptor agonist, suggesting similar systemic absorption [70]. Additional mechanisms, such as direct suppression of A and C fiber activity, likely account for their synergistic analgesic effect [71]. Clonidine is widely used in human regional anesthetic techniques and is associated with improved quality and duration of blockade [70–72]. Dexmedetomidine is more commonly used in veterinary medicine and has demonstrated similar beneficial effects in human patients [73]. The majority of nerve blocks in dogs and cats are performed under either sedation or general anesthesia. Patients should be relaxed, easy to manipulate and position, and either minimally or completely unresponsive to needle advancement, electrolocation, and injection. Patient positioning is an important aspect of any regional anesthetic procedure since nerves are flexible structures whose locations can vary depending on the patient’s position. Recommended approaches for different nerve blocks may or may not be possible depending on the patient’s position and, in some instances, patient positioning may also influence the distribution of drugs following injection. Using described and standardized positioning may minimize complications. Thorough medical history‐taking and physical examination should be performed prior to sedation or anesthesia on candidates for regional anesthetic techniques to document any pre‐existing condition that may complicate or contraindicate locoregional blocks, such as known or presumed hypersensitivities, peripheral neuropathies or, in the case of neuraxial blocks, hypovolemia, coagulation disorders, or infection or neoplasia involving the puncture site. Clinicians should evaluate sensory and motor function prior to performing any nerve block. An overview of the regional anesthesia technique should be provided to the patient’s owners when obtaining informed consent. The anesthetist should also discuss the risk of potential complications with the members of the healthcare team, including the patient’s owners. Injection sites should be aseptically prepared to decrease the risk of infection. Equipment and agents for the locoregional technique should be selected and readied ahead of time to limit unnecessary delays and improve patient safety. Historically, equipment for companion animal regional anesthesia has often been sourced from human medical supply companies. However, as interest in advanced pain management techniques for animals expands, specialized equipment designed for veterinary use is becoming more commonly available. A wide variety of equipment may be encountered in veterinary practice, with variable price and quality. Continued development of veterinary‐specific products is likely to improve utility and patient safety. Needles are used to deliver local anesthetic and other drugs, but they may serve additional purposes, such as aiding in nerve location or catheter implantation. The utility of a particular needle for these purposes is dependent on its design and physical characteristics. Needles are available in many different lengths and gauges with varying tip designs and, in the case of ultrasound‐guided techniques, echogenicity. They may be insulated or non‐insulated, depending on whether they have a plastic, non‐conducting sheath coating the shaft of the needle. In general, longer needles are used when targeting deeper structures, but they may be more challenging to manipulate and prone to bending. Selecting a larger gauge needle reduces the flexibility of the needle, but larger gauges are associated with more tissue trauma and injury of nerves that are inadvertently punctured [74,75]. Smaller needles may be prone to breaking or bending, especially if manufacturing defects are present, the patient moves during the procedure, or an incorrect technique is used [76,77]. The design of the needle tip may aid the anesthetist in estimating the position of the needle (Fig. 60.1). Hypodermic needles have long, acutely angled (12–15°) bevels designed to easily cut through tissue. This is not a desirable feature of a needle used for peripheral nerve blocks since this tip design provides little tactile feedback and is more likely to injure delicate nerves [78]. Needles designed for peripheral nerve blocks have short, blunt (30–45°) bevels that encounter variable resistance as the needle is advanced through tissue layers. This design is less likely to cut or puncture through the perineurium if a nerve is accidentally contacted [79]. The tip of Tuohy needles is blunt and rounded which, when used to perform an epidural injection, may convey a popping sensation when the needle passes through the ligamentum flavum and enters the epidural space. Figure 60.1 Needle tips at 25× magnification. A. and B. 22 gauge hypodermic needle; C. and D. 20 gauge insulated nerve block needle; E. and F. 20 gauge Tuohy needle. Note the short bevel of the insulated needle and the round, blunt tip of the Tuohy needle when compared with the hypodermic needle. Insulated stimulating needles are coated with a non‐conducting material along the entire length of the shaft, which allows precise application of electrical current at the tip of the needle for nerve location. Whereas a non‐insulated needle may cause muscle or nerve stimulation along the entire length of its shaft, an insulated needle only stimulates structures in close proximity to the tip. Non‐insulated needles are therefore less precise in terms of confirming that the tip is close to the target nerve and require a higher threshold stimulation current to elicit a motor response. Stimulating needles are manufactured with a cable that connects to a nerve stimulator and often have an extension line that may be connected to a syringe. During ultrasound‐guided procedures, visualization of the needle is important both for the success of the nerve block and patient safety. Poor visibility of the needle tip may result in inadvertent damage to the nerve or other nearby structures. Various methods of mechanical and optical needle guidance have been developed to improve accuracy, such as needle guides that attach directly to the ultrasound transducer. Echogenic needles have etched textures along their shafts, providing multiple reflective angles that increase ultrasonic visibility [80]. This is particularly helpful when a steep insertion angle is required to reach the target nerve, since visibility decreases as ultrasound waves are reflected away from the transducer [81]. Advances in both ultrasound and needle technology promise further improvement in needle visualization. Ultrasound‐actuated needles that vibrate and produce a color Doppler signal are currently under investigation [82]. A photoacoustic needle that produces its own ultrasound waves via pulsed laser and an intraluminal optical fiber has also been reported [83]. Spinal needles are designed to puncture the dura mater and access the subarachnoid space for delivery of drugs or to sample cerebrospinal fluid. While more blunt than hypodermic needles, spinal needles are sharper than needles that are specifically designed for epidural use; therefore, using spinal needles for epidural injection may increase the risk of inadvertent dural puncture depending on the site of injection. Most spinal needles contain a close‐fitting stylet that prevents occlusion of the lumen with a tissue plug as the needle is advanced. The hub of a spinal needle is usually transparent, allowing the operator to watch for the appearance of spinal fluid or blood as the needle is advanced. Many different tip designs have been developed, but the most common currently in use are Quincke, Sprotte, and Atraucan® spinal needles. The Quincke needle, first developed by German surgeon Heinrich Quincke in 1891, has a short V‐shaped cutting bevel [84]. It remains the most common spinal needle in use today but may be associated with a higher incidence of post‐dural puncture headache due to transdural fluid leakage [85]. The Sprotte needle is a modification of the earlier Whitacre (or pencil‐point) spinal needle, and has a long, blunt conical shape tapering to a sharp point. The distal orifice is on the side of the tip, rather than at the point, and is larger than the original Whitacre design. This design is intended to separate dural fibers laterally, rather than cutting through them, allowing them to seal over the puncture site when the needle is withdrawn [86]. However, because the distal orifice is proximal to the tip of the needle, it is possible for the tip and the orifice to be located in separate compartments, especially in very small patients. The Atraucan® spinal needle is a proprietary Huber‐type design introduced in 1993. The Atraucan® needle produces a small, linear cut in the dura, rather than the V‐shaped cut produced by Quincke needles, resulting in less transdural fluid leakage. Unlike the Sprotte needle, the distal orifice of the Atraucan® needle is located at the tip. However, it has a sharp, delicate tip that is prone to being damaged [86]. Tuohy needles are designed specifically for epidural use, but they can also be used for peripheral nerve blocks or catheter insertion. As with spinal needles, Tuohy needles have a close‐fitting removable stylet and a transparent hub. Tuohy needles have a curved, blunt bevel and are typically marked every 10 mm along the shaft. This design provides high‐fidelity tactile feedback, minimizes the risk of inadvertent dural puncture, and directs catheter insertion. Both uninsulated and insulated Tuohy needles are available. The Weiss epidural needle is a modification of the Tuohy needle that adds wings to the needle hub. These wings are grasped between the thumb and index finger during placement to stabilize and direct the needle. Some needle designs have removable wings that slot into place on the hub. Many different types and brands of catheters are available for continuous or intermittent locoregional anesthetic techniques. They may be characterized by their material, length, gauge, method of insertion, number of fenestrations, and additional functions such as electrical stimulation. Most catheters used in locoregional anesthesia are through‐the‐needle, but over‐the‐needle catheters are also commercially available, as well as systems that use an over‐the‐needle cannula as an introducer for the catheter. A variety of needle types and designs are used as described above. Once inserted using aseptic technique, the needle is removed, and the catheter is connected to an injection port and 0.2 μm bacterial filter, secured with suture, and covered with a protective dressing. The catheter may be tunneled subcutaneously near the insertion site for additional security. Complications related to the use of catheters in locoregional anesthesia may be equipment‐ or drug‐related. Catheter dislodgement, disconnection, and occlusion are relatively minor incidents that may require removal or replacement of the catheter. Breakage of catheters is a rare but potentially problematic complication, although surgical removal of the broken fragment is not usually indicated if the fragment is sterile and inert [87]. Withdrawing a catheter through the needle during placement may increase the risk of catheter breakage, since the catheter may shear on the needle bevel. Drug‐related complications may be more serious, especially if cumulative local anesthetic doses exceed toxic doses. Careful calculation and confirmation of local anesthetic doses is essential to minimize these risks and, although it is a rare event, care providers should be well versed in the signs and treatments for local anesthetic systemic toxicity (LAST). Modern epidural catheters are often composed of materials with high melting points, high tensile strength, and low friction, such as nylon blends or polytetrafluoroethylene (Teflon). Most are radiopaque, and some brands contain coiled wire for added strength and kink resistance [88]. MRI compatibility varies by manufacturer. Epidural catheters are often supplied in kits containing the catheter itself, a Tuohy needle, an adapter, an injection port, and a bacterial filter. Epidural catheters are usually long and thin, so resistance to injection is much higher than would be expected with a normal epidural injection. Microcatheters are available for spinal use; however, continuous spinal anesthesia is less commonly performed due to the higher risk of neurotoxicity and detrimental hemodynamic effects [89]. Continuous nerve block catheters evolved from epidural catheters, and share similar qualities of heat resistance, strength, and low friction. Like epidural catheters, these catheters are commonly supplied in kits containing all necessary equipment for implantation. Some kits contain an insulated needle with an attached port through which the catheter can be passed. This allows simultaneous nerve stimulation, injection, and feeding of the catheter without disconnecting the extension line and potentially moving the position of the needle. Nerve block catheters may have additional features, such as incorporated stimulating wires to verify correct positioning and echogenic reflectors to improve ultrasound visibility. Self‐coiling “pigtail” catheters have been described for some types of continuous nerve blocks to prevent displacement of the catheter from the target injection site [90]. Multi‐fenestrated catheters have many small openings at the distal end of the catheter, providing better dispersion of local anesthetic in the target area. Dispersion is primarily dependent on the number, size, and position of fenestrations [91]. Rate of drug administration may also affect dispersion, as bolus doses tend to disperse more homogeneously than slow infusions [92]. Wound soaker catheters are typically long, flexible, closed‐end polyurethane catheters with multiple side fenestrations to allow for wide distribution of injected local anesthetic. They allow for continuous or intermittent delivery of local anesthetic as part of a multimodal postoperative analgesic strategy [21]. In dogs, the use of bupivacaine through wound soaker catheters has been associated with lower pain scores and analgesic requirements and improved appetite compared to a saline control [93]. Typically, wound soaker catheters are implanted with the tip positioned in the deepest layer of the surgical site during closure, with the proximal end exiting dorsally through a separate skin incision. However, they may be placed in a variety of locations, including subcutaneous, subfascial, intra‐articular, periosteal, and peripleural. While purpose‐made wound soaker catheters are commercially available, they can also be improvised from red rubber and other similar catheters by heat sealing the distal opening and creating fenestrations with a small (25–27 gauge) needle. However, cutting additional fenestrations into a red rubber catheter may compromise its structural integrity, increasing the risk of breakage within the patient. While injection pressure is partially dependent on equipment variables such as needle gauge and length, changes in injection pressure may be associated with injection into different tissues [94]. Nerve injury due to intrafascicular injection has been associated with high injection pressure (25–45 psi), while perineural and epidural injections are associated with relatively low injection pressure [95–97]. Therefore, monitoring injection pressure may be useful for both safety and accuracy of locoregional techniques. Three major types of injection pressure monitors are currently available [98]. The first is the loss of resistance syringe, which relies on Boyle’s law where the compression of a known volume of air in the syringe is used to estimate injection pressure; greater bubble compression is associated with higher injection pressure [98]. This method is commonly used for epidural techniques, as a significant drop in injection pressure occurs as the tip of the needle crosses the ligamentum flavum and enters the epidural space. Saline is commonly added to the syringe as well to prevent injection of air into the epidural space, which may be associated with complications such as venous air embolism or nerve root compression [99]. The second type of injection pressure monitor is the electronic manometer in syringe pumps; these devices are primarily used to detect occlusions during constant rate infusions and may have adjustable alarm thresholds. A syringe pump designed for regional anesthesia procedures, the Medovate SAFIRA®, is operated by a foot pedal and injects local anesthetic automatically while monitoring injection pressure. The third category of injection pressure monitors is inline devices. Two inline pressure monitors that are commercially available are the B. Braun B‐Smart™ and the PAJUNK® NerveGuard. Both devices are attached between the syringe and the extension line of the needle. The B‐Smart™ consists of a rising piston which displays the approximate pressure in psi, while the NerveGuard has a spring device that automatically blocks injection once a threshold pressure is exceeded. An injection pressure monitor integrated into the needle tip has been described but is not yet commercially available [100]. Peripheral nerve stimulators deliver intermittent square‐wave electrical currents via stimulating needles, eliciting specific muscular twitch responses to aid nerve location. They can be used in conjunction with ultrasound guidance. The minimum current required to elicit a response depends on the distance between the tip of the needle and the nerve. This relationship is described by Coulomb’s law, where the required stimulating charge is related to the electrode‐to‐nerve distance [101]. Therefore, as the tip of the stimulating needle approaches the nerve, a lower current is required to stimulate it, and current can be used to approximate needle‐to‐nerve distance. It was previously thought that intraneural injections could be avoided by closely monitoring the minimum stimulating current. However, studies evaluating simultaneous nerve stimulation and ultrasound guidance have found that the minimum stimulating current actually increases when the needle is in direct contact with the nerve; therefore, relatively high minimum stimulating currents do not rule out the possibility of intraneural injection [102–104]. Current channeling has been proposed as an explanation for this phenomenon [105]. The susceptibility of a particular nerve fiber to stimulation can be described by two basic properties, rheobase and chronaxie. Rheobase is the minimum electrical current required to produce a sustained action potential and reflects membrane excitability. Chronaxie is the minimum impulse duration required to stimulate the nerve at a current twice the rheobase. Because chronaxie is influenced by axon diameter and myelination, chronaxie is typically shorter for motor fibers compared to sensory fibers. This difference allows peripheral nerve stimulators to selectively stimulate motor fibers using short‐duration impulses. Impulse duration delivered by most peripheral nerve stimulators used for locoregional anesthesia is approximately 0.1 ms. Peripheral nerve stimulators should have an easily readable display and the ability to adjust the current (0.1–1.0 mA) and frequency of stimulation. Some peripheral nerve stimulators incorporate a remote control or foot pedal to allow the operator to make adjustments without breaking sterility. Because currents as low as 0.2–0.3 mA may be used when locating peripheral nerves, nerve stimulators used for monitoring neuromuscular blockade may not be suitable for use in regional anesthesia. Terminology describing the polarity of peripheral nerve stimulator components may be confusing and counterintuitive at first. Peripheral nerve stimulators use cathodal stimulation, meaning the negative electrode (cathode) is the searching electrode (i.e., the stimulating needle or catheter), and the positive electrode (anode) is the returning electrode. Current in the form of electrons flows from the cathode to the anode; as the cathode approaches the nerve, the density of negative charges on the outer surface axonal membrane increases, inducing nerve depolarization. If the electrodes were reversed, hyperpolarization of the nerve would occur due to accumulation of positive extracellular charge [106]. In the case of modern peripheral nerve stimulators, the cathode and anode have non‐interchangeable connectors to prevent them from being inadvertently switched. The electrical resistance encountered by the stimulating needle changes as it passes through body tissue. Therefore, peripheral nerve stimulators must continuously adjust their voltage to maintain a constant current in accordance with Ohm’s law. However, the electrical characteristics and performance of commercially available peripheral nerve stimulators are highly variable, and anesthetists should be aware of the limitations of the equipment they are using [107–109]. Error often increases with high resistance and low current [109]. Modern peripheral nerve stimulators often incorporate a flashing light or audible tone change if the circuit is disconnected or for some other reason the indicated current cannot be delivered [106]. Peripheral nerve stimulators should be used with insulated needles so that the application of electrical current is precise. The negative electrode (i.e., black, cathode) connects to the stimulating needle, while the positive electrode (i.e., red, anode) attaches to the patient’s skin, usually by an alligator clip or electrode patch. With modern nerve stimulators, the positive electrode can be attached anywhere on the patient provided good contact is achieved. The frequency of the nerve stimulator should be set to 2 Hz if the stimulator is being used alone, or 1 Hz if used in conjunction with an ultrasound to minimize disruption of the image. The insulated needle is inserted through the skin using anatomic landmarks or ultrasound guidance, depending on the specific technique being used. The initial current should be set to 1.0–1.5 mA, depending on the depth of the targeted structure. The needle is then slowly advanced, while observing for the desired muscular response(s); twitches elicited by direct muscle stimulation should not be interpreted as success. If no twitches are observed, the needle is withdrawn to the level of the skin and redirected. Once a twitch is obtained, the current is slowly decreased to zero, making small adjustments to maintain the muscular response. The current is then slowly increased until a minimum stimulating current of < 0.5 mA is achieved, at which point the local anesthetic can be delivered. The syringe is gently aspirated to check for blood and local anesthetic solution is injected, monitoring closely for high injection pressure. If blood is aspirated or resistance to injection is encountered, the needle should be repositioned before injecting. As local anesthetic solution is injected, the conductive area around the tip of the needle expands, and the low current used for stimulation is dispersed. This usually leads to a loss of the muscular response and does not indicate that the block has taken immediate effect. Nerve blockade performed using visual ultrasound guidance was first reported in 1994 when Kapral et al. used the technique for supraclavicular brachial plexus block in humans [110]. Since then, ultrasound guidance has revolutionized the field of locoregional anesthesia, improving the safety and accuracy of many different nerve blocks in both human and veterinary medicine. Ultrasound is now commonly used to identify peripheral nerves, as well as other anatomic landmarks such as blood vessels, bones, muscles, and fascial planes. It may be used in conjunction with nerve stimulation to further enhance safety and accuracy. Besides regional anesthesia, ultrasound has many useful roles in anesthesia, such as aiding vascular access, assessment of cardiac and diaphragmatic function, diagnosis of pneumothorax or effusions, and others. However, its utility is dependent on selection of an appropriate ultrasound machine, transducer, and settings, as well as the skill and ability of the operator to correctly identify anatomy. Ultrasound transducers convert electrical energy into sound waves using piezoelectric materials, such as quartz crystals, that vibrate when current is applied. These sound waves are transmitted into the body at a particular frequency and are then reflected back toward the transducer. The reflected information is simultaneously processed to create an image [111]. Transducers may be linear or curved, and are available in a wide variety of frequencies, shapes, and widths. Frequency is directly related to axial resolution and inversely related to penetration depth [111]. High‐frequency linear transducers are most commonly used to perform ultrasound‐guided nerve blocks, as they produce high‐resolution images; however, lower‐frequency curved transducers are occasionally used when targeting deeper structures. Conventional B‐mode ultrasound generates an image based on a single beam angle at a frequency designated by the transducer. However, this mode of imaging is particularly susceptible to speckle, reflection, and shadowing artifacts [112]. These artifacts may make small structures difficult or impossible to distinguish when ultrasound machines that employ conventional B‐mode imaging are used. Advances in ultrasound technology have led to the creation of spatial compound and tissue harmonic imaging to reduce artifacts and improve image quality. Spatial compound imaging acquires overlapping frames from multiple frequencies or beam angles, collating them into a single image. In practice, this leads to an averaging of artifacts across the multiple images acquired, reducing noise and clutter in the compound image. Tissue harmonic imaging collects information from harmonic frequencies, which are multiples of the fundamental transducer frequency [113]. Combining tissue harmonic and compound imaging produces better axial resolution and image quality than either modality alone [114–116]. Doppler ultrasonography uses shifts in ultrasound wave frequency to detect movement, as with blood flow in vascular structures or spread of local anesthetic during injection. It is displayed as a color signal overlying the grayscale ultrasound image, with the color indicating the direction of movement with respect to the transducer. Doppler ultrasound is often used in regional anesthesia to identify vascular structures and distinguish them from nerves; however, it may reduce temporal resolution due to its low frame rate. Reducing the size of the color window may minimize this effect [111]. To reduce color artifact, Doppler velocity should be set between 15 and 35 cm/s [112]. Ultrasound transducers should be held with a low grip to facilitate fine movements. Hydrophilic gel or alcohol is used for acoustic coupling, and enough pressure should be applied to maintain adequate contact without collapsing veins or distorting anatomic landmarks. Movements to scan the anatomy may include sliding, rotating, or tilting the transducer [117]. The ultrasound machine should be positioned ergonomically within the operator’s direct line of vision. Depending on the specific technique used, the needle may be introduced in‐plane, in which the long axis of the needle is aligned with the transducer, or out‐of‐plane, in which the long axis of the needle is perpendicular to the transducer. Shallow insertion angles provide better needle visualization than steep angles due to reflection of more ultrasound waves back toward the transducer [118]. The region of interest should be positioned in the center of the ultrasound image, where surrounding structures and their spatial relationships can be seen clearly, and within the transducer’s focal zone. The gain, or brightness of the image, should be adjusted to provide the best contrast between the nerve and surrounding structures. Peripheral nerves are variable in appearance on ultrasound, but generally consist of a hyperechoic epineurium surrounding a hypoechoic center. The short axis may be round, oval, or spindle‐shaped, depending on the location and the nerve. Individual hypoechoic nerve fascicles surrounded by hyperechoic perineurium are sometimes seen with high‐resolution ultrasound. Nerves often lie in close proximity to blood vessels, which are readily identifiable with color Doppler. Anatomic variation and imaging artifacts may be disorienting to novice operators; therefore, a thorough understanding of regional anatomy and familiarity with ultrasound imaging is essential for the safety and success of ultrasound‐guided techniques [119,120]. Figure 60.2 Distribution of the maxillary (V2) and mandibular (V3) branches of the trigeminal nerve (cranial nerve V). Oral, maxillofacial, ophthalmic, and auricular diseases requiring surgical intervention are common in small animals. Nerve blocks are widely used and indicated for the prevention and relief of pain associated with maxillofacial and dental surgery [121,122]. Correctly administered regional anesthesia reduces systemic anesthetic and analgesic requirements and is associated with improved postoperative analgesia and attenuation of the surgical stress response [44,123,124]. Common indications for local and regional anesthesia of the head in small animals include dental and oral surgery, maxillomandibular fracture repair, and ophthalmic surgery. The implementation of local and regional anesthesia requires a thorough understanding of the relevant anatomy. The majority of sensory innervation to the head is supplied by the trigeminal nerve (cranial nerve V), with small caudal contributions from branches of the facial nerve (cranial nerve VII), and cervical spinal nerves [125]. The trigeminal nerve divides into the ophthalmic (V1), maxillary (V2), and mandibular (V3) branches. The sensory innervation to the oral cavity, including all the intraoral structures, is provided by the mandibular and maxillary divisions and their corresponding branches (Fig. 60.2). Selection of a regional anesthetic technique should be predicated on the procedure and anatomy of the individual patient. Advanced imaging, such as computed tomography, may aid in both surgical planning and the selection of a regional anesthetic technique. As with any technique, care should be taken to avoid passing the needle through diseased or neoplastic tissue. All patients receiving regional anesthesia should be monitored for signs of systemic local anesthetic toxicity, though it is rare with appropriate dosing and aspiration to avoid intravascular injection [126]. Needle sizes ranging from 1 to 1.5 inch, 25 to 27 gauge, have been recommended [121]; needles should be flexible and ideally have a short, atraumatic bevel [127]. Because the tips of these needles become easily blunt or bent, the needle that is used to draw the anesthetic from the vial should not be used to administer the block [128]. Additionally, a new needle should be used for each site when performing more than one block. The maxillary nerve provides sensory innervation to the maxillary teeth and associated hard and soft tissues, hard and soft palates, nose, and lower eyelids. It exits the cranium via the round foramen and courses rostrally along the dorsal surface of the medial pterygoid muscle toward the pterygopalatine fossa (Fig. 60.3). At that point, the zygomatic and pterygopalatine nerves arise, and the maxillary nerve continues its course as the infraorbital nerve, entering the infraorbital canal via the maxillary foramen. The pterygopalatine nerve gives rise to the minor and major palatine nerves. These emerge at the palatal area via the corresponding foramina, and provide innervation to the soft and hard palate, respectively [125]. The caudal, middle, and rostral superior alveolar nerves arise from the infraorbital nerve. The caudal superior alveolar nerves arise in the pterygopalatine fossa before the infraorbital nerve enters the canal, and they supply the first and second molar teeth. Within the infraorbital canal, the middle and rostral superior alveolar nerves emerge; these nerves supply the rostral premolars via numerous foramina located on the floor of the canal, and the canine and incisor teeth via the maxillary incisive canal. The infraorbital nerve exits the infraorbital canal via the infraorbital foramen, and gives rise to the external nasal, internal nasal, and superior labial branches [125]. Figure 60.3 Caudal view of the pterygopalatine fossa. The maxillary foramen is the larger oval‐shaped foramen in the center of the fossa and represents the caudal opening of the infraorbital canal. When the maxillary nerve is blocked, the entire ipsilateral maxillary quadrant is anesthetized, including all teeth, associated gingiva, alveolar bone, dental pulp, and the hard and soft palates. Other maxillofacial structures anesthetized include part of the nasal mucosa (excluding the septal mucosa served by the ethmoidal nerve) and the upper lip [129–131]. The indications for a maxillary block or rostral blockade of the infraorbital nerve depend on the area of desired local anesthetic coverage and include ipsilateral maxillary dental extractions, oral surgery, and endodontic therapy, maxillectomy, incisivectomy, rhinotomy, rhinoscopy, and palatal surgery [121,132]. Several techniques have been described to block the maxillary nerve and its more distal branches and detailed descriptions are provided elsewhere [121,130,132]. Some of the techniques approach the nerve extraorally while others utilize an intraoral approach. Dependent on the technique utilized, the patient may be positioned in dorsal, sternal, or lateral recumbency. A subzygomatic approach to the maxillary nerve has been described; however, the authors recommend caution with this advanced technique due to the risk of salivary gland and maxillary artery puncture, as well as nerve trauma, associated with the perpendicular approach to the nerve [121,131,133]. Similarly, the approach to the maxillary nerve via the maxillary tuberosity (or caudal intraoral) approach is associated with risks of accidental penetration of the orbit, possible perpendicular contact with the maxillary nerve, and injury to the maxillary artery and zygomatic salivary gland [132]. There are no clinical studies documenting the efficacy and safety of the maxillary tuberosity technique. The rostral branches of the maxillary nerve can also be blocked using an infraorbital approach. The infraorbital foramen may be palpated extra‐ or intraorally. In dogs, the foramen is consistently located dorsal to the maxillary third premolar tooth and is easily palpated as an oval‐shaped structure. In cats, the foramen is located immediately medial to the prominent rostral end of the zygomatic arch [121,134]. After the infraorbital foramen has been identified, the dominant hand is used to advance the needle caudally on a horizontal plane, maintaining it parallel to the hard palate (Fig. 60.4A) [132]. Following careful aspiration to ensure an absence of vascular puncture, a volume ranging from 0.1–1 mL, dependent upon size and conformation, of 0.5% bupivacaine may be injected. In cats and brachycephalic dogs, a short needle is recommended. Larger dogs may have a canal longer than 2 cm, so a longer needle or catheter is necessary to reach the targeted area [121]. It is important to note that insertion of the needle into the full length of the infraorbital canal should be avoided due to the risk of damage to the vascular structures within the canal or, in the case of brachycephalic or feline patients, orbital structures. One safe alternative is to advance an intravenous catheter off the stylet following entrance into the canal to deliver local anesthetic (Fig. 60.4B). The infraorbital approach offers several advantages over other techniques used to block the maxillary nerve. First, it ensures that the needle is advanced accurately to the desired location. Also, the tip of the needle advances parallel to nerves, decreasing the risk of nerve damage by avoiding perpendicular contact. Moreover, because the canal guides the needle, damage to the zygomatic salivary gland is unlikely. Evidence suggests that this technique is safer and more effective than the lateral approach in both cats and dogs; however, the infraorbital approach may not consistently anesthetize the maxillary molar area if the anesthetic agent is deposited in the infraorbital canal and not in the pterygopalatine fossa [121,135]. It is also possible that this is due to collateral innervation to the associated alveolar mucosa and gingiva via the buccal nerve (branch of the mandibular nerve) [129]. A technique to block the buccal nerve in small animals has not been documented. Hematoma formation at the infraorbital foramen area is a frequent but usually minor complication that results from accidental laceration of the infraorbital artery or one of its branches, or the accompanying vein [121,134]. Immediate treatment is usually limited to applying manual pressure over the area to promote hemostasis. The mandibular nerve exits the cranium via the oval foramen and courses rostrally on the medial aspect of the temporomandibular joint, giving rise to the buccal and masticatory nerves. The masticatory nerve provides motor innervation to the rostral belly of the digastricus muscle; the buccal nerve crosses the pterygoid muscles toward the lateral aspect of the ramus and provides sensory innervation to the vestibular mucosa and skin ventral to the zygomatic bone. As the mandibular nerve continues its course rostrally, it gives rise to the lingual, mylohyoid, and inferior alveolar nerves. The mylohyoid nerve supplies the rostral belly of the digastricus muscle and the mylohyoid muscle. The lingual nerve supplies the rostral two‐thirds of the tongue while the sublingual nerve, a branch of the lingual nerve, supplies the sublingual mucosa. On the lateral aspect of the medial pterygoid muscle, the mandibular nerve becomes the inferior alveolar nerve, coursing rostrally toward the mandibular foramen. At that point, the inferior alveolar nerve penetrates the mandibular canal via the mandibular foramen, where it provides sensory fibers to the teeth before further ramifying into the caudal, middle, and rostral mental nerves, which exit the mandibular canal via the corresponding mental foramen [125]. Figure 60.4 A. Caudal view of the infraorbital canal with a hypodermic needle inserted into the canal. B. Lateral view of the infraorbital canal with an intravenous catheter threaded into the canal for single delivery of local anesthetics. Source: Dr. Raphaël Vézina Audette. Blocking the inferior alveolar nerve provides regional anesthesia to the ipsilateral mandibular quadrant, including teeth, alveolar bone, and gingiva. The indications for an inferior alveolar nerve block include ipsilateral mandibular dental extractions and oral surgery, endodontic therapy, biopsies, and mandibulectomy [121,132]. The mental nerves supply the lower lip and rostral intermandibular area [125]. Ideally, the patient should be placed in dorsal or lateral recumbency to allow for successful palpation of landmarks as well as needle advancement. The mandibular foramen can be palpated intraorally using the index finger of the non‐dominant hand (Fig. 60.5). The foramen is located on the medial and ventral portion of the ramus, between the third mandibular molar tooth and the angular process (Fig. 60.6). Alternatively, gentle palpation can be used to identify the inferior alveolar nerve and artery before it enters the canal [121,132]. While maintaining the index finger on the mandibular foramen, the other hand is used to advance the needle. The needle can be introduced intraorally under the mucosa, or extraorally through the skin of the ventral aspect of the mandible (Fig. 60.7). For both techniques, the needle must be slowly advanced along the medial aspect of the mandible until the tip is located over the mandibular foramen and 0.1–0.5 mL of 0.5% bupivacaine is injected [121]. Hematoma formation at the mandibular foramen may be a frequent but usually minor complication that results from laceration of the local blood vessels [134]. Immediate treatment is applying manual pressure over the area to promote hemostasis. Another reported, but apparently infrequent, complication is self‐mutilation of the tongue during recovery from general anesthesia [133]. It has been suggested that this is due to diffusion of the local anesthetic solution onto the lingual nerve when attempting an inferior alveolar block using the caudal approach [121]. Deep tongue lacerations can occur very quickly and may bleed profusely; if severe, the patient may require immediate surgical repair under general anesthesia. Figure 60.5 Palpation of the mandibular foramen of a dog cadaver. The index finger of the nondominant hand is used to locate the foramen intraorally at a point between the mandibular third molar tooth and the angular process, on the medial aspect of the ramus. Figure 60.6 Location of the mandibular foramen, located on the medial and ventral portion of the ramus, between the third mandibular molar tooth and the angular process. Source: Dr. Raphaël Vézina Audette. Figure 60.7 Extraoral caudal approach to the inferior alveolar nerve of a cat cadaver. The needle is introduced through the skin of the ventral mandibular area and is slowly advanced along the medial aspect of the ramus toward the mandibular foramen, where the anesthetic agent will be deposited. The rostral approach consists of blocking the inferior alveolar nerve directly in the mandibular canal via the middle mental foramen (Fig. 60.8). The area anesthetized is variable and likely depends on how caudal within the canal the local anesthetic agent diffuses [136–138]. In general, the applicability of this technique may be limited to the rostral premolar, canine, and incisor teeth, and the rostral area of the lower lip. The middle mental foramen is palpated either intra‐ or extraorally using the non‐dominant hand. The foramen is typically located between the roots of the canine and the first premolar teeth. Intraorally, the area is covered by the labial frenulum; it may be necessary to displace it rostrally or caudally. Owing to its small size, the middle mental foramen is generally not palpable in small dogs and cats. Once the foramen has been identified, a needle is introduced at a 45° angle and advanced until it is engaged in the mental foramen and 0.1–0.3 mL of 0.5% bupivacaine is injected; this can be done intra‐ or extraorally. Significant needle manipulation may be necessary, and damage to the middle mental and inferior alveolar nerve and artery is possible. Figure 60.8 Insertion of a hypodermic needle into the mental foramen for blockade of the inferior alveolar nerve. Source: Dr. Raphaël Vézina Audette. Local dental infiltrative blocks are routinely used in human dentistry either as the sole means to achieve anesthesia for dental and oral procedures or to supplement regional anesthesia techniques [139]. Common local block techniques include intraosseous, intraligamentary (periodontal ligament), supraperiosteal, and intrapulpal injection [121,132]. Local anesthesia for dental procedures in dogs and cats has been reviewed in several publications [129,140]; however, no published clinical data are available on the use of these techniques in dogs and cats. Some local block techniques (e.g., intraligamentary) have been tested in dogs under experimental conditions [141], while others (e.g., supraperiosteal) are hypothetically considered ineffective owing to the thickness of the associated cortical bone [132]. Some local blocks require specialized equipment, representing an additional cost [141]. In addition, the duration of action of local dental blocks is shorter than that of regional blocks [132]. As a result, local dental blocks are infrequently used in dogs and cats. The orbit and surrounding tissues receive sensory innervation from the ophthalmic division of the trigeminal nerve (V1), which emerges through the orbital fissure and divides into the frontal, lacrimal, and nasociliary nerves. The lower eyelid receives sensory innervation from the zygomatic nerve, which branches from the maxillary division of the trigeminal nerve (V2) [125]. Motor innervation to the globe is provided by the oculomotor, trochlear, and abducens nerves. These nerves and their branches are located within the retrobulbar musculofascial cone, a tapering structure behind the globe consisting of the four rectus muscles and their associated connective tissues. The musculofascial cone tapers posteriorly to the annular tendon, which surrounds the optic nerve as it emerges from the skull through the optic foramen. Ophthalmic nerve blocks in this region are indicated for the prevention and relief of ophthalmic pain and to prevent the oculocardiac reflex during procedures involving the eye [142–144]. Preoperative administration of a retrobulbar nerve block is associated with a reduced incidence of the oculocardiac reflex during enucleation and superior analgesia when compared to an intraoperative splash block [144,145]. Several different techniques have been described to provide regional anesthesia of the eye and orbital tissues, which vary in their ability to produce akinesia of the globe. These techniques may be classified as extraconal or intraconal (outside or within the musculofascial cone), or retrobulbar or peribulbar (behind or around the eye). Retrobulbar blocks are more invasive and carry a risk of damaging the optic nerve; therefore, they are almost exclusively performed for enucleation, while less invasive peribulbar techniques or topical anesthesia are preferred for other types of ophthalmic procedures. Classically, a retrobulbar block is performed by inserting a curved needle at the junction of the middle and temporal third of the lower eyelid, directing the tip behind the globe until a popping sensation is appreciated and the eye rolls dorsally, indicating puncture into the musculofascial cone. Volume of injectate is dependent on the size of the patient, ranging from approximately 2–3 mL of 0.5% bupivacaine [143]. Alternatively, a supratemporal approach modeled on the technique used in horses has also been developed in dogs [146]. In this technique, the needle is inserted between the posterior aspect of the orbital ligament and the zygomatic process of the temporal bone and advanced ventromedially until the musculofascial cone is punctured. Deposition of local anesthetic within the musculofascial cone results in complete analgesia and akinesia of the globe. It is important to note that the optic nerve sheath contains cerebrospinal fluid (CSF) that is contiguous with intracranial CSF; accidental injection of local anesthetic within the optic nerve sheath can result in severe complications, such as total brainstem anesthesia [147–149]. As with all regional anesthetic techniques, cautious needle advancement and aspiration prior to injection of local anesthetic should be used. Peribulbar and extraconal techniques are less invasive than intraconal techniques and carry a lower risk of damage to the optic nerve. They rely on diffusion of local anesthetic from the extraconal space into the intraconal space and may produce akinesia less reliably than intraconal techniques. Using a blind technique, the needle is inserted percutaneously at the anterior dorsomedial and ventrolateral margins of the eye and advanced until the tip of the needle is level with the equator of the globe, being careful not to puncture the globe itself [150]. Ultrasound guidance may aid the operator in positioning the needle within the extraconal space. An ultrasound‐guided in‐plane peribulbar technique has been described in canine cadavers, in which the ultrasound transducer is positioned caudal to the orbital ligament, and the needle is introduced caudally, guiding the tip into the extraconal space. Peribulbar spread of injectate was successful in all attempts [151]. A similar posterior ultrasound‐guided approach with the transducer oriented transversely was less consistent in delivering injectate to the targeted structures [152]. Sub‐Tenon’s anesthesia refers to infiltration of local anesthetic within Tenon’s capsule, the fibrous sheath surrounding the eyeball. It requires a specialized sub‐Tenon’s cannula, several different types of which have been described with similar characteristics [153]. Sub‐Tenon’s anesthesia, described in depth elsewhere, has been reported to produce analgesia and akinesia for cataract surgery in dogs [154]. Nerve blocks of the auricular region may be indicated for pain management or surgeries involving the ear canal, tympanic bulla, or pinna. Sensory innervation of the external ear canal is provided by the auriculotemporal nerve and the auricular branches of the facial nerve, while the pinna is innervated by the caudal auricular and the greater occipital nerves [150]. The auriculotemporal nerve arises from the mandibular division of the trigeminal nerve (V3), running cranially to the external ear canal along the caudodorsal border of the masseter muscle and in close proximity to the facial nerve. The caudal auricular nerve is a branch of the facial nerve, and the greater occipital nerve is the largest branch of the second cervical spinal nerve. A blind approach to the great auricular nerve has been described in which the needle is inserted ventral to the transverse process of the atlas and advanced 0.3 cm ventromedially toward the jugular groove [155]. The auriculotemporal nerve can be blocked by inserting a needle perpendicular to the skin at the caudolateral border of the zygomatic arch and advancing toward the temporomandibular junction [155]. Alternatively, ultrasound guidance may be used to block the auriculotemporal and caudal auricular nerves; an ultrasound transducer is positioned in the coronal plane over the parotid salivary gland, perpendicular to the ramus of the mandible [150]. The auriculotemporal and caudal auricular nerves can be visualized between the external acoustic meatus and parotid salivary gland caudally and the condyloid process of the mandible cranially. The needle is introduced out‐of‐plane ventral to the transducer and advanced dorsomedially at an approximately 45° angle until the tip is positioned caudal to the condyloid process. The great auricular nerve is located superficially, cranial to the bifurcation of the jugular vein into the maxillary and linguofacial veins. It is easily visualized on ultrasound with the transducer oriented in the transverse plane at the level of the atlas. The great auricular nerve is a small, oval structure dorsal to the maxillary vein and mandibular salivary gland. Blocking the great auricular nerve provides analgesia to the skin overlying the caudal ramus of the mandible and on the dorsal surface of the pinna. Akinesia of the eyelid is possible due to the relationship between the auriculotemporal nerve and the facial nerve and should be treated with ophthalmic lubricants until palpebral function has returned. Ultrasound‐guided trigeminal nerve block has been reported in a dog undergoing extensive head surgery involving exenteration, caudal maxillectomy, and zygomatic arch resection [156]. Proximal blockade of the trigeminal nerve provides a broad area of desensitization in a single injection site, including areas innervated by the ophthalmic branch (V1) such as the orbit and nasal septum. However, the block is technically challenging compared to more peripheral techniques. The needle is introduced in‐plane to the ultrasound transducer immediately caudal to the orbital ligament and advanced ventrally toward the caudal pterygopalatine fossa, where the three branches of the trigeminal nerve and the maxillary artery can be visualized lateral to the frontal bone and overlying the sphenoid bone [150,156]. Doppler ultrasonography can aid in identification of the maxillary artery. The volume delivered is 0.1 mL/cm of the cranium length from the inion to the nasion. Dependent upon the region that requires blockade, there are a number of techniques that may be used to provide regional anesthesia to the thoracic walls, with or without visceral analgesia. In general, the innervation of the thorax and abdomen is provided by branches of spinal nerves. Dorsal branches of thoracic spinal nerves extend dorsally and divide into medial and lateral branches to innervate the epaxial muscles and the skin near the dorsal midline. The sensory innervation of the lateral and ventral thoracic wall is provided by the ventral branches of the thoracic spinal nerves via the intercostal nerves [125]. The skin of the thorax is innervated by dorsal, lateral, and ventral cutaneous branches of the spinal nerves [157]. The thoracic paravertebral space in dogs has been described as a wedge‐shaped area bordered ventrally by the parietal pleura, medially by the vertebral bodies, intervertebral discs, and foramina, laterally by the intercostal muscles, and dorsally incompletely by the costotransverse ligament [158]. Ventral branches of the spinal nerves travel distally via the dorsal compartment of the thoracic paravertebral space. Before the advent of technologies such as neurostimulation or ultrasonography to guide injections of local anesthetics, the standard approach to the thoracic paravertebral space consisted of a blind technique guided by anatomical landmarks [159]. Loss of resistance to injection performed with a loss of resistance syringe and the characteristic pop‐like feeling perceived by the operator once the endothoracic fascia is pierced by the tip of a Tuohy needle were long considered confirmatory for locating the thoracic paravertebral space. Portela et al. described a nerve stimulator‐guided method for injections into the thoracic paravertebral space; however, this technique was associated with a complication rate of 20%, which included instances of epidural spread and intrapleural migration of injectate from accidental puncture of the parietal pleura [158]. This technique, though imperfect, brought about a level of objectivity beyond that of blind injections where the subjective pop‐like feeling of the needle through the internal intercostal membrane guided the injection. The sonoanatomy of the canine thoracic paravertebral space in dog cadavers was subsequently described, along with a method to perform a thoracic paravertebral block with ultrasound guidance, further decreasing risks associated with the thoracic paravertebral block [160,161]. In addition to enabling the use of a lower volume of injectate, ultrasound guidance afforded a superior level of safety as direct visualization of the needle during injection prevented the puncture of the parietal pleura. Single injection points of diluted dye injectate in cadaveric studies resulted in staining beyond the thoracic paravertebral space despite correctly located injections, suggesting that local anesthetic spread outside of the immediate paravertebral space may play a role in the regional anesthesia observed with this technique. Based on inconsistent staining of multiple levels of spinal nerves under cadaveric conditions, complete thoracic anesthesia may require injection at multiple spaces. Another canine cadaveric study suggests the utility of this technique may extend beyond the thorax. Spinal nerves originating from T10 to L1 contribute sensory innervation to the cranial abdomen; caudal thoracic paravertebral spaces can also be injected to provide somatic and visceral analgesia at the level of the cranial abdomen [162]. Though this technique was associated with mediastinal contamination in 80% of injections, no other complications were noted. Clinically, a single injection at the level of the caudal thoracic paravertebral space has been shown to provide adequate analgesia in radical mastectomy [163], lateral thoracotomy [164], and adrenalectomy via midline laparotomy [165]. The thoracic paravertebral block was also described in other animal species (Vulpes vulpes) [166]. Together, the evidence suggests thoracic paravertebral blocks are useful for the provision of perioperative analgesia in thoracic and cranial abdominal surgeries. It is important to note that ultrasound‐guided thoracic paravertebral block is a technically challenging technique and is recommended for more advanced practitioners of ultrasound‐guided regional anesthesia.
60
Canine and Feline Local Anesthetic and Analgesic Techniques
Introduction
Classification of locoregional anesthesia
Topical anesthesia
Local infiltration
Intravenous regional anesthesia
Neuraxial anesthesia
Peripheral nerve blocks
Physiology of nerve blockade
Anatomy of the peripheral nerve
Local anesthetic pharmacology
Differential blockade
Local anesthetic additives
Opioids
Adrenergic agonists
Patient preparation
Equipment
Needles
Catheters
Injection pressure monitors
Peripheral nerve stimulators
Ultrasound
Blocks of the head
Maxillary techniques
Anatomy
Indications
Technique
Mandibular techniques
Anatomy
Indications
Technique
Local tissue dental blocks
Anesthesia for ophthalmic procedures
Anatomy
Techniques
Auricular techniques
Anatomy
Techniques
Trigeminal nerve block
Blocks of the thorax and abdomen
Thoracic paravertebral block
Intercostal nerve block
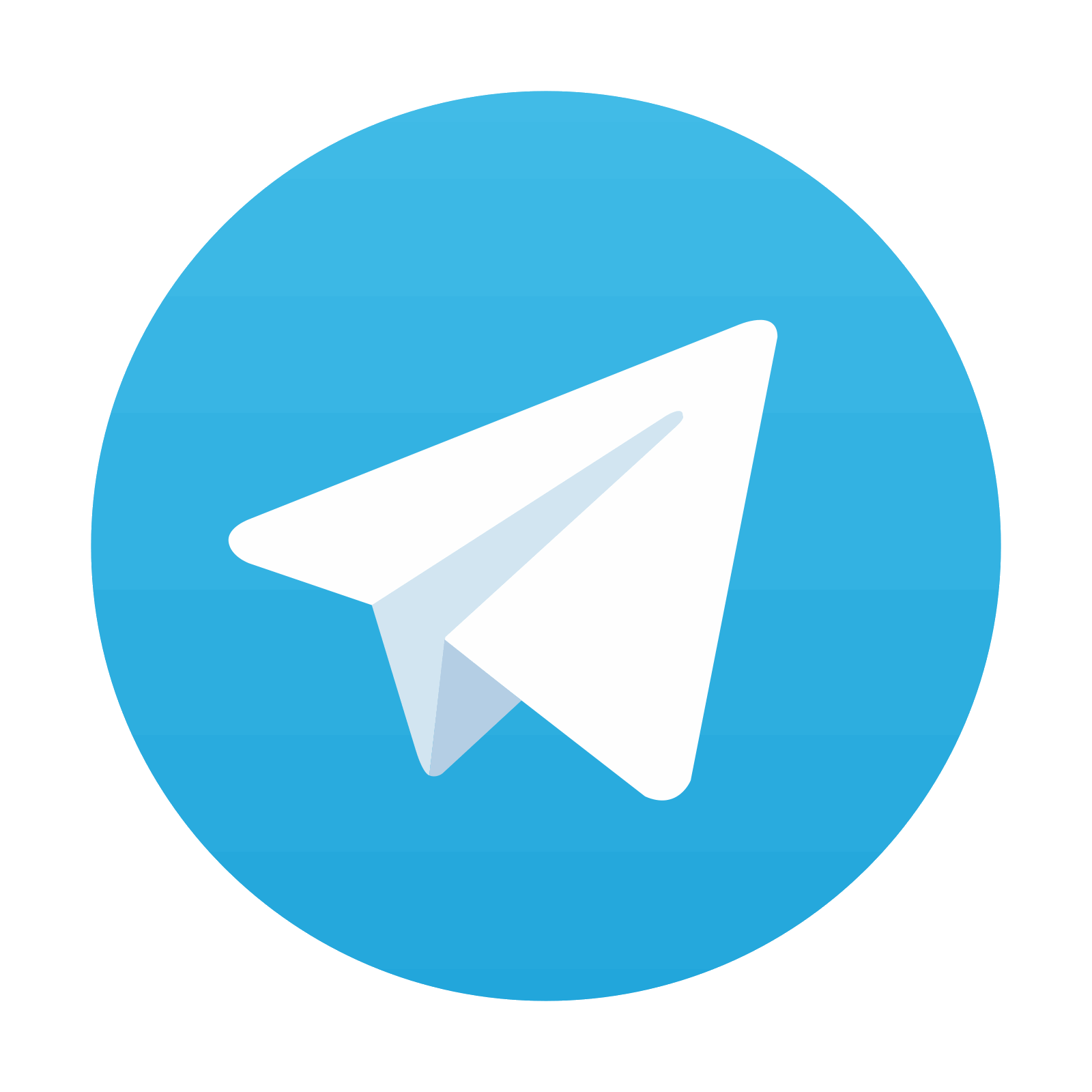
Stay updated, free articles. Join our Telegram channel
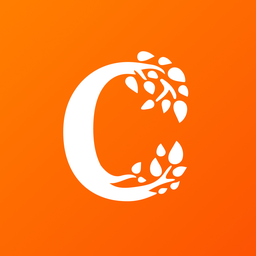
Full access? Get Clinical Tree
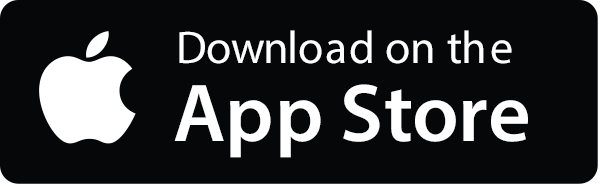
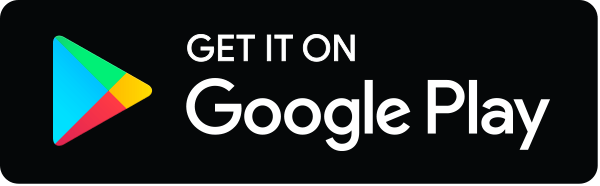