7 Bovine Parainfluenza Virus 3 Virginia-Maryland Regional College of Veterinary Bovine parainfluenza virus 3 (BPIV-3) was first isolated in the USA from the nasal discharge of calves showing clinical signs of shipping fever, including rapid onset of fever, nasal discharge, coughing and other respiratory signs, lacrimation, conjunctivitis and lack of appetite (Andrewes et al., 1959; Reisinger et al., 1959). Since then, the virus has been reported globally from infected and clinically normal cattle. It is generally accepted that co-infection of BPIV-3 along with other viruses and Mannheimia/Pasturella species could result in a clinical disease known as shipping fever, now known as undifferentiated fever/bovine respiratory disease complex (UF/BRDC) (Edwards, 2010). A variety of factors, such as environmental temperature, transportation, hygiene, stocking density, co-mingling and host immune status, can contribute to increased susceptibility to secondary bacterial infection and severity of clinical disease. UF/BRDC is the most important cause of economic losses for the stocker and feedlot production systems (Gagea et al., 2006; Snowder et al., 2006) in many countries where the climate provides an additional stress. Poor growth performance, therapeutic costs, mortality and reduced carcass value contribute to these losses, estimated to be about US$1 billion per year (Griffin, 1997). Research on the viral aetiology of UF/BRDC has largely focused on bovine herpes virus-1 (BHV-1), bovine viral diarrhoea virus (BVDV) and bovine respiratory syncytial virus (BRSV). Few publications have considered HPIV-3 on its own, with most reports including BPIV-3 as one among multiple viruses and bacteria described (Fulton, 2009). The role of BPIV-3 in UF/BRDC is still elusive because of its endemicity, concurrent infections with other viruses, bacteria and Mycoplasma, and the continued use of modified live virus vaccines. BPIV-3 has a worldwide distribution. BPIV-3 infections were found in a variety of species including cattle (Andrewes et al., 1959; Reisinger et al., 1959), humans, sheep (Lyon et al., 1997), goats (Yener et al., 2005), bison (Zarnke and Erickson, 1990), water buffalo (Maidana et al., 2012), guinea pigs (Ohsawa et al., 1998), black and white rhinoceros (Fischer-Tenhagen et al., 2000), moose (Thorsen and Henderson, 1971), bighorn sheep (Parks et al., 1972) and camels (Eisa et al., 1979). Cross-species infection has been reported in humans, sheep and non-human primates with bovine PIV-3 (Stevenson and Hore, 1970). Unlike HPIV-3, human-to-human transmission of bPIV-3 is poor. Between susceptible animals, the virus is transmitted by aerosol and fomites contaminated with nasal discharges. The infection is generally subclinical in calves, kids and lambs, but may manifest as severe respiratory disease and pneumonia. The role of BPIV-3 in shipping fever is fiercely debated. However, poor hygiene, crowding, transport, harsh climatic conditions and other stressors typically result in UF/BRDC where BPIV-3 may or may not be involved. BPIV-3 is an enveloped, non-segmented, negative-sense, single-stranded RNA virus classified in the genus Respirovirus in the Paramyxoviridae family, order Mononegavirales (King et al., 2012). The other members of the genus Respirovirus include human parainfluenza viruses 1 and 3 (HPIV1 and HPIV3), and Sendai virus (Karron and Collins, 2007), Interestingly, three of the five genera of the subfamily Paramyxovirinae include parainfluenza viruses (PIV), which share physical and morphologic properties (Karron and Collins, 2007). Genus Rubulavirus contains HPIV2, SV5, SV41 and mumps viruses, while genus Avulavirus contains avian paramyxoviruses (APMV) serotypes 1 through 9, of which Newcastle disease virus is the prototype APMV-1 (Karron and Collins, 2007). The virions of PIV are morphologically indistinguishable and also share distinct biophysical characteristics. All of them possess haemagglutinating and neuraminidase activities (Karron and Collins, 2007). There are three geno-types of BPIV-3 designated A, B and C. Previously, the complete genome analyses of the representative BPIV-3 isolates from Australia and North America indicated that BPIV-3 falls into two distinct genotypes, BPIV-3 genotype A (BPIV-3a) and BPIV-3 genotype B (BPIV-3b) (Horwood et al., 2008). Recently, four isolates, which were only detected in China, were proposed as genotype C, (Zhu et al., 2011). The family Paramyxoviridae has a typical negative-stranded RNA virus structural pattern. The enveloped virus particle contains a left-handed, helically symmetrical ribonucleoprotein core, called the nucleocapsid, which consists of a non-segmented single-negative-stranded viral RNA genome surrounded by nucleocapsid proteins and associated with phosphoprotein and a large polymerase protein (Lamb and Parks, 2007). The nucleocapsid of Paramyxovirinae, 18 nm in diameter, 1 μm in length and a pitch of 5.5 nm in diameter, is enclosed by an outer lipoprotein envelope (Lamb and Parks, 2007). The helical nucleocapsid of paramyxoviruses presents a ‘herring bone’ morphology when viewed under an electron microscope. Pneumovirinae subfamily can be differentiated from Paramyxovirinae subfamily morphologically because they have narrower nucleocapsids (Lamb and Parks, 2007). The virions of Paramyxoviridae are pleomorphic in shape, being spherical or filamentous. In general, spherical virions are 150–350 nm in diameter (Lamb and Parks, 2007). Like other PIV, BPIV-3 virions are pleomorphic 150–300 nm in diameter. BPIV-3 has a buoyant density of 1.197. The RNA of BPIV-3, like that of Sendai virus, is a single continuous chain that lacks polyadenylic acid sequences and tends to self-anneal to a marked extent. It has a sedimentation coefficient of 42S and a molecular weight of 4.5 × 106 (Shibuta et al., 1979). The genome length of family Paramyxoviridae ranges from 15,000 to 19,000 nucleotides (nt), encoding 6–10 tandem linked genes in the order 3′-N-P-M-F-HN-L-5, where N, P, M, F, HN and L indicate the genes for the nucleocapsid protein, phosphoprotein, matrix, fusion, attachment and large polymerase proteins (Karron and Collins, 2007). The phosphoproteins encode additional accessory proteins by a process called ‘RNA editing’. The genomic map of a typical parainfluenza-virus is shown (Fig. 7.1). Interestingly, the genome length of most members of Paramyxoviridae is divisible by six, known as the ‘rule of six’ (Calain and Roux, 1993; Kolakofsky et al., 1998, 2005), i.e. their genomes must be of polyhexameric length (6n+0) to replicate efficiently. Each nucleo-protein subunit associates with 6 nt and that makes the RNA chain length an even multiple of six when completely encapsidated. There is a ~50 nt 3′ extracistronic leader region and a 50–171 nt 5′ extracistronic trailer region (or [–] leader) at the two ends of the genome. The leader and trailer are considered to be cis-acting signals and are essential for transcription and replication. Between the gene boundaries, there are intergenic regions, which are strictly conserved trinucleotides in Respirovirus, Henipavirus and Morbillivirus, but quite variable in length for the Rubulavirus and Pneumovirinae, ranging from 1 to 56 nt. The newly discovered paramyxoviruses, such as Tioman virus, have even longer intergenic regions, which may be up to 70 nt in length. Similar to other Respiroviruses, BPIV-3 consists of six non-overlapping genes, which are predicted to encode nine proteins with conserved and complementary 3′ leader and 5′ trailer regions, conserved gene starts, gene stops and trinucleotide intergenic sequences. The genome of BPIV-3 follows the ‘rule of six’. Envelope glycoprotein complexes of Paramyxoviridae are stalk-like spikes on the surface of virions. Fusion protein (F) and an attachment protein, called haemagglutininneuraminidase (HN) or haemagglutinin (H) or glycoprotein (G) depending on the virus genera (Lamb and Parks, 2007), are the major components of the spikes. The F and HN/H/G proteins may be in the form of trimers (F) and tetramers (attachment protein), respectively. The envelope glycoproteins are responsible for mediating virus attachment and penetration during infection. The internal helical nucleocapsid core contains the RNA genome and nucleocapsid (N), phospho- (P) and large (L) polymerase proteins. Another structural protein, matrix (M) protein, is located between the envelope and the core, and is important in virion architecture and the infection process. Accessory proteins are mostly generated from overlapping open reading frames within P gene transcriptional units by a process called RNA editing or by alternative translation initiation. These accessory proteins are important in viral morphogenesis, RNA synthesis and pathogenesis (Lamb and Parkes, 2007). Fig. 7.1. Schematic of genome organization of BPIV-3 (not to scale). The BPIV-3 genome is depicted as a rectangle with vertical bars denoting the intergenic regions. The 3′ leader and the 5′ trailer regions are shown. The gene-end and gene-start sequences flanking each of the intergenic sequences are depicted. The P gene also encodes V/D/W and C proteins. The genome termini carry complementary sequences. The N protein of BPIV-3 is one of the most abundant proteins and is present as the first transcribed gene, adjacent to the leader sequence at the 3′ end of viral genomic RNA. The N protein is composed of 489–553 amino acids (aa) with the predicted molecular weight of 53–58 kDa. The N-terminal region of the N protein of paramyxoviruses is involved with encapsidation of viral RNA, and coats the full length of viral [–] sense genomic and [+] sense antigenomic RNAs to form the helical nucleocapsid. This helical structure serves several functions, including protection from nuclease digestion, alignment of distal RNA segments to create a functional 3′-end promoter and providing interaction with P and L proteins during transcription and replication, and association with M protein during virus assembly (Lamb and Parks, 2007). Sendai virus N binds approximately six consecutive nt and 13 subunits constitute each turn of the nucleocapsid helix (Egelman et al., 1989). But slight differences in the number of N subunits per helix turn and pitch of the helix have been reported among paramyxoviruses (Bhella et al., 2002). BPIV-3 N is expected to bind the genome and antigenome similar to that of SeV. N protein exists in at least two forms in infected cells. The first form is tightly associated with RNA in a nucleocapsid structure (Karron and Collins, 2007). The second unassembled soluble form termed N0 has been shown to be associated with P protein for a number of paramyxoviruses, including SeV (Horikami et al., 1992), SV5 (Precious et al., 1995), hPIV 3 (Zhao and Banerjee, 1995) and others (Karron and Collins, 2007). Paramyxovirinae P gene encoding multiple viral proteins can be considered to be a remarkable example of exploiting the coding capacity of viruses. SeV P gene can direct the expression of at least seven polypeptides, including P, V, W, C′, C, Y1 and Y2. Other paramyxoviruses may express fewer proteins from the P gene, but this capability is always seen. Generally, two mechanisms involve production of multiple mRNA species from the P gene. One is known as alternative initiation, by which the family of C proteins is produced from an alternative translation initiation codon. The second mechanism involves pseudotemplated insertion of multiple G residues known as ‘RNA editing’ at a specific position, termed the mRNA editing site, to produce mRNAs of P, V proteins and virus-specific proteins variously called W/D/I proteins, depending on the virus genus. P, V, W/D/I are a set of proteins that are amino co-terminal and the mRNAs are only different by inserted G nucleotides that shift the translation reading frame after the insertion site (Lamb and Parkes, 2007). In BPIV-3, the P protein is encoded as the translation product from the unedited mRNA (+0G), the V protein by a transcript containing a single G residue at the editing site (+1G) and the D protein by the insertion of two G nucleotides (+2G), but the C protein is produced by alternate translation initiation (Karron and Collins, 2007). The P protein of PIV is essential for viral RNA synthesis and it interacts with multiple partners during the viral growth cycle (Karron and Collins, 2007). The paramyxovirus P protein is generally 400–600-aa long. It is heavily phosphorylated at serine and threonine residues predominantly within the N-terminal region (Lamb and Parks, 2007). Distinct modular carboxy and N-terminal domains within the P protein play essential roles as a polymerase co-factor and in nascent chain assembly. The C-terminal polymerase co-factor module is conserved in the predicted secondary structure for all Paramyxovirinae members. It contains domains for P–P multi-merization, for L protein interactions and for N–RNA template binding (Horikami et al., 1992). The N-terminal region is believed to act as a chaperone to facilitate interactions with unassembled N in order to prevent N aggregation and to prevent uncontrolled encapsidation of non-viral RNA (Curran et al., 1995). A defined N-terminal region of P up to residue 78 is required for genome replication and assembly as deletion of residues 78–324 still makes the protein active (Curran et al., 1985; Karron and Collins, 2007). The P protein functions as a multimer and Sev P protein is a tetramer (Tarbouriech et al., 2000a, 2000b). Corresponding domain structure and functional studies with BPIV-3 P proteins are lacking but a comparative domain structure with P-gene RNA editing strategy is shown (Fig. 7.2). Fig. 7.2. Schematic and structure-based sequence alignment of BPIV-3 P/V/D and C proteins. The P, V and D proteins: rectangular boxes with a common NH2 terminal domain but unique C-terminal domains that result from addition of 1 (V) or 2 non-templated G (D) residues. Vertical line: the RNA editing site from where the point of sequence divergence starts. The P gene mRNA editing site of various members of the Paramyxovirinae is compared. The free N0 binding region of P protein is shown at the amino-terminal. The oligomerization domain of the P protein at the C-terminal is shown as 4′mer. The L–P interaction domain is shown as L. The extreme C-terminal domain of the P protein is required for the interaction of the P protein to the N-RNA template. The C protein is expressed from the P mRNA using a +1 reading frame with respect to the P open reading frame. BPIV-3: Bovine parainfluenzavirus 3, ASPV: Atlantic salmon paramyxovirus, J-V: J virus, BeV: Beilong virus, FDLV: Fer-de-Lance virus, TPMV: Tupaia paramyxovirus. The type I interferon (IFN) response is one of the most important antiviral responses and has two general phases: a primary transcriptional phase of induction of IFN synthesis, and a secondary transcriptional phase through the type I IFN signaling pathway (Borden et al., 2007). Paramyxovirus accessory proteins can counteract the host-cell IFN pathways at both levels. The paramyxovirus V protein cysteine-rich domain can limit dsRNA-induced activation of the IFN-β promoter by direct interaction with melanoma differentiation-associated gene 5 (MDA5) (Andrejeva et al., 2004; Komatsu et al., 2004). The expression of C proteins in SeV and W proteins in NiV can also reduce IFN-β promoter activation in response to dsRNA (Komatsu et al., 2004). IFN signaling is initiated by binding of secreted IFN and its cognate receptor on the cell surface leading to phosphorylation of latent transcription factors, STAT1 and STAT2, which are signal transducers and activators of transcription (Karron and Collins, 2007; Lamb and Parks, 2007). The V protein of some paramyxoviruses such as PIV5, MuV, SV41, hPIV2 and NDV can target one of the STATs for degradation to block the IFN signaling (Goodbourn and Randall, 2009), while other V proteins, like those of henipaviruses and measles virus, can form a V and STAT protein binding complex and prevent its translocation to the nucleus (Palosaari et al., 2003; Rodriguez et al., 2003; Shaw et al., 2004). The C protein of respiro- and morbilliviruses are involved in blocking IFN signaling by a different mechanism (Garcin et al., 2001, 2002; Gotoh et al., 2003). SeV and HPIV3 can alter STAT phosphorylation patterns (Gotoh et al., 2003), interact with STAT1 (Garcin et al., 2001; Takeuchi et al., 2001), and induce ubiquitination and degradation of STAT1 in some mouse cells (Garcin et al., 2002). The V protein is a 25–30 kDa polypeptide that is amino co-terminal with P protein but with a variable C-terminal domain. The C-terminal V-specific domain is highly conserved among paramyxoviruses with invariantly spaced histidine and cysteine residues forming a domain (cys-rich domain) that binds two zinc molecules per V protein (Liston and Briedis, 1994; Paterson et al., 1995; Fukuhara et al., 2002). The V protein plays important roles in virus replication (Curran et al., 1991; Baron and Barrett, 2000), functions as a negative regulator to inhibit RNA synthesis (Delenda et al., 1997; Durbin et al., 1999; Baron and Barrett, 2000) and inhibits host-cell antiviral response by interacting with cellular proteins (Lin et al., 1998; Andrejeva et al., 2002). The V protein of BPIV-3 has been shown to target melanoma differentiation antigen-5 (MDA5), but not RIG-I for blocking the IFN-β activation signal (Komatsu et al., 2007). Interestingly, it does not suppress IFN-β production induced by TRIF, TBK1 and IKKi molecules downstream of MDA5 and RIG-I (Komatsu et al., 2007). The W and D proteins are expressed by inserting two G residues at the P gene mRNA editing site in respiro-, morbilli- and henipaviruses. The W protein in SeV is found to interact with unassembled β, suggesting an inhibitory role in viral RNA synthesis (Horikami et al., 1996). In PIV3, insertion of two G residues at the editing site produces a protein called D protein (Galinski et al., 1992). In Rubulaviruses, insertion of one or four G residues during RNA editing produces I protein (Thomas et al., 1988; Paterson and Lamb, 1990). Despite high sequence homology (~59%) between the cysteine-rich carboxy terminus of V proteins of BPIV-3 and SeV, there is high sequence diversity in the carboxy terminal regions between the D protein of BPIV-3 and W protein of SeV (Nagai, 1999). The D protein consists of the P–V amino co-terminal region (241 aa) plus a long unique carboxy terminal region (Fig. 7.2), while the W open reading frame is terminated by a stop codon immediately after the editing site. The role of W/D/I in the viral growth cycle has not been understood (Lamb and Parks, 2007). The D protein of BPIV-3 does not block type I IFN in infected cells (Komatsu et al., 2007). The exact function of the D protein is unknown. I protein is not produced by BPIV-3. It is only seen in members of Rubulavirus due to the insertion of either one or four G residues to the P mRNA during RNA editing. C proteins are generated using alternative translation initiation codons (+1 reading frame with respect to the P open reading frame) in Respiro-, Henipa-, Morbillivirus (Lamb and Parks, 2007). In SeV, C′, C, Y1 and Y2 comprise a nested set with a shared C-terminal. There is less degree of sequence homology in the C protein between SeV and BPIV-3, compared to the high degree of homology (~70%) between SeV and HPIV1 (Nagai, 1999). The C proteins are small basic polypeptides that may be involved in the viral growth cycle, control of viral RNA synthesis (Lamb and Parks, 2007), counteracting host-cell antiviral pathways (Komatsu et al., 2004) and facilitating the release of virus from infected cells (Garcin et al., 1997; Kato et al., 2001). The C protein of BPIV-3 has been shown to suppress double-stranded RNA-stimulated IFN-β production to some degree, possibly through MDA5 or RIG-I mediated signaling because its also inhibits IFN-β production stimulated, not only by TRIF, but also by TBK1 or IKKi downstream of both MDA5 and RIG-I (Komatsu et al., 2007). Therefore, the available evidence suggests the involvement of both V and C proteins in inhibition of type I IFN. However, it is presently unknown whether the BPIV-3 V or C proteins block IFN-β by targeting members of the JAK-STAT pathway. The M protein is the most abundant protein in the virion, comprising 341–375 residues with a molecular weight of 38.5–41.5 kDa. The net charge at neutral pH is +14 to 17, making it a basic protein. Although there is no transmembrane domain that has sufficient length to span a lipid bilayer, M protein is somewhat hydrophobic and peripherally associated with membranes (Lamb and Parks, 2007). The M protein is considered to be the central organizer of viral morphogenesis, interacting with the cytoplasmic tails of integral membrane protein, F and HN, the lipid bilayer and the nucleocapsids (Blumberg et al., 1984; Sanderson et al., 1993; Stricker et al., 1994; Cathomen et al., 1998; Schmitt et al., 1999). The so-called ‘late domains’ have been identified in several paramyxoviruses that interact with cellular protein sorting machinery (Karron and Collins, 2007). The presence or absence of a phosphorylation site in the M protein did not change the phenotype of SeV virions (Sakaguchi et al., 1997). The L protein, located at the most promoter distal part in the genome, is the least abundant protein in virus particles. A paramyxovirus particle contains only ~50 copies of L (Lamb et al., 1976). It is an essential subunit of paramyxovirus RNA-dependent RNA polymerase (RdRp). The L gene consists of ~2200 aa with a molecular weight of 220–250 kDa. The L protein possesses all the enzymatic activities needed for polymerization, 5′-end capping, methylation and 3′-end polyadenylation of mRNA (Grdzelishvili et al., 2005; Karron and Collins, 2007; Lamb and Parks, 2007). The L protein associates with P protein to form the active viral polymerase and the polymerase complex can recognize the helical N-RNA template (Hamaguchi et al., 1983; Poch et al., 1990). The L protein of BPIV-3 possesses a conserved domain structure, template recognition, ATP-binding and polymerization sites similar to those of other Respiroviruses. Attachment protein is another integral membrane glycoprotein in Paramyxoviridae involved in the cell attachment process, which is also an integral membrane protein (Lamb and Parks, 2007). The attachment protein of Respirovirus, Avulavirus and Rubulavirus binds to cellular sialic acid-containing receptors and are capable of agglutinating erythrocytes. These attachment proteins also have neuraminidase activity that cleaves sialic acid from the progeny virus particles to prevent viral self-aggregation (Scheid and Choppin, 1974) and enable release of virions from the cell membrane. Thus the protein has been designated HN. HN is a type II membrane protein composed of 565–582 residues that span the membrane once. It contains an N-terminal cytoplasmic tail, a single N-terminal transmembrane domain and a membrane-proximal stalk domain that supports a C-terminal globular head domain. The globular head domain contains both receptor binding and enzymatic activity (Hiebert et al., 1985). The attachment protein of BPIV-3 is 1888-nt long with a single open reading frame that could encode a 572-aa residue protein. HN presents on the surface of virions and infects cells as a tetramer consisting of pairs of homodimers (Thompson et al., 1988). BPIV-3 strains SK217 and SF/Ka were shown to differ in virulence (Shibuta et al., 1981; Breker-Klassen et al., 1996). This virulence difference had been associated with a change in amino acid 193 in HN protein, which had a dramatic effect on syncytium-inducing activity, neuraminidase activity and haemagglutinating activity (Breker-Klassen et al., 1996). For all paramyxoviruses, co-expression of F and HN (H or G) is required for fusion or the co-expression of HN makes fusion more efficient (Karron and Collins, 2007). An interaction of F with homotypic and not heterotypic HN has been shown to promote fusion (Horvath et al., 1992; Sergel et al., 1993). The F and HN glycoprotein genes of BPIV-3 were shown to be the major determinants of host-range restriction in rhesus monkeys by generating HPIV3 chimeras with BPIV-3 envelope glycoproteins (Schmidt et al., 2000), but a subsequent study confirmed that the host-range restriction of replication of BPIV-3 is polygenic (Skiadopoulos et al., 2003). The immunity to PIV appears to be short lived (Glezen et al., 1984) and reinfections with the same types may occur within short time intervals (Chanock et al., 1961; Muchmore et al., 1981). This is stated to be the result of poor immunological response in the respiratory tract rather than of antigenic instability (Yanagihara and McIntosh, 1980). The efficacy of post-infection immunity and difference in the virulence of isolates/strains may also depend on antigenic differences (Shibuta et al., 1982, 1985). Plaque variants of BPIV-3 strain YN show differences in virulence in mice (Shibuta et al., 1985). The SC-YN variant and 910N strain induce a non-lethal hydroencephalus when inoculated intracerebrally in newborn mice, but the M-YN variant causes a lethal brain disease (Shibuta et al., 1982). There are very few studies that examined the antigenic characteristics of BPIV-3 employing monoclonal antibodies (MAbs). Human and bovine PIV3 share neutralizing epitopes but show distinct antigenic properties (Muchmore et al., 1981; Glezen et al., 1984; Coelingh et al., 1986). In an analysis with 52 MAbs against N, M, F, HN proteins of PIV3, it was established that the bovine and human PIV3 strains were antigenically quite different (Rydbeck et al., 1987). Six epitope groups in the HN were described (Shibuta et al., 1986; Rydbeck et al., 1987). Epitope III specific HN-MAbs showed no heterotypic reaction to human PIV3 (Klippmark et al., 1990). Fifty-three MAbs to the human C243 strain were directed against six, four, nine and seven epitopes of the HN, F, N and M proteins, respectively. Seven MAbs to the bovine strain 910N were directed against three epitopes of the HN and three epitopes of the F protein. The M protein of BPIV-3 is antigenically conserved and the F protein is equally homogeneous. There appears to be more variation in the N protein and limited variations of HN antigenic epitopes amongst BPIV-3 (Klippmark et al., 1990). Overall, the available evidence suggests that there is only limited antigenic variation among BPIV-3 strains recovered at different geographic regions at various times. The PIV ribonucleoprotein (RNP) or transcriptase complex, consisting of the negative sense genomic RNA encapsidated with N protein, and the associated RdRp hetero-complex, the L and P proteins, is released into the cytoplasm following fusion of the cell membrane with the viral envelope (Chattopadhyay et al., 2011). The N-RNA is used by the RdRp as a template for transcription and replication in the presence of P protein co-factor activity. It is generally believed that all negative sense, non-segmented RNA viruses (NSV) including PIV follow a common transcription and replication strategy (Banerjee et al., 1977; Karron and Collins, 2007; Lamb and Parks, 2007; Chattopadhyay et al., 2011). The standard scheme for transcription and replication of NSV at present is as follows: the RdRp heterocomplex enters the viral genome at the 3′ end and initiates transcription to synthesize the leader RNA and viral mRNAs by a start–stop mechanism (Emerson, 1982). The RdRp also is responsible for capping, cap methylation and addition of polyA tail at the 3′ end of the nascent mRNA (Ogino and Banerjee, 2007). Re-initiation of transcription at the gene-start site of NSV is imperfect, which leads to a gradient of mRNA abundance that decreases down the viral genome, depending on the distance from the 3′ end of the viral genome. This property is known as ‘polarity of transcription’ (Fig. 7.3). Several host proteins such as cellular actin, tubulin (Moyer et al., 1986; Ogino et al., 2003), β catenin (Bose and Banerjee, 2004), and cellular glycolytic enzymes, phosphoglycerate kinase and enolase (Ogino et al., 2001), and specific phosphorylated forms of glyceraldehyde-3-phosphate dehydrogenase (GAPDH) (Choudhary et al., 2000) have been shown to be involved in the transcription and replication of PIV. The switch from transcription to replication is unclear. However, when a sufficient amount of N protein accumulates in the cell, it complexes with the P protein to form a soluble N–P complex, which is used for the replication of the progeny genome RNA. Replication commences when the RdRp ignores the transcription stop signals at each gene junction and a full-length positive sense antigenome is synthesized. The anti-genome is also fully encapsidated by the nucleocapsid proteins and the anti-genome then serves as a template for the synthesis of the negative sense genome (Fig. 7.3). It is surmised that the antigenomic promoter is stronger than the genomic promoter, resulting in more copies of the genome sense RNA, which is encapsidated, packaged and released as virions. There are excellent recent reviews on the complex mechanisms of transcription and replication in NSV (Karron and Collins, 2007; Lamb and Parks, 2007; Banerjee, 2008; Whelan, 2008; Chattopadhyay et al., 2011).
Medicine, Blacksburg, USA
7.1 History and Importance
7.2 Epizootiology
7.3 Infectious Agent
7.3.1 Classification
7.3.2 Virion structure
7.3.3 Genome organization
7.4 Viral Proteins
7.4.1 Nucleocapsid protein
7.4.2 The P gene and its encoded proteins
7.4.3 Viral accessory proteins and their interactions with the host
V protein
W/D/I protein
C protein
7.4.4 Matrix protein
7.4.5 L protein
7.5 Viral Glycoproteins
7.5.1 Fusion protein
7.5.2 Attachment protein
7.6 Antigenic Analysis
7.7 Transcription/Replication
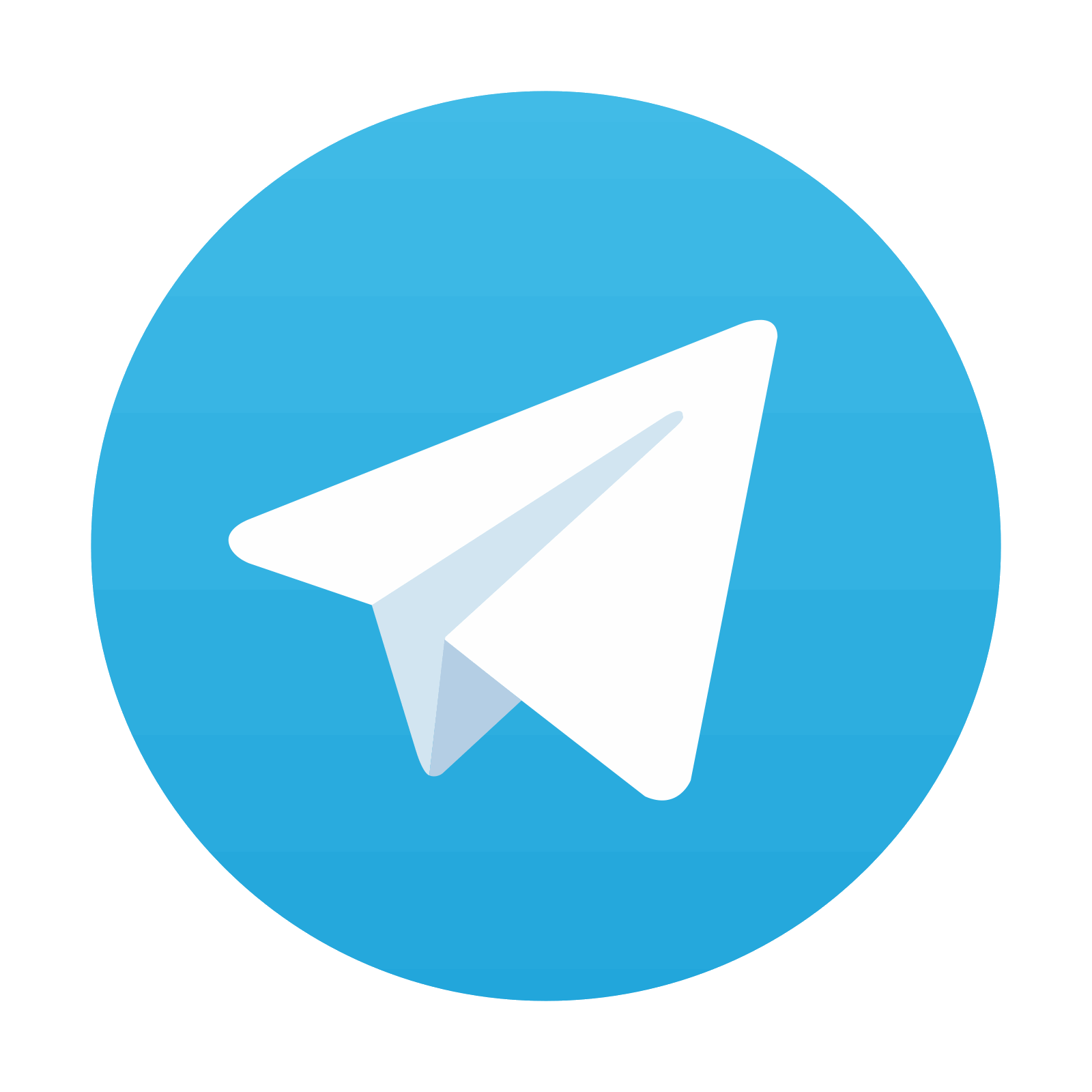
Stay updated, free articles. Join our Telegram channel
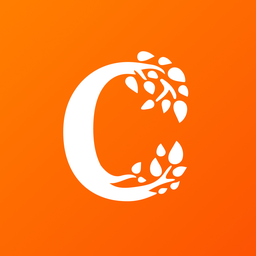
Full access? Get Clinical Tree
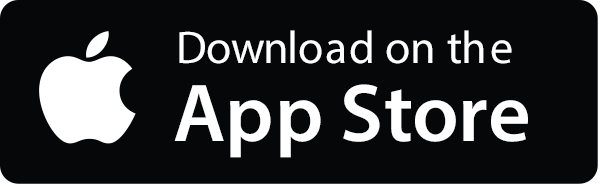
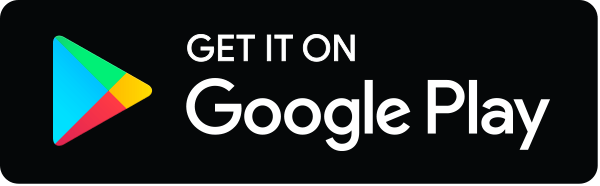