Kahina Boukherroub and Sally L. Noll The musculoskeletal structure of birds can vary tremendously based on whether they fly or not. The majority of the muscles can be concentrated on the torso for birds that rely primarily on flight to move, as is the case in grouse, whereas birds that rely mostly on running, such as the pheasant and quail, have larger leg muscles [1]. Skeletal muscle cells are elongated, multinucleated cells, called myofibers. At hatch, chicks possess fully differentiated skeletal muscle cells, and muscle growth that occurs thereafter is due to an increase in muscle fiber size [2]. During early stages of embryonic development, the primordial muscle cells, called myoblasts, concentrate in a block called the myotome. As development progresses, the myoblasts migrate from the myotome into their muscle beds where they continue to proliferate. Some myoblasts fuse with each other to form primary multinucleated muscle fibers, others continue to proliferate and eventually fuse into secondary muscle fibers. The formation of muscle fiber is complete at hatch and subsequent muscle growth is due to the enlargement of existing fibers in a process called hypertrophy [2, 3]. Chicks, for most common gamebirds, are precocial and possess relatively well‐developed leg muscles at hatch. This is due to high levels of circulating maternal thyroid hormone during embryonic development and highly active thyroid during the perinatal period [4, 5]. Muscle hypertrophy occurs by the fusion of satellite cells located between the basement membranes of muscle fibers [6, 7]. The proliferation potential of satellite cells is higher in younger animals and diminishes as the animal gets older. Much of what is known about factors regulating the proliferation of satellite cells is derived from in vitro studies in cultured chicken and turkey embryonic myoblast satellite cells [1]. Among these factors, insulin‐like growth factor (IGF) was found to enhance chicken myoblast differentiation and turkey myoblast proliferation [8]; fibroblast growth factor (FGF) was found to enhance turkey myoblasts and satellite cell proliferation [9, 10]; platelet‐derived growth factor (PDGF) was found to enhance chicken myoblast proliferation [11, 12]; hepatocyte growth factor (HGF) was found to activate and enhance proliferation of dormant chicken and turkey satellite cells [13, 14]; transforming growth factor beta (TGF‐beta) was found to inhibit both proliferation and differentiation of turkey satellite cells [15] but when added with FGF, it leads to chicken myoblast differentiation [16]; and myostatin was found to inhibit turkey and chicken myoblast and satellite cell differentiation [17, 18]. Muscle morphology is an important determinant of meat quality, and focusing on fast growth rates and muscling alone results in poor meat quality that displays pale, soft, exuding meat – PSE. When cooked, PSE results in soft, poor water binding and poor juiciness. Muscle morphology defined as extracellular matrix spacing and muscle fiber size was determined to be inherited from the mother in turkeys [19]. Although these studies were done in turkeys, maternal inheritance of muscle morphology could be a contributing factor in muscle morphology in gamebirds, so selection of sires and dams during breeding should be carefully considered. The avian skeleton evolved to support flight, locomotion, and protection of the organs. It is a dynamic tissue that responds and adapts to physical stressors and is constantly undergoing remodeling. It also serves as a reservoir for calcium, phosphate, and other ions. The two main tissues of the skeleton are bone and cartilage. Cartilage is capable of growing quickly and that is why it is abundant in embryos and young individuals. Bone is more rigid and strong and predominant in the adult skeleton. Bone and cartilage parts are interconnected by joint structures, including synovial joints that connect between limb elements, intervertebral discs that confer pliability on the vertebral column, and fibrous joints to minimize movement between skull bones [20]. Bone acquisition during growth is referred to as bone modeling. It occurs in one of two ways: endochondral ossification or intramembranous ossification. During endochondral ossification, a cartilage anlagen is first formed, in a process referred to as chondrogenesis, followed by its replacement by bone. During embryonic development, progenitor cells that undergo chondrogenesis derive from the ectoderm and mesoderm. These mesenchymal cells condense and differentiate into cartilage‐secreting cells known as chondroblasts. The resulting cartilaginous primary skeleton anlagen grows quickly and is subsequently turned into bone, during prenatal and postnatal growth. This process, referred to as endochondral ossification, is mediated by the activity of chondroclasts, that resorb the cartilage, and osteoblasts, that deposit bone [21]. The second process by which bone modeling occurs is intramembranous ossification, in which bones grow through a mesenchymal template without having to go through the cartilage state first. During this process, condensed mesenchymal cells differentiate directly into osteoblasts at a number of different sites simultaneously and start depositing bone [22]. Long bones grow in both thickness and length. Increase in bone thickness (appositional growth) occurs through the formation of new bone on the outer surfaces of existing bone; increase in bone length (longitudinal growth) is achieved through the activities of the cartilaginous growth plates. Growth plates can be divided into several distinct zones: resting, proliferating, maturing, and terminally differentiated chondrocytes Appositional growth is the result of osteoblast activity on the outer layer of the bone where bone is deposited in successive laminar patterns. This process is coupled with osteoclast activity that causes the resorption of the inner surface of the bone. Medullary bone is a secondary bone tissue that is exclusive to birds [23]. It deposits in spicules in the cavities of long bones and serves as a labile calcium reservoir to support the formation of eggshell in the uterus of the female. The contents of the medullary bone fluctuate with the ovulatory cycle and the position of the egg in the oviduct [24, 25]. When the egg is in the infundibulum, isthmus, or magnum, the bone‐building osteoblasts, under the action of estrogen, actively deposit medullary bone; when the egg transitions to the uterus (eggshell gland), osteoblasts cease depositing bone, while the bone‐resorbing osteoclasts, under the activity of parathyroid hormone, start their bone resorption activity to release calcium. The freed Ca is then deposited on the eggshell, which in turn serves as a reservoir for the developing embryo. The amount of Ca in each eggshell typically represents about 10% of the total body Ca stores in the bird [26]. In avian species that lay a lot of eggs, such as the commercial laying hen, the calcium demand for eggshell formation can get too high and result in the mobilization of calcium from cortical bone. This leads to thinning of the bone and osteoporosis [27]. Osmoregulation refers to the various mechanisms organisms use to regulate the levels of water and electrolytes in their bodies. Unlike in mammals, where osmoregulation is solely regulated by the kidneys, birds have three organs that play a role in osmoregulation: the kidneys, the lower gastrointestinal tract, and salt glands [28]. In this section, only kidney function will be discussed. For more on adaptive strategies for postrenal handling of urine in birds, see the review by Laverty and Skadhauge [29]. Osmoregulation in the avian embryo involves a balance between water loss, due to evaporation though the eggshell, and water gain through increased metabolic activity as development advances. Maintaining a balance between water loss and water gain is crucial for avoiding osmotic stress, dehydration, and embryonic death [30]. The metanephric kidney and the chorioallantoic membrane (CAM) work together to regulate water and electrolyte balance [31]. Early in embryonic development, the CAM is the major regulator of water and ion balance [32]. As development progresses, the mesonephros, which is then replaced by the metanephric kidneys, takes over osmoregulation as well as nitrogen secretion. During this time, nitrogen secretion switches from urea to uric acid [31]. The urinary system in birds consists of paired kidneys and ureters that empty into the urodeum of the cloaca. The role of the kidney is to filter blood, excrete waste products and ions, and reabsorb needed substances like glucose. The avian kidney is divided into three divisions: the anterior, middle, and posterior lobes [33]. Each lobe is a collection of units referred to as lobules. The outer part of the lobule is called the cortex, which constitutes about >75% of the lobule, and the inner part of the lobule is called the medulla, which constitutes <15% of the lobule; the rest is blood vessels. The functional unit in the kidney is a long convoluted tubule called the nephron [34]. Because birds do not have a urinary bladder, the output of the kidneys is emptied into the cloaca. It then moves in a retrograde fashion toward the large intestine where more water, sodium, and potassium are reabsorbed [35, 36]. In birds, the cloaca and lower intestine serve a similar function to that of the distal nephron in mammals, making fine adjustments in water and sodium reabsorption from the urine. Unlike mammals, the ability of birds to produce hyperosmotic urine is limited. Domestic chickens, for instance, can produce urine that is only twice the osmolality of their plasma [37]. This limited ability is attributed to the fact that the majority of the nephrons in their kidney (85%) are reptilian type nephrons, meaning they lack a loop of Henle, which is the part of the nephron that gives mammalian type nephrons the ability to excrete salt. When birds are subjected to extreme dehydration that results in acute osmotic load, the kidneys respond by slowing down their glomerular filtration rate (GFR) [38] and by reducing the number of functioning reptilian type nephrons [34]. When the bird is severely dehydrated, GFR can be reduced as much as 65%. GFR is controlled by the pituitary‐secreted antidiuretic hormone arginine‐vasotocin (AVT). AVT affects the GFR by decreasing the number of filtering loopless nephrons, by constricting the vasculature around the nephrons [34] and epithelium of renal tubules [39]. As in other animals, the respiratory system functions to exchange oxygen (O2) and carbon dioxide (CO2) in the lungs. Oxygenated blood supplies the various tissues and organs with O2 to support metabolic demands including maintenance, growth, and activities such as flight. Carbon dioxide is produced as the result of these metabolic processes and enters the bloodstream. Gases in the blood are expressed in terms of partial pressure (PO2 or PCO2) and in arterial blood as PaO2 and PaCO2. Besides exchange of gases, the respiratory system is involved in acid–base balance, thermoregulation, and water balance [40]. Further detail is available on the avian respiratory system [40, 41]. The function and anatomy of the respiratory system of gamebirds are not much different from other birds [42]. Cycles of inspiration and expiration bring air into the respiratory tract where the gaseous exchange occurs in the paleopulmonic parabronchi of the lung in a humidified environment. Gas exchange does not occur in the air sacs; however, the air sacs act as a bellows to move the air through and out of the respiratory tract. Breathing is an active process as the lung is not expansible and birds do not have a diaphragm. Muscle contraction is needed for both inspiration and expiration to create pressure differences for airflow. Contraction with inspiration moves the sternum forward and down, and the vertebral ribs move to expand the sternal ribs and abdominal cavity, creating negative pressure. During expiration, contraction with a different set of muscles decreases abdominal volume [40, 41]. The pattern of airflow through the respiratory tract means that two cycles of inspiration and expiration need to occur to move an initial volume of air into the tract and then its subsequent exit through the upper part of the tract [41, 43]. With both inspiration and expiration, airflow through the paleopulmonic parabronchi is unidirectional from caudal to cranial. Upon inspiration, airflow is to the cranial and caudal air sacs and air leaves the paleopulmonic parabronchi to the cranial air sacs; with expiration, air leaves the cranial air sacs for exhalation through the trachea and air from the caudal air sacs passes cranially to the parabronchi of the lung. Ventilation changes in response to blood pH or arterial gases (PaO2 and PaCO2) can be species dependent. In response to changes from normal, ventilation frequency and/or tidal volume will adjust. Sensing is done by chemoreceptors with central, arterial and intrapulmonary receptors located in the brain, carotid artery, and lung, respectively. The arterial chemoreceptors detect changes in PaO2, PaCO2, and blood pH. Intrapulmonary receptors are very sensitive to PCO2, assessing on a breath‐by‐breath basis. Overall, the system is more sensitive to changes in CO2 than O2 [44]. Under conditions of increased CO2 in inspired air, frequency and tidal volume increase. When a decrease in PaCO2 or intrapulmonary PCO2 occurs, ventilation frequency is decreased. With hypoxia (low O2), while typically not experienced, ventilation is initially increased, although once PaCO2 starts to decrease, ventilation frequency will also decrease. Shifts in blood pH can have a number of negative effects on the animal’s system. The respiratory system is able to respond quickly to these shifts. By changing ventilation frequency, blood pH can be modified through PaCO2 adjustments [40]. Circulating levels of CO2 are a primary determinant of blood pH. In the blood, CO2 is present in different forms, about 5% as dissolved in arterial blood, combined with hemoglobin, and the majority in the form of bicarbonate ion (HCO3−). To produce bicarbonate, CO2 combines with water to form carbonic acid that dissociates to bicarbonate and hydrogen ions under enzymatic action as demonstrated in the following equation:
5
Avian Physiology
5.1 Muscle Development and Growth
5.1.1 Skeletal Muscle Development and Growth
5.1.2 Embryonic Development of the Skeletal Muscle
5.1.3 Muscle Growth Post Hatch
5.1.4 Maternal Inheritance of Muscle Growth Property
5.2 Bone Development and Growth
5.2.1 Bone Modeling
5.2.2 Long Bone Growth
5.2.3 Medullary Bone
5.3 Osmoregulation
5.3.1 Metanephric Kidney Development and Osmoregulation in the Embryo
5.3.2 The Urinary System in the Adult
5.3.3 Urine Concentration and Response to Dehydration
5.4 Respiratory System
5.4.1 Gaseous Exchange
5.4.2 Ventilation Control
5.4.3 Respiration and Acid–Base Balance
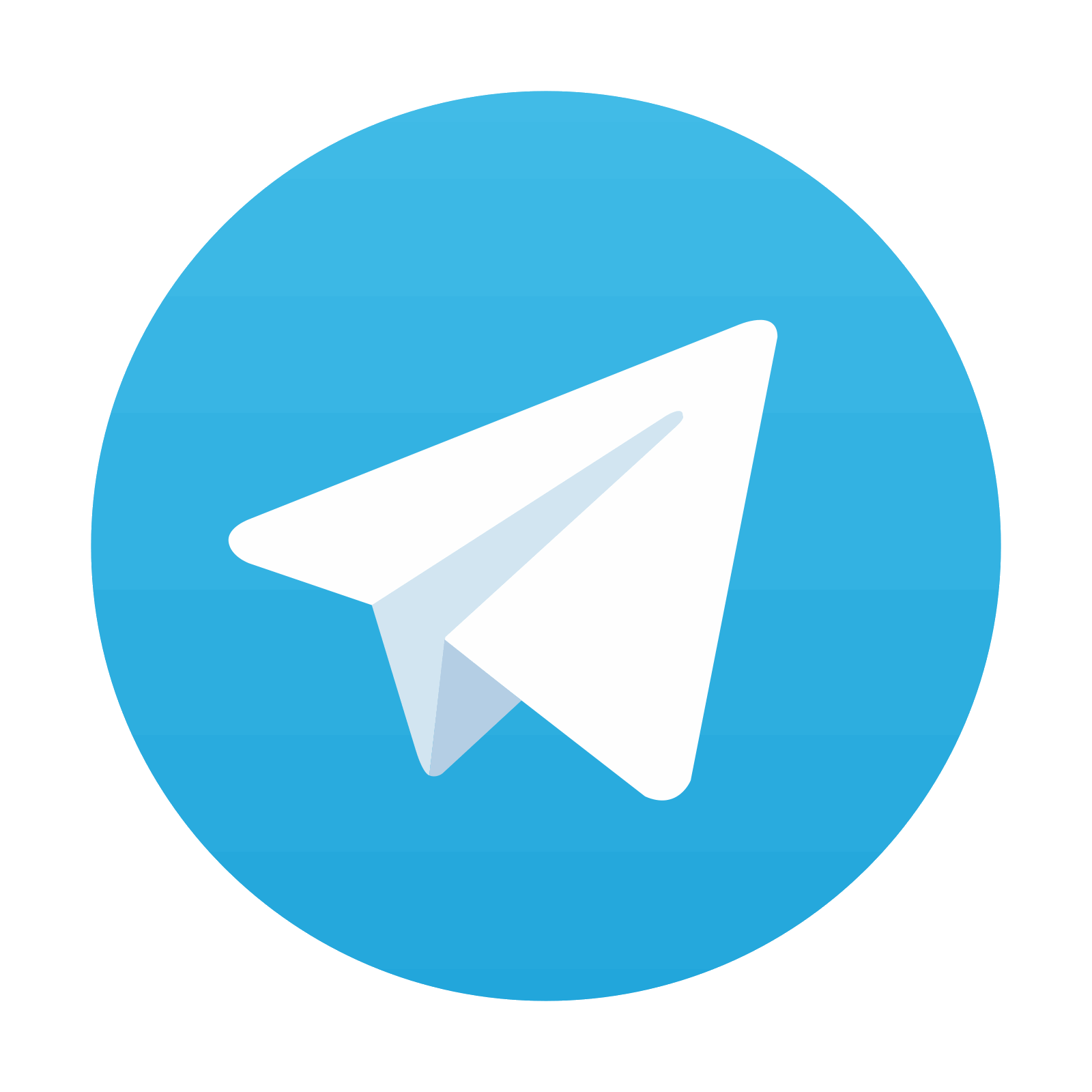
Stay updated, free articles. Join our Telegram channel
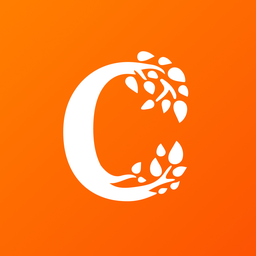
Full access? Get Clinical Tree
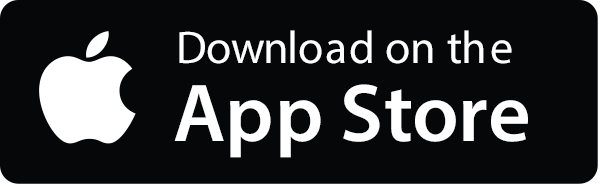
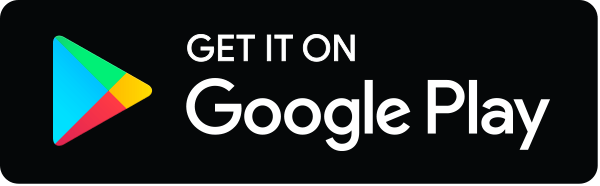