Odette O SAGE Veterinary Centers, a member of Ethos Animal Health, Dublin, California, USA Blood pressure (BP) is a vital sign that is relatively easy to measure in most veterinary patients. Perfusion of organs and tissues is vital in delivering oxygen and nutrients as well as removing metabolic byproducts. While the measurement of BP does not directly measure perfusion, pressure is required to bring blood to tissues and vital organs including the heart, brain, and kidneys. Trauma and surgical interventions may cause hemodynamic shifts, while the administration of sedation and general anesthesia results in alterations in cardiac function and vasomotor tone, thereby affecting BP (Box 12.1). BP monitoring should be a part of the care and management of all patients undergoing sedation and anesthesia, as outlined in both the American Animal Hospital Association and American College of Veterinary Anesthesia and Analgesia monitoring guidelines [1,2]. BP measurement is traditionally expressed as a series of three values: systolic arterial pressure (SAP), diastolic arterial pressure (DAP), and mean arterial pressure (MAP), each with their own importance in patient assessment. SAP is the pressure exerted by blood against the arterial walls during systole of the cardiac cycle. DAP represents the amount of pressure that blood exerts on the arterial walls during cardiac relaxation (diastole). MAP is of great importance in anesthetized patients, as it determines perfusion pressure in the tissues and is directly influenced by cardiac output (CO) and systemic vascular resistance (SVR). Conversely, pulse pressure (PP) is simply the difference between SAP and DAP and does not indicate appropriate tissue perfusion. Since most sedative and anesthetic agents affect vasomotor tone, digital pulse palpation should not be used as a substitute for accurate measurement of BP in anesthetized patients. MAP represents the area under the arterial pressure/time curve divided by the cardiac cycle duration. Modern invasive blood pressure measurement systems accurately calculate MAP based on analysis of the arterial waveform, while most non‐invasive oscillometric systems measure MAP as the greatest cuff pressure amplitude variation. In addition, MAP may be roughly estimated by the following formula, though this equation has a number of limitations: Factors that influence MAP include CO and SVR: CO is a product of heart rate (HR) and stroke volume (SV): A more in‐depth discussion of CO may be found in elsewhere in this text (Chapter 33). Briefly, HR is influenced by chronotropy, lusitropy, and dromotropy, whereas SV is influenced by volume (inotropy, preload, and total blood volume) in addition to afterload. Chronotropy defines the physiologic timing of HR. Lusitropy is the period of cardiac relaxation that occurs during diastole when cardiac myocytes have a decreased level of cytosolic calcium, as opposed to inotropy when increased calcium uptake causes positive contractile effects in the cardiac myocytes. Dromotropy is the actual conduction of an impulse, usually through the AV node, which in turn influences HR. SVR, also known as “total peripheral resistance,” is influenced by vascular size (1/∞) and blood viscosity and is defined by the amount of force that the vasculature system exerts on circulating blood, excluding pulmonary circulation. SVR influences BP via three factors: vessel length, vessel diameter, and the viscosity of the blood [3]. Alterations in vessel diameter have an enormous effect on vessel flow, as defined by the Hagen–Poiseuille equation, whereby a reduction of vessel diameter by 1/2 decreases flow to only 1/16th of the original flow: where ∆P is the pressure difference between the two ends of the vessel, Q is the volumetric flow rate, L is the length of vessel, η is the dynamic viscosity, r4 is the vessel radius to the fourth power, and 8/π is a constant of proportionality. A consensus statement by the American College of Veterinary Internal Medicine (ACVIM) details guidelines for the identification, evaluation, and management of systemic hypertension in dogs and cats [4]. This statement cites difficulty in determining a single value or range acceptable for all cats and dogs and that normal ranges of BP will vary by species and patient factors, including temperament, age, breed, body condition, and sex. Measurement technique and location will also affect the values obtained. Numerous studies have attempted to investigate and define normal ranges of BP in various species with variable results. In general, prehypertension is defined as SAP 140–150 mmHg, hypertension that may lead to potential target organ damage as SAP > 160–179 mmHg, and severe hypertension as SAP > 180 mmHg. Difficulties in defining a universally acceptable range of BP emphasize the importance of obtaining baseline BP values in patients prior to an anesthetic event, especially if concerns exist for target organ damage and/or underlying risk factors are identified (Box 12.2). Hypotension‐related morbidity and mortality is difficult to determine and most likely depends on a number of individual factors. In a study of over 30,000 humans undergoing non‐cardiac surgery, hypotension (defined as MAP < 55 mmHg) for as little as 1–5 min was associated with increased risk of acute kidney injury [5]. While large‐scale trials in veterinary medicine are lacking, the importance of maintaining perfusion during anesthesia should nonetheless be prioritized. For instance, use of excessive levels of inhalant leads to a significant decrease in SVR and a relative hypovolemia [6]. Hypotension is one of the most common complications of anesthesia and can have serious consequences if left unmanaged [1]. In horses, postanesthetic myopathy is a complex and potentially fatal consequence of inadequate tissue perfusion pressure during anesthesia. Multiple studies in horses have demonstrated increased risk of complications when MAP < 60 mmHg [7,8]; as such, common practice is to ensure that MAP is greater than 70 mmHg at all times [9]. Current information indicates that, although maintenance of MAP is important, maintaining global perfusion without increasing SVR should be prioritized, and vasoactive support agents should be selected accordingly [10]. The ability of organs to maintain a relatively constant blood flow despite changes in perfusion pressure is known as “autoregulation.” Organs perform this task intrinsically via three main mechanisms [11]. Metabolic regulation of blood flow occurs when changes in metabolism result in the release of vasodilatory substances such as adenosine, carbon dioxide, and lactic acid. An example of such metabolic regulation would be the vasodilation that occurs in muscle beds in response to hypoxia. Myogenic (pressure‐dependent) mechanisms autoregulate blood flow via smooth muscle‐lined vessels; increased pressure within the vessels leads to vasoconstriction, and decreased pressure is followed by vasodilation in smaller arteries and arterioles. Myogenic regulation of blood flow is observed in renal tissues. Shear‐stress‐dependent (endothelial) mechanisms regulate blood flow via the endothelial release of vasoactive factors such as nitric oxide and prostacyclin, causing vasodilation of smooth muscle‐lined vessels, as well as endothelin, which modulates vasoconstriction [12]. Such regulation may be observed in brain and cardiac tissue. Organs that demonstrate the greatest ability for autoregulation of blood flow include the brain, heart, and kidneys. The brain maintains autoregulation largely by metabolic means along with myogenic and endothelial responses. Recall that cerebral perfusion pressure (CPP) is the difference between MAP and intracranial pressure (ICP); it is critical to maintain constant CPP and cerebral blood flow (see Chapter 39). In humans, cerebral perfusion is autoregulated within an MAP range of 60–160 mmHg, where, in the dog, autoregulation of cerebral perfusion has been reported to occur with the MAP range of 70–140 mmHg [13,14]. In the kidneys, autoregulation of blood flow protects them from injury due to fluctuations of arterial BP and maintains the perfusion necessary to meet metabolic needs. Myogenic mechanisms of autoregulation allow for maintenance of constant renal blood flow (RBF) within the MAP range of 90–150 mmHg in rats, 70–130 mmHg in humans, and 40–100 mmHg in dogs [15,16]. Interestingly, RBF and glomerular filtration rate are maintained independently of renal perfusion pressure (RPP) via intrarenal autoregulatory mechanisms in the defined range of 80–180 mmHg in humans [17]. This safeguard is provided by a combination of myogenic and tubuloglomerular feedback that buffers transmission of changes in RPP to the capillaries of the glomeruli, protecting them from potential barotrauma while preserving renal function. During anesthetic events, the maintenance of systemic BP within ranges of autoregulation ensures consistent blood flow to the vital organs, including the kidneys, heart, and brain. Various studies in differing species and physiologic states demonstrate slight differences in ranges, so one recommended approach is to maintain BP within 20% of measured baseline BP taken prior to arrival in the operating room [18]. Invasive blood pressure (IBP) monitoring is considered the gold standard for BP measurement in anesthetized veterinary patients and allows for continuous, beat‐by‐beat assessment of BP and pulse waveform analysis. As this technique requires catheterization of an artery (thus the name “invasive”), it also facilitates sampling for arterial blood gas analysis. The term “direct” BP measurement is sometimes also used to describe this method; however, almost all IBP systems incorporate a transducer (see below) to convert the kinetic energy produced by changes in arterial pressure into electrical energy. SAP and DAP are directly measured with this technique, and MAP is calculated as the area under the arterial waveform over the cardiac cycle (Fig. 12.1). Careful setup and specialized equipment are needed to obtain the graphical display of the arterial pressure waveform and the numeric values; accuracy relies upon factors including the arterial catheter, the saline‐filled tubing connecting the catheter to the transducer, the transducer itself, and the electrical components. Figure 12.1 A. Mean arterial pressure (Pm) represents the area under the arterial blood pressure curve divided by the duration of the cardiac cycle. It can be estimated by adding one‐third of the difference between the systolic arterial pressure (Ps) and diastolic arterial pressure (Pd) to Pd. Ps minus Pd is the pulse pressure (PP). B. Ps increases and Pm and Pd decrease with decreasing arterial diameter. Patients where IBP monitoring should be considered for perioperative care include those where continuous monitoring of BP and/or repeated arterial blood gas/electrolyte sampling is necessary. These include high‐risk surgical and critically ill patients with anticipated rapid changes in hemodynamic status. Patients with comorbidities such as cardiac, pulmonary, or renal disease, significant risk of hemorrhage, and/or those receiving vasoactive substances (e.g., inotropes, vasopressors) should also be considered for IBP monitoring. Disadvantages of this technique include the cost or availability of advanced monitoring equipment and the need to catheterize a peripheral artery as well as potential risk of local pain, hemorrhage, hematoma formation, infection at the catheterization site, embolism, or ischemia/thrombosis (Fig. 12.2). A retrospective study examining the catheterization sites of 267 dogs and cats found loss of palpable pulse postoperatively in 22.3% of animals without evidence of associated tissue ischemia [19]. Care should always be taken to avoid potential complications. Arterial catheters should be clearly labeled to avoid inadvertent administration of drugs and/or other substances into this catheter. Postoperative care and management of arterial catheters in hemodynamically stable patients include the recommendation for its removal as soon as possible with the placement of a temporary pressure wrap. For additional blood gas sampling, the push–pull technique has been described from venous catheters in order to minimize blood loss, which is an especially important consideration for very small patients. This method was described using 20 gauge catheters placed in the cephalic vein of dogs. An empty 3‐mL syringe was used to aspirate 1.2 mL of blood from the intravenous (IV) catheter, then this blood was immediately pushed back into the catheter. This process is repeated three times. Finally, a new empty syringe is used to draw 1 mL of blood for immediate analysis. The IV catheter was then flushed with approximately 1 mL of saline [20]. It is important to note that this technique was utilized in venous catheters and has not been assessed in arterial catheters. Figure 12.2 A. Hemorrhage from displaced injection cap on a 22 gauge arterial catheter. The patient became tachycardic (> 180 bpm) and hypotensive (MAP < 60 mmHg). B. Blood loss was estimated to be 350 mL before disconnection was identified. Source: Dr. Odette O, with permission. While the use of an aneroid manometer alone has been described [21], standard IBP components typically include (1) an intra‐arterial catheter, (2) fluid‐filled, non‐compliant tubing, (3) a pressure transducer, and (4) signal conditioning and monitoring software. For peripheral arterial catheterization, a standard over‐the‐needle catheter that is kink‐resistant, biologically inert, and incompressible is used most often. The saline‐filled tubing forms a continuous, non‐compressible column of fluid producing “hydraulic coupling” between the arterial circulation and the transducer. In the clinical setting, most modern disposable IBP transducers are resistive devices that convert the mechanical (kinetic) energy arising from arterial pressure changes to changes in electrical resistance and ultimately an output voltage. Ohm’s law provides a useful framework for understanding how this system works: where R is the resistance (measured in ohms, Ω), P is the potential or voltage (measured in volts, V), and I is the current (measured in amperes, A). An ohm (Ω) is the basic unit of electrical resistance and, as defined by Ohm’s law, a resistance of 1 Ω allows 1 A of current to flow under the influence of 1 V of potential. Resistance‐type transducers are based on the principle that resistance of a wire (i.e., resistor) changes when it is subjected to “strain.” Resistance increases as the wire is put under tension (i.e., as it is stretched and becomes longer and thinner) and decreases as it is subjected to compression (i.e., as it becomes shorter and wider) [22]. Most modern commercial BP transducers utilize four strain gauges (resistors) that are bonded to a movable diaphragm and arranged in a Wheatstone bridge circuit. Mechanical pressure variations associated with arterial pulsations physically deform the diaphragm, which creates tension in two of the strain gauges and simultaneous compression of the other two. These strain changes lead to variations in electrical resistance and, because the Wheatstone bridge is very sensitive to small changes in resistance, the bridge becomes unbalanced, and the potential difference (i.e., voltage) generated is proportional to the pressure applied [23]. The resulting raw electrical signal output from the galvanometer is processed, amplified, and converted to values that are displayed graphically and numerically by the monitor [24]. The Wheatstone bridge also compensates for any temperature changes as all four strain gauges are affected equally. The transducer must be connected to the arterial catheter via a length of non‐compliant pressure tubing primed with normal saline to create a continuous non‐compressible fluid column. For most accurate results, the length of tubing and the number of stopcocks should be minimized. In most cases, the tubing packaged with commercial transducers is appropriate. Transducers may be connected to a pressurized bag of saline (with or without heparin added) to facilitate continuous flush of the catheter, or they may be intermittently flushed to maintain patency (see arterial catheter maintenance below). It is convention to place the arterial BP transducer at the level of the aortic root or base of the right atrium (RA) to ensure a common reference standard and accurate interpretation of IBP measurements (Fig. 12.3). Positioning the transducer below that level results in artifactually high pressures, whereas positioning it above that level results in artifactually low pressures. For every 10 cm that the transducer is positioned below the RA, the system will reportedly add 7.4 mmHg to displayed pressures, and vice versa [25]. Figure 12.3 Invasive blood pressure setup. The transducer is positioned at the level of the right atrium and zeroed to atmospheric pressure. Note the open‐ended (red) injection caps, which aid in maintenance of hygiene. Source: Dr. Odette O, with permission. While the processes of zeroing and leveling the IBP transducer tend to occur together in most clinical scenarios, they are actually different processes. Zeroing exposes the transducer to atmospheric pressure via an open air–fluid interface while leveling (as described above) assigns this zero‐reference point to a specific position relative to the patient’s body (i.e., the RA). To zero the transducer, thus eliminating the effects of atmospheric pressure, the stopcock is turned “off” to the patient, the system is opened to atmospheric pressure (i.e., the non‐vented cap from the stopcock is temporarily removed after opening it to room air), and the monitor’s zeroing function is activated. Once the system has been zeroed, the stopcock can then be turned off to the atmosphere and an arterial waveform should appear on the monitor [25]. While zeroing does not necessarily have to be performed with the transducer positioned at the level of the patient’s RA (as atmospheric pressure does not vary with these modest positional changes), rezeroing should be performed sporadically (i.e., several times per day) as both the transducer and atmospheric pressure will gradually drift away from the calibration point over time. The IBP monitoring system (i.e., catheter, fluid‐filled tubing, transducer, and electronics) is characterized as an underdamped, second‐order dynamic system [24]. The fluid column between the artery and the transducer can be described as a simple harmonic oscillator that, when displaced by a heartbeat, begins to oscillate. In a frictionless system, the oscillations would continue indefinitely at the system’s natural frequency; however, in a clinical patient, friction causes the system to oscillate briefly after a single displacement (i.e., one heartbeat) and then come to rest. This is analogous to a tennis ball dropped from a height that bounces several times before coming to rest on the ground [24]. The complex arterial pressure waveform is composed of one fundamental waveform (the first harmonic), which is equal to the pulse rate, and numerous other higher frequency/lower amplitude waveforms (second harmonic, third harmonic, etc.) that are multiples of the fundamental frequency. The IBP waveform is ultimately created by Fourier analysis, which sums the various sine waves of differing amplitudes and frequencies into a single complex waveform. If the natural frequency of the IBP system (i.e., the frequency at which it freely oscillates) is close to the frequency of any of the harmonics, the system will resonate leading to amplification of the signal and falsely wide and tall waveform peaks. Consequently, the IBP system should have the highest possible natural frequency (preferably above the eighth harmonic frequency) to minimize resonance distortion, especially in patients with faster HRs and steeper systolic upstrokes. As an example, a cat with a HR of 180 beats per minute (bpm) (i.e., 3 Hz) would require a system with a natural frequency greater than 24 Hz. A number of variables influence natural frequency including the radius and length of the tubing, the elasticity of the system, and the density of the fluid. By (1) shortening the length of the pressure tubing, (2) increasing the diameter of the pressure tubing, (3) using non‐compliant pressure tubing, and (4) using a low‐density fluid to fill the system (i.e., saline), the system’s elasticity is decreased and its natural frequency is increased [24]. Conversely, the presence of air bubbles in the system will increase elasticity and decrease the natural frequency. The dynamic response of the IBP system to pulse pressure oscillations from the cardiac cycle is determined by the balance of natural frequency (discussed earlier) and damping of the system. Damping is defined as the frictional forces that oppose the oscillations and result in decreased wave amplitude. It is described by the damping coefficient and, like natural frequency, is influenced by the diameter and length of the catheter and tubing. A damping coefficient of approximately 0.7 is considered optimal [24]. Over‐ and underdamping will affect the waveform and accurate measurement of IBP (Fig. 12.4). A dynamic pressure response test or “fast flush test” can be used to help identify damping issues. To perform this test, high pressure (> 300 mmHg) is rapidly introduced to the catheter from the continuous flush device for 1–2 s and then abruptly stopped. A square waveform is produced and information is derived by observing the oscillation pattern as the pressure returns to baseline (Fig. 12.5). Upon termination of the flush, the waveform should return to baseline within one to two oscillations, neither being greater than 1/3 the height of the previous oscillation. A waveform with a dicrotic notch should return thereafter. Absence of this feature indicates an overdamped waveform. The presence of many oscillations after the square wave indicated underdamping [26]. A smaller gauge catheter has a higher damping coefficient and is more likely to be overdamped than a larger gauge catheter. Other factors leading to an overdamped BP waveform include air bubbles, catheter obstruction (i.e., clot or kinking of catheter), pressure tubing that is overly compliant or long, and/or the use of too many stopcocks. Overdamping causes an underestimation of SAP, while DAP may either be accurate or overestimated [26]. Underdamping results in an overestimation of SAP and underestimation of DAP. MAP is the parameter least affected by system damping [25]. Recent literature recommends the use of IBP MAP instead of SAP in humans for interventional decisions while in the perioperative period [27]. Figure 12.4 Normal, overdamped, and underdamped arterial pressure waveforms. Figure 12.5 Dynamic pressure response test. A dynamic pressure response test is used to determine the resonant frequency and amplitude reduction ratio of a measuring system. A high pressure is applied to the measuring system while it is attached to the patient (usually with the continuous flush device). Sudden release of this pressure results in return of oscillations while the waveform returns to baseline. In a system with optimal damping, one to two positive and one to two negative oscillations should be observed before the waveform returns to baseline. Common sites for arterial cannulation in small animals include the dorsal pedal/metatarsal, palmar digital/metacarpal, radial, and coccygeal arteries; the femoral, brachial, and lingual arteries may also be used. In large animals, common sites include the auricular artery in cattle and branches of the facial or metatarsal artery in equids. The location of catheter placement affects values obtained (Fig. 12.1B), so different peripheral sites should not be used interchangeably for comparative results in patients [28–31]. Figure 12.6 Parks Doppler unit. Source: Dr. Carrie Schroeder, with permission. Catheter size varies from 24 to 22 gauge in very small patients, 22 to 20 gauge in medium and large dogs, and 20 to 18 gauge in large animals. Special care should always be taken to minimize the risk of intravascular catheter infections and guidelines in both human and veterinary medicine include staff education and training, hand hygiene, use of aseptic technique, and proper skin preparation as well as daily care after placement [32,33]. Indwelling arterial catheters require constant or intermittent flushing to remain patent. Heparinized saline is often used at various concentrations, but caution should be used in very small patients due to the risk of clinical effects with doses starting at 150 U/kg [34]. Recent studies have shown 0.9% sodium chloride to be as effective as heparinized saline for maintaining catheter patency [35,36]. Doppler ultrasonography is an indirect, non‐invasive method of measuring BP (NIBP). The equipment necessary for this technique includes a non‐directional transcutaneous Doppler unit, probe, sphygmomanometer, inflatable cuffs of various sizes, and ultrasonic coupling gel (Fig. 12.6). A small 8.0–9.9 MHz ultrasound probe is placed over a shaved peripheral artery with ultrasonic coupling gel. The probe contains piezoelectric crystals, traditionally composed of quartz and more recently of polarized ceramics. A 10‐mm beam of ultrasound wave contacts the artery at about 15° from perpendicular [37]. The waves generated from contact of the ceramics with pulsatile red blood cells within the peripheral artery result in deformation of the piezoelectric elements. As defined by the piezoelectric effect, deformation converts mechanical energy into electrical energy to receive the wave; then, realignment of the ceramics changes electrical energy back to mechanical energy, thereby creating sound waves; in other words, movement of red blood cells results in a change in pitch of the reflected sound waves [38]. Flat probes are designed to be taped into place for repeated use, while other shapes may be used for periodic pulse amplification. An amplifier connected to this probe intensifies the audible sound via a built‐in speaker or headphones [37]. Common peripheral arteries used for Doppler BP measurement include the palmar metacarpal, plantar metatarsal, dorsal pedal, and coccygeal. An inflatable cuff is placed proximal to the Doppler probe. The cuff should have a width measuring approximately 30–40% of the circumference of the limb; alternatively, the measurement guide provided by the manufacturer may be used. A sphygmomanometer is then attached and rapidly inflated until occlusion of the artery occurs, as demonstrated by loss of the audible signal, and then inflated an additional 20–30 mmHg. The cuff is then deflated slowly until a return of the audible signal (known as “Korotkoff sounds”) occurs. The sphygmomanometer pressure correlating to return of the first Korotkoff sound is widely accepted as SAP in most species. While changes in the character of the Korotkoff sounds may be appreciated as the cuff is deflated, DAP is not reliably estimated by this method, so calculation of MAP is not possible using this method of BP measurement. In cats, Doppler NIBP measurement tends to underestimate BP when compared to invasive femoral artery measurements, with Doppler SAP +14 mmHg more closely representing invasive femoral SAP values [39]. Nonetheless, it should be noted that in dogs < 5 kg, Doppler BP most closely reflects SAP and was found to be highly specific in predicting hypotension in anesthetized small dogs [40]. The advantages of Doppler NIBP measurement include that it is non‐invasive, inexpensive, portable, provides real‐time audible pulse sounds, and is easy to use in a variety of species. Disadvantages of this technique include that the cuff must be inflated manually, only a single value is provided for BP, and that electrical interference from other equipment (e.g., electrocautery) may result in excessive noise. Oscillometric BP measurement technology has been commercially available since the 1970s and remains popular today. The reasons for its popularity across a variety of species include ease of use, minimal equipment requirements, and automation. With many device options on the market, a multiorganizational universal standard for validation of this equipment for use in human patients was set in 2018 [41]. Figure 12.7 Blood pressure cuff width should be 30–40% of the limb circumference for accurate measurement. To use the range guidance, roll the edge of the cuff around the limb, where the index line falls within the range guide when secured onto the limb of use. Source: Dr. Odette O, with permission. In general, this is a counterpressure measurement technique whereby the arterial pulse produces changes in the volume of a limb, which can be transmitted to and detected as changes in pressure within a cuff encircling the limb. An inflatable cuff is placed over the artery of a limb or the tail and attached with tubing to a monitor containing a microprocessor and pressure sensor (transducer) and, in some monitors, an amplifier and filter. The tongue has also recently been reported for use in canine patients, with MAP meeting ACVIM and American Association for Medical Instruments criteria for a wide range of pressures. However, it should be noted that SAP accuracy and precision was poor, only meeting ACVIM criteria during states of hypotension [42]. Identical to Doppler NIBP measurement, the cuff should have a width measuring approximately 30–40% of the circumference of the limb or the measurement guide provided by the manufacturer should be used (Fig. 12.7). Oscillometric devices inflate the cuff to a pressure approximately 20–30 mmHg above that needed to obstruct blood flow and then slowly deflate it using either continuous linear or nonlinear (step‐deflation) methods. Automatic cuff deflation allows for the gradual return of arterial blood flow and the corresponding changes in cuff pressure amplitudes (i.e., oscillations) persist until inflation pressure is released and blood flow returns to normal. The monitor records this series of cuff pressure amplitudes (i.e., absent, increasing to a maximum, then decreasing until absent) and analyses them. Microprocessors with various proprietary empirical algorithms then determine values for SAP, MAP, and DAP. All oscillometric NIBP monitors make use of these proprietary algorithms, making comparison between monitors from different manufacturers a challenge. There are no current regulatory standards governing how these systems obtain cuff pressure amplitudes and determine BP. The result is that accuracy, validation as compared to IBP, agreement among monitors, as well as repeatability remains a challenge in human and veterinary medicine alike. In addition, there are also no current reliable instruments to test the accuracy of these various algorithms or their performance, leaving the consumer to rely heavily on the manufacturers for quality assurance and on publications that attempt to establish formulas to illuminate details [43,44]. The MAP is considered the most valid pressure value provided by oscillometric NIBP systems, with SAP and DAP being calculated via manufacturer‐specific proprietary algorithms [43]. Three of the most popular empirical techniques include the maximum amplitude, derivative, and fixed‐ratio algorithms. The maximum amplitude algorithm compares cuff pressure amplitudes to determine the point of maximal (peak) oscillations, which is measured as the MAP. There are various published methods for determining SAP and DAP based on the ratio of oscillation amplitudes, and different investigators have proposed different set points or ratios for SAP and DAP [43,45]. To add to this confusion, there is at least one study that has called into question the widely held assertion that the formula for the maximum amplitude algorithm represents MAP. Instead, they suggest that this algorithm actually estimates a weighted average of SAP and DAP where weighting is determined by arterial compliance curve widths [44]. Regarding the other techniques, the derivative algorithm estimates DAP and SAP from the slope of the oscillogram, while with the fixed‐ratio algorithm estimates DAP and SAP as population‐based fractions of their peak values. This last algorithm is prone to amplifying error, resulting in inaccurate BP measurements [44]. SunTech Medical, Inc. created the NIBP technology that is incorporated into many of the commonly used veterinary multiparameter monitors today, including the Digicare, DRE Veterinary, Midmark, Nonin, Smiths Medical (Surgivet), and Vmed Technology, in addition to human‐based products such as the Masimo Root®. This manufacturer also produces a veterinary‐specific line of standalone NIBP monitors reported to function at pulse rates of 25–300 bpm in small animals and 15–150 bpm in horses. These monitors measure SAP, MAP, and DAP at ranges of 40–265, 27–222, and 20–200 mmHg, respectively. The system uses a default inflation pressure of 180 mmHg, with subsequent inflations set at SAP +30 mmHg with a minimum inflation pressure of 120 mmHg. These monitors use nonlinear (stepwise) deflation for increased tolerance to motion and MAP determination based on their proprietary maximum amplitude and SAP and DAP calculation algorithms. The veterinary models also have reference ranges, changes in gain based on differing pulse volumes based on patient size, and internal manufacturer validation designed from specific animal populations, including small animals and horses [personal communication: P. Matsumura, SunTech Medical; September 7, 2021] Limitations to accurate performance of the oscillometric method of BP measurement include situations of reduced blood flow, poor cuff sizing, patient movement/shivering, extremes of patient size, severe hypotension/hypertension, and cardiac arrhythmias. The uneven pulse waveforms created by arrhythmias result in an inaccurate MAP and subsequent SAP/DAP determinations. Oscillometric NIBP measurement is also intermittent, with lag time varying based on user settings. High‐definition oscillometry (HDO) is a non‐invasive means of BP measurement that uses an HDO device with a 32‐bit processor in conjunction with a computer or tablet, enabling real‐time visualization of the pulse waveform during cuff inflation/deflation and assessment of validation through recognition of artifacts [46]. Gain is a feature unique to HDO. The system can optimize gain (amplify signal) and deflation rates to read low BPs and complete readings often within 8–15 s. The HDO permits a scan bandwidth of 16,000 Hz compared to an input of 50/60 Hz used in standard oscillometric devices. The system in units such as the HDO Vet MD PRO utilizes linear deflation and a veterinary‐specific algorithm that enables measurement of SAP, MAP, and DAP. Coccygeal artery assessment is recommended, so tail base cuff placement is necessary, though the antebrachium can also be used in patients where coccygeal access is not possible. Software enables in‐depth pulse wave analysis for users to assess functional parameters including SV, SVR, and SV variance. In anesthetized canine patients, HDO BP measurement has been shown to meet ACVIM consensus panel validation criteria for MAP and DAP but not SAP, where it was found to be unreliable in hypotensive dogs [47]. In awake cats, this BP technique met the criteria for SAP, but underestimated DAP [48]. Central venous pressure (CVP) measures the hydrostatic pressure of the intrathoracic vena cava, as a close reflection of right atrial pressure (RAP) [49]. Traditionally, this modality has been considered an indirect indicator of intravascular volume and fluid responsiveness in veterinary patients. Although alternative means of measurement of CO, perfusion, and volume status exist, CVP measurement is still utilized in veterinary medicine due to its practicality, low cost, and relative ease of use. Lack of consistent and repeatable reference ranges for CVP across species, however, introduces challenges when it comes to interpretation of these values in veterinary medicine. CVP is actually a measure of intravascular pressure in relation to atmospheric pressure, whereas true RAP and right ventricular (RV) pressure (used as surrogates of left ventricular (LV) end‐diastolic volume and cardiac preload) are transmural pressures. The complex relationship between CVP, CO, and the vascular system further complicates interpretation, despite values themselves being relatively easy to obtain [50]. In addition, the nonlinear nature of the Frank–Starling curve along with factors causing changes in cardiac and pulmonary compliance results in circumstances where pressure does not correlate well with volume status [51]. Consequently, CVP has fallen out of favor in human medicine in a number of situations, including guidance of fluid resuscitation in septic shock [52], while others still encourage its use with a clear understanding of the caveats [53]. Current veterinary publications on this topic remain inconclusive. CVP should not be used as a single static measurement to make clinical decisions. Instead, it should be considered as part of a clinical picture, in conjunction with repeated readings and assessments of trends in the individual patient of interest, along with use of clinical values such as HR, arterial BP, lactate trends, and pulse quality for assessing patient hydration and perfusion status. In horses, increased lactate in combination with decreased CVP was noted in subjects experiencing blood loss despite insignificant changes in HR and venous blood gas values [54]. Patients that present with concerns related to their volume status and/or overhydration are potential candidates for CVP measurement as part of an overall clinical assessment. These patients may include high‐risk anesthesia patients with shock or ongoing hemorrhage requiring large volume resuscitation and those with significant renal or cardiac disease. Catheterization of the vena cava is necessary for the measurement of CVP. The external jugular vein is most often used in veterinary medicine, due to its size, easy accessibility, and ability to maintain hygiene. Radiographic confirmation of correct placement should verify that the tip of the catheter is located at the entry to the RA. A catheter originating in the lateral saphenous vein (dogs) or medial saphenous vein (cats) may also be used; however, the catheter must be advanced into the thoracic vena cava at the level of the RA for accurate CVP measurement. It should be noted that peripheral venous pressure was poorly correlated with CVP in dogs and cats with stable cardiovascular status [55]. Various central line kits, both veterinary and non‐veterinary, are commercially available. The use of prepackaged kits permits sterile placement of these catheters using the modified Seldinger technique and has been found to increase procedural quality through decreased technical errors and time required [56]. With appropriate care, these catheters may safely remain in place for multiple days. The normal reference range for CVP is 0–10 centimeters of water (cmH2O), more commonly 0–5 cmH2O [49]. Depending on the technique used for measurement, millimeters of mercury (mmHg) can be converted to cmH2O using a conversion factor of 1.36 (i.e., 1 mmHg = 1.36 cmH2O). In the absence of other complicating factors, patients demonstrating negative values should be assessed for hypovolemia, while those in the range of 0–5 cmH2O may be considered euvolemic, and those with values trending beyond 7–10 cmH2O may be at increased risk for hypervolemia. These values are more helpful when considering the response to fluid challenges, rather than single‐point determinations about volume status since many factors alter interpretation. For instance, the absence of a decrease in CVP that is normally observed during inspiration in fluid responsive patients (known as “Kussmaul’s sign”) may indicate concern for fluid intolerance [49,51]. Two methods may be utilized for the measurement of CVP. Readings should be performed in a consistent manner with the patient in the same body position for all measurements, the system leveled at the RA, and values taken at end‐expiration. Historically, a water column setup was used, reflecting the common units used for CVP measurement, cmH2O. This system offers the advantages of being low cost and requiring minimal equipment (i.e., a central line kit, ruler, IV pole, non‐compliant tubing, three‐way stopcock, and fluids). However, challenges with this setup include the need to manually level the zero point of the water manometer at the level of patient’s RA and the lack of a waveform for analysis. Alternatively, an electronic pressure transducer system may be used to measure CVP. The setup is identical to that used for IBP measurement, as described previously. Benefits to use of this system include constant, real‐time monitoring, and the production of a waveform for analysis (Fig. 12.8). Values from these electronic systems may be expressed in mmHg, depending on the manufacturer and monitor settings. Figure 12.8 Central venous pressure (CVP) tracing and waveform. Note the features of the CVP waveform throughout the cardiac cycle: A wave represents right atrial contraction, seen after ECG P wave but prior to QRS; C wave reflects the tricuspid valve being pushed up into the atrium during ventricular contraction (note: this wave may or may not be evident); X descent reflects right atrial relaxation; V wave reflects rapid atrial filling with plateau; and Y descent reflects ventricular relaxation and early ventricular filling. The decision to perform CVP monitoring should be made with a risk/benefit analysis. Aside from the limitations in accurately assessing vascular volume status and fluid responsiveness, there has been much controversy regarding its ability to predict morbidity and mortality in human medicine and its use and interpretation in veterinary medicine should be viewed with caution [51–53]. Highly skilled personnel are needed for both placement and maintenance of a central line as well as interpretation of the results. Potential immediate risks associated with jugular catheter placement are cardiac arrhythmias (most commonly atrial or ventricular premature contractions if a guide wire or catheter is advanced into the heart), hemorrhage, hematoma, air embolus, thrombus, pneumomediastinum, pneumothorax, hemothorax, tracheal trauma, and inadvertent carotid artery puncture [57]. Longer‐term concerns include catheter‐associated infection, recurrent laryngeal nerve damage, and jugular vein stenosis or occlusion. Contraindications to this approach include patients with concerns for increased intracranial pressure (ICP), coagulopathies, and those at increased risk of thromboembolic events. As such, current recommendations in veterinary medicine are to use CVP trends for additional data to assess patient needs, but central venous access should not be undertaken solely for this purpose [58]. The circulatory effects in dogs provided intermittent positive‐pressure ventilation (IPPV) were first measured almost 60 years ago, with the cause of these effects attributed to the effect of intrathoracic pressure on venous return [59]. Since then, many studies have noted the variation in the arterial pressure waveform during IPPV and demonstrated that in mechanically ventilated patients, under certain circumstances, this physical sign can be an accurate predictor of fluid responsiveness. Pulse pressure variation (PPV) is a measure of the difference between systolic and diastolic pressures during IPPV inspiratory and expiratory phases. In‐depth reviews of the physiologic changes that occur during mechanical ventilation may be found elsewhere [60]. In short, increased pleural pressure due to positive‐pressure ventilation compresses the vena cava and increases RAP, resulting in decreased venous return. In turn, decreased RA preload decreases RV output and pulmonary artery blood flow. Finally, LV filling and, thereby, output are decreased. Three additional mechanisms may cause respiratory variation via alteration of LV SV during the inspiratory phase of IPPV: (1) RV ejection is impeded by increased RV afterload resulting from increases in alveolar pressure that exceed increases in pleural pressure; (2) the increase in alveolar pressure relative to pleural pressure also sends blood from the alveolar capillaries toward the left side of the heart, resulting in increased LV preload; and (3) LV afterload is decreased via the reduction in thoracic blood volume resulting from increased systolic extracardiac pressure along with decreased systolic intracardiac pressure from the positive pleural pressure. In summary, during the IPPV inspiratory phase, RV preload is decreased, while RV afterload is increased; and LV preload is increased, while LV afterload is decreased. The RV output decrease is then translated a few beats later to the left heart. The main determinant of respiratory variation in PP is the variation in LV SV. In general, hypovolemic patients demonstrate greater respiratory variations in SV and arterial pressure for four main reasons: (1) the vena cava is more collapsible during hypovolemia; (2) an underfilled RA during hypovolemia will be more sensitive to the transmission of pleural pressure during inspiration; (3) the effect of inspiration on RV afterload is more significant during hypovolemia where West’s zone 1 (pulmonary arterial pressure < alveolar pressure) and/or West’s zone II (pulmonary venous pressure < alveolar pressure) conditions are likely met; and (4) during hypovolemia, both left and right ventricles are more likely functioning on the steep portion of the Frank–Starling curve where there is a greater sensitivity to preload changes than if operating on the flatter portion of the curve (Fig. 12.9). Thus, during hypovolemic conditions, patients demonstrate greater respiratory variation in PP. When these waveforms are displayed on patient‐side monitors, they can be a valuable tool in assessing fluid responsiveness during IPPV. Waveform variation requires quantification for true utility since monitors variably record arterial waveform at differing speeds and scales. Analysis of these waveforms has been described by various methods including systolic pressure variation (SPV), which is the difference between maximum and minimum SAP over a single respiratory cycle. Assessment of systolic reference pressure at the end‐expiratory pause then allows differentiation between inspiratory increase and expiratory decrease. When PPV was first described in critically ill patients in 1978, SPV > 10 mmHg was observed in hypovolemic patients with SPV < 10 mmHg seen in normo‐ and hypervolemic patients [61]. Alternatively, there is a different calculation with the aim of monitoring changes in LV SV where respiratory variation in arterial PP is quantified by determining the difference between maximum and minimum PPs during one mechanical breath with the mean of the two values expressed as a percentage [62]:
12
Blood Pressure Monitoring
Introduction
Blood pressure determinants and definitions
Mean arterial pressure
Cardiac output
Systemic vascular resistance
Normal ranges of blood pressure
Autoregulation of blood pressure
Invasive blood pressure measurement
Components for invasive blood pressure measurement
Transducer setup and priming of pressure tubing
Leveling the transducer
Zeroing the transducer
Physics of invasive blood pressure monitoring systems
Natural frequency and resonance
Dynamic response testing and damping
Arterial catheter placement and maintenance
Non‐invasive blood pressure measurement
Doppler ultrasonographic blood pressure measurement
Oscillometric blood pressure measurement
High‐definition oscillometric blood pressure measurement
Central venous pressure measurement
Indices of fluid responsiveness
Pulse pressure variation and systolic pressure variation
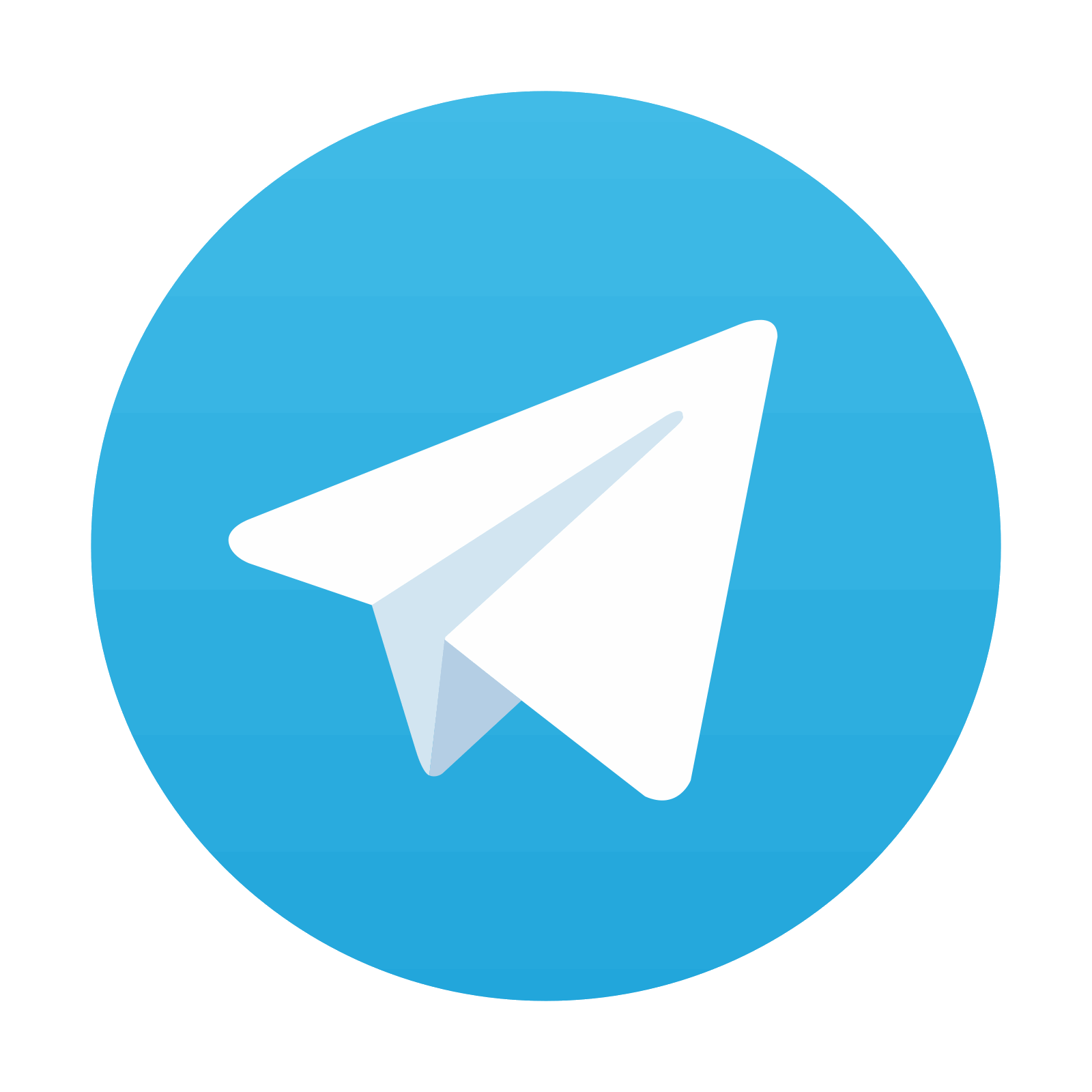
Stay updated, free articles. Join our Telegram channel
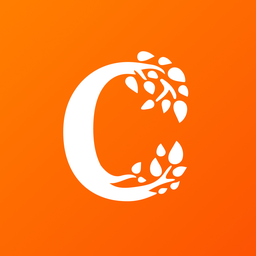
Full access? Get Clinical Tree
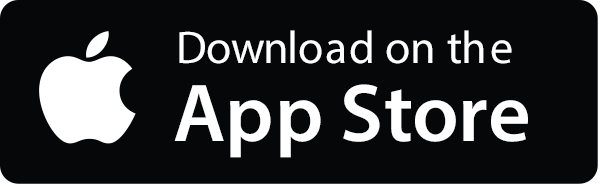
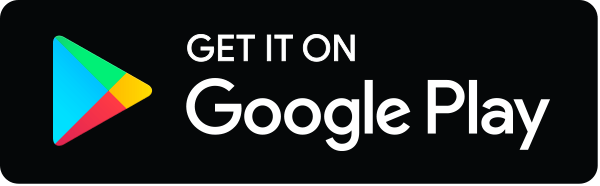