4 Thomas F. Murray Creighton University, Omaha, NE, USA Acetylcholine was the first compound to be identified as a neurotransmitter in the peripheral nervous system; however, knowledge concerning the anatomical organization of cholinergic neurons lagged behind other transmitter substances due to the lack of suitable techniques for mapping cholinergic pathways. Many of these technical obstacles have been surmounted in recent years. Acetylcholine is synthesized in cholinergic neurons by a reaction catalyzed by the cytosolic enzyme choline acetyltransferase (CAT). This linkage of choline and an acetate group provided by acetyl‐CoA is not, however, the rate‐limiting step in the biosynthesis of acetylcholine (ACh); rather, the high‐affinity transport of choline from the extracellular medium by a specific transporter protein represents the rate‐limiting step. ACh is stored in vesicles in cholinergic terminals and released into the synaptic cleft by exocytosis (Figure 4.1). The proteins involved in this exocytotic release of ACh are the targets for botulinum toxin; the therapeutic actions of Botox in the treatment of neuromuscular disorders derive from the ability of this toxin to block the release of ACh from cholinergic neuron terminals (Coffield 2003). Acetylcholine released from neurons into the synaptic cleft is hydrolyzed by the membrane bound enzyme, acetylcholinesterase (ACHE), which represents the mechanism for termination of the signal in cholinergic neurotransmission. ACHE has one of the highest catalytic powers ever reported for an enzyme and is therefore capable of a rapid clearance of ACh from the synaptic cleft. This distinguishes cholinergic neurons from those of other biogenic amines where the synaptic signal is terminated by the high‐affinity reuptake of the transmitter. Acetylcholine is the neurotransmitter of all autonomic ganglia, postganglionic parasympathetic synapses, the neuromuscular junction, and cholinergic neurons in the central nervous system. Figure 4.1 Schematic representation of a cholinergic synapse in the central nervous system. The precursor for acetylcholine (ACh) synthesis is choline. ACh is synthesized from choline and acetyl CoA by the enzyme choline acetyltransferase. The ACh is stored in and released from vesicles. Synaptic ACh activates postsynaptic receptors comprised of both muscarinic and nicotinic subtypes. The synaptic action of ACh is terminated by the enzyme acetyl cholinesterase (AChE) through hydrolysis to acetate and choline. The choline is salvaged by a high affinity transporter for reutilization in the syntheses of ACh. Table 4.1 Interaction of antidepressants with the norepinephrine (NET) and serotonin (SERT) transporters in vitro. Note: The antidepressants are listed in decreasing order of NET selectivity. Cholinergic pathways in the brain have now been identified by histochemical and neurochemical methods (Figure 4.2). The majority of cholinergic neurons in the mammalian brain are found in four regions. These include: (i) the brainstem pedunculo‐pontine and lateral dorsal tegmental nuclei; (ii) a subset of the thalamic nuclei; (iii) the striatum, where cholinergic neurons serve as local interneurons; and (iv) the basal forebrain nuclei, which collectively serve as the major sources of cholinergic projection neurons to the cerebral cortex, hippocampus, and amygdala (Ballinger et al. 2016). The cholinergic neurons originating in the nucleus basalis of Meynert, substantia innominata, and the diagonal band (basal forebrain) project to virtually all cerebral cortical areas and layers (Sarter et al. 2016). These projections terminating throughout the cerebral cortex degenerate in senile dementia and Alzheimer’s disease and this cholinergic neuron loss has been correlated with the degree of cognitive decline. The ACh content of the brain in Alzheimer’s patients at postmortem is profoundly reduced. These observations have led to the cholinergic hypothesis of cognitive decline in senile dementia that has served as the basis for pharmacological attempts to ameliorate learning and memory deficits by restoration of cholinergic neurotransmission. One pharmacologic strategy for augmenting cholinergic transmission has been the use of inhibitors of ACHE. Inhibition of this enzyme prolongs the half‐life of ACh in the synapse and therefore enhances cholinergic transmission. Drugs such as tacrine, donepezil (Aricept®) and rivastigmine (Exelon®) are ACHE inhibitors (ACHEI) that have been used in the treatment of cognitive dysfunction associated with Alzheimer’s disease. These drugs produce modest beneficial effects for periods of approximately one year, but do not prevent the progressive deterioration of mental function in Alzheimer’s patients. Inhibition of ACHE has moreover a high propensity for adverse effects and a significant fraction of patients withdraw from treatment due to side effects. Figure 4.2 Schematic diagram of the cholinergic pathways in animal brain. Central cholinergic neurons exist as both local circuit interneurons and projection neurons. Abbreviation: BMSI = Basal Nucleus of Meynert/Substantia Inominata. Cholinergic receptors that mediate the synaptic actions of ACh are either muscarinic or nicotinic cholinergic receptors. The muscarinic receptors are members of the G‐protein coupled receptor family and five subtypes of muscarinic cholinergic receptors (M1–M5) have been characterized. Muscarinic receptor activation therefore trigger their signaling through activation of heterotrimeric G proteins that in turn affect the opening, closing, and kinetics of K+, Ca2+, and non‐selective cation channels. The alkaloids, atropine and scopolamine, act as nonselective, competitive antagonists of these muscarinic receptors. Both atropine and scopolamine are capable of disrupting working memory in humans and animals through their antimuscarinic actions in the cerebral cortex and hippocampus. High doses of either drug can also produce delirium, hyperactivity, visual hallucinations, and disorientation. The M2 muscarinic receptor predominates in hindbrain regions while the M1 receptor is expressed at high levels in the cerebral cortex and hippocampus which are brain regions involved with memory and cognition. The M1 muscarinic receptor has accordingly generated considerable recent interest as a target for drug discovery of agents that might facilitate learning and memory. In animal models, M1 muscarinic receptor agonists restore cognitive impairment associated with damage to cholinergic pathways and may exert additional disease‐modifying effects beneficial to Alzheimer’s disease (Fisher et al. 2003). The other class of cholinergic receptors that exist in the central nervous system are nicotinic receptors. These ACh receptors are members of the ligand‐gated ion channel superfamily and are composed of multiple homologous subunits oriented around a central cation channel. Twelve different types of nicotinic receptor subunits have been identified in the brain (α2–10 and β2–β4) and each nicotinic cholinergic receptor is composed of five of these subunits. The combination of these subunits into a pentameric structure determines its electrophysiological and pharmacological properties. It takes the binding of two molecules of ACh to open the cation channel and allow the Na+ influx and depolarization of neuronal membranes. The most common nAChR subtypes in the brain are α4β2 and α7 receptors. In addition to responding to ACh, these receptors, as their name implies, are also activated by the alkaloid nicotine found in tobacco leaves. Nicotine shares with other drugs of abuse the ability to activate the mesocortical limbic dopaminergic pathways that represent the reward circuitry of mammalian brain. The interaction with nicotinic cholinergic receptors in this pathway underlies the rewarding and dependence‐related actions of nicotine. In addition to the adverse addictive properties of nicotine, this naturally occurring product increases mental alertness and improves memory in humans and animals. Chronic transdermal nicotine has indeed been found to improve attentional performance in Alzheimer’s disease patients and in individuals with the precursor age‐associated memory impairment syndrome (White and Levin 2004). There is therefore a great deal of interest in the pharmaceutical industry directed toward the development of synthetic nicotinic receptor agonists for the treatment of memory deficits and cognitive dysfunction. There are currently several nicotine analogs in various stages of preclinical and clinical development for use in neurodegenerative and Alzheimer’s disease to mitigate the cognitive effects of the disease and delay clinical cognitive manifestations. The neurotransmitter norepinephrine is distributed throughout the brain. The relatively low concentration of norepinephrine in the brain, however, initially led physiologists to question its functional importance. Application of a fluorescent histochemical technique subsequently permitted the visualization of norepinephrine containing neurons and pathways in both the central and peripheral nervous system. Norepinephrine, dopamine, and epinephrine are catecholamines. The microanatomy of catecholamine‐containing neurons in the central nervous system is distinct from amino acid and cholinergic fibers in that they possess varicosities along the axons in terminal fields. These varicosities contain all the machinery for neurotransmitter synthesis, storage, and release and are therefore the points of synaptic contact with target neurons. This unique microanatomy therefore allows a single catecholamine neuron with long terminal branches to possess thousands of varicosities. One catecholamine neuron consequently can influence the activity of thousands of target neurons. The biosynthesis of norepinephrine in central neurons begins with the precursor tyrosine. Tyrosine is converted to l‐dopa by the soluble enzyme tyrosine hydroxylase (Figure 4.3). Tyrosine hydroxylase is the rate‐limiting step in the biosynthesis of norepinephrine and the activity of this enzyme is closely coupled to the neuronal firing rate. The l‐dopa is in turn converted to dopamine by the enzyme l‐dopa decarboxylase. In noradrenergic, but not dopaminergic, neurons the dopamine is converted into norepinephrine by dopamine‐β‐hydroxylase and stored in vesicles for release. Once the norepinephrine is released into the synaptic cleft, the signaling action of this transmitter is terminated by the selective, high affinity of reuptake of norepinephrine by a distinct transporter expressed on noradrenergic neurons (NET). Amphetamine and related psychostimulants exert their sympathomimetic and central stimulant properties through the inhibition of norepinephrine reuptake and the facilitation of the transmitter release. Figure 4.3 Schematic representation of a noradrenergic synapse. The amino acid precursor tyrosine is converted to l‐dopa by the enzyme tyrosine hydroxylose (TH) in noradrenergic neurons. The l‐dopa is the converted to dopamine by l‐aromatic amino acid decarboxylase (L‐AADC), and the dopamine is in turn converted to norepinephrine (NE) by dopamine‐β‐hydroxylase (DBH). The NE is stored in and released from vesicles, and synaptic NE activates a complement of pre‐ and postsynaptic receptors. Synaptic NE action is terminated by the reuptake of the transmitter into the presynaptic terminal. This reuptake is mediated by the plasma membrane NE transporter (NET). Cytoplasmic NE can be degraded by the mitochondrial enzyme monoamine oxidase (MAO). Presynaptic release modulating autoreceptors are the α2‐adrenergic subtype. Norepinephrine interacts with both alpha and beta adrenergic receptors expressed in the brain. There are eight subtypes of adrenergic receptors expressed in the brain including alpha‐1A, 1B, 1D, alpha‐2A, 2B, 2C, beta‐1 and beta‐2 adrenergic. All adrenergic receptors are G‐protein coupled receptors. While the release of all neurotransmitters is regulated by presynaptic autoreceptors that respond to the released transmitter, this was first demonstrated in noradrenergic neurons. The noradrenergic neuron autoreceptors are members of the alpha‐2 adrenergic receptor class. Agonists for alpha‐2 adrenergic receptors, such as clonidine, produce an inhibition of norepinephrine release, while alpha‐2 antagonists, such as yohimbine, increase the amount of norepinephrine released from presynaptic terminals. Given the existence of three subtypes of alpha‐2 adrenergic receptors, the identity of the subtypes representing presynaptic autoreceptors was addressed using gene knockout strategies in mice. This approach has shown that both alpha‐2A and alpha‐2C‐adrenergic receptors are involved in the presynaptic control of transmitter release in noradrenergic neurons (Hein et al. 1999). Noradrenergic neurons in the brain emanate from cell bodies in the pons (Figure 4.4). The primary nuclear complex from which noradrenergic neurons arise in the mammalian brain is the locus ceruleus. Norepinephrine neurons belonging to this nuclear complex branch widely in their terminal fields, allowing, for example, a single norepinephrine axon to innervate a large fraction of the cerebral cortex. Locus ceruleus noradrenergic efferents innervate target cells in cerebral cortical, subcortical, and spinomedullary fields. The sole source of norepinephrine input to the cerebral cortex and hippocampus, brain regions critical for cognitive and affective processes, is derived from the locus ceruleus. Collectively, this noradrenergic system plays a critical role in the regulation of arousal, attention, mood, and cardiovascular function. An additional group of noradrenergic neurons lies outside the locus ceruleus where they are sparsely distributed throughout the lateral ventral tegmental area of the pons (Cooper et al. 2003).
Biogenic Amine Transmitters: Acetylcholine, Norepinephrine, and Dopamine
Acetylcholine
Drug
KI (nM) NET
KI (nM) SERT
NET Selectivity (ratio SERT/NET)
Desipramine
0.77
288
374
Reboxetine
8
1070
130
Nortriptyline
4.34
190
44
Doxepin
40
355
9
Amitriptyline
27
107
4
Imipramine
28
37.2
1.3
Fluoxetine
1235
17.7
0.014
Sertraline
220
3.4
0.015
Paroxetine
161
0.033
0.0002
Norepinephrine
Stay updated, free articles. Join our Telegram channel
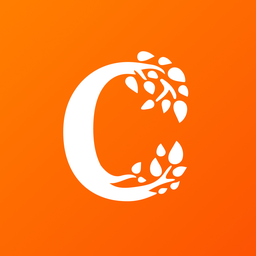
Full access? Get Clinical Tree
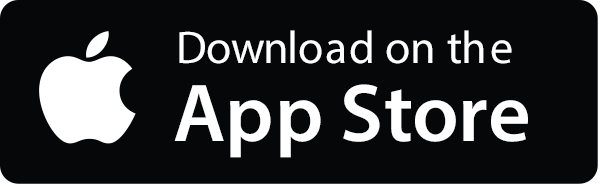
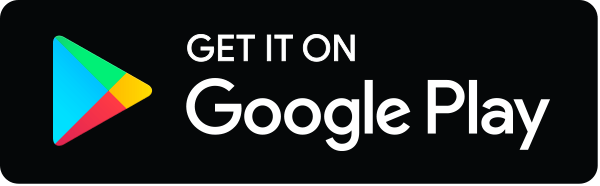