Fig. 1.
Techniques most frequently used to study gliosis in CNS injury. A review of citations in PubMed from January 2008 to March 2010 was conducted using the keywords “gliosis and brain injury” and “gliosis and spinal cord injury” and the techniques used to evaluate gliosis were tallied. The majority of studies in both traumatic brain injury (TBI) and spinal cord injury (SCI) use GFAP-immunoreactivity (GFAP-IR) to indicate gliosis. The evaluation of microglial markers was also termed gliosis. Fewer studies (dark gray portion) used techniques other than GFAP-IR.
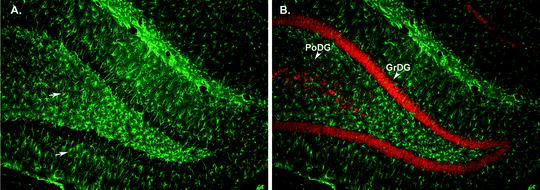
Fig. 2.
GFAP immunohistochemistry in hippocampus of rat brain. (a) Micrograph (×20 magnification) illustrating GFAP-IHC in the dentate gyrus region of the hippocampus from an adult rat. GFAP-immunoreactive astrocytes (arrows, green cells) are clearly visible throughout the hippocampus. (b) Micrograph (×20 magnification) demonstrating labeling of GFAP-immunopositive astrocytes (green) with labeling of NeuN-immunopositive (red) neuronal cell bodies. Note the neuronal cell bodies in the polymorphic layer (PoDG) and in the granular cell layer (GrDG) of the dentate gyrus (arrow heads). Micrographs were provided by E.J. West of the University of California, Davis.
In the adult brain and spinal cord, astrocytes are terminally differentiated and highly complex cells that serve as active partners to neurons in normal CNS function. Indeed, astrocytes are essential in providing energy metabolites to neurons, maintaining extracellular ionic homeostasis, removing extracellular glutamate, regulating blood flow, and are active signaling partners to neurons in synaptic function and plasticity (1–3). Under pathological conditions, such as acute traumatic brain injury (TBI) or spinal cord injury (SCI), ischemia, infection, neurodegeneration, or seizure/epilepsy, astrocytes become reactive. However, the precise definition of reactive astrogliosis remains an area of active scientific debate and ongoing research; accordingly, the reader is directed to an excellent recent review of the issues surrounding defining reactive astrogliosis (2). The definition of reactive astrogliosis proposed in this review contains four critical components. First is that reactive astrogliosis is a spectrum of changes that occurs in response to a graded severities of injury/insult, and secondly that the changes in astrocytes are dependent on the nature and severity of the injury. Additionally, the astrogliotic response is regulated by the context in which it occurs. Lastly, the functional consequences of reactive astrogliosis occur through both gain and loss of astrocyte functions and can have either beneficial or detrimental consequences to surrounding tissue. This definition emphasizes the importance of understanding the attributes and functional consequences of reactive astrogliosis in the context of the CNS insult and underscores the importance of studying reactive astrogliosis in response to different types and severities of CNS insults (2).
1.2 Histological Techniques to Evaluate Reactive Astrogliosis
Although the molecular, biochemical, and functional changes associated with reactive gliosis are not fully elucidated, the morphological changes are better described (4). One morphological hallmark of reactive astrogliosis is the up-regulation of the intermediate filament proteins glial fibrillary acidic protein (GFAP) and vimentin which is often accompanied by a thickening of the main astrocyte processes, or hypertrophy (5, 6). In healthy tissue, GFAP is the main intermediate filament expressed and the levels of vimentin are typically low and depend upon the subpopulation of astrocytes examined (6). However, the expression of both these proteins is significantly increased in reactive astrogliosis albeit heterogeneity and regional differences remain (7, 8). Thus, one of the most commonly used techniques to measure reactive gliosis is to evaluate the expression of GFAP. As demonstrated by a recent review of research studies cited in PubMed from January 2008 until March 2010 obtained by searching the keywords “gliosis and brain injury” and “gliosis and spinal cord injury,” a vast majority of the published work uses evaluation of GFAP expression as the main outcome measure for gliosis (Fig. 1). It is important to note that in both non-reactive and reactive astrocytes, the expression of GFAP protein that can be detected by immunohistochemistry (IHC) is limited to the proximal portions of cell processes which means that the complexity of the fine distal processes and their associated volume cannot be visualized with GFAP-IHC (for review, see ref. (6). In other words, the volume and complexity of each astrocyte is significantly underestimated by the visualization of intermediate filaments. Techniques to determine the expression of intermediate filaments in CNS injury models typically utilize standardized techniques for IHC using commercially available antibodies against GFAP. Table 1 lists sources for the most commonly used GFAP antibodies and detailed protocols for carrying out IHC in brain or spinal cord tissue are described in the Materials, Methods, and Notes sections below.
Table 1
Antibodies commonly used to detect intermediate GFAP in CNS astrocytes
Protein | Antibody type | Vendor |
---|---|---|
GFAP | Rabbit polyclonal Mouse monoclonal Chicken polyclonal Goat polyclonal | Z0334 Dako, Inc., Carpinteria, CA MAB360 Milipore, Inc., Billerica, MA Ab4674 Abcam, Inc., Cambridge, MA SC-6171 Santa Cruz Biotechnology, Inc., Santa Cruz, CA |
It recently has been discovered that in healthy tissue, cortical and hippocampal astrocytes are organized into adjacent, but nonoverlapping domains and that one domain can contact greater than 100,000 synapses in rodent brain and over 1,000,000 in human brain (9, 10). Additionally, recent studies demonstrate that under some conditions of CNS brain injury, this “tiling” of astrocyte processes can be lost in reactive astrogliosis (11). For example, Nedergaard and colleagues recently utilized diolistic labeling of fixed brain tissue and subsequent analysis in reactive astrocytes to demonstrate that seizure/epilepsy caused a loss of astrocytic domain organization. Moreover, they reported that this loss of astrocyte domains was not a universal feature of astrogliosis, as it was not found in a mouse model of neurodegeneration even though extensive reactive astrogliosis was observed (11). Thus, a current hypothesis of the alteration of domain organization in reactive astrogliosis is that in mild-moderate gliosis these distinct domains remain, but in severe gliosis and scar formation, particularly when proliferation is involved, astrocyte processes interdigitate and overlaps resulting in a loss of distinct domains (2). Techniques for diolistic labeling using a Helios Gene Gun System (Bio-Rad, Hercules, CA) to determine the domain organization of astrocytes are discussed in the Materials, Methods, and Notes sections below.
1.3 New Functional or Genomic Techniques to Evaluate Reactive Astrogliosis
Subsequent sections in this chapter provide protocols and tips on conducting histological evaluation of GFAP expression and astrocyte organization as measures of reactive astrogliosis. Although these techniques are among the most prominently used and newly developed techniques to measure reactive astrogliosis, they are certainly not the only methods available. Thus, a brief review of additional selected techniques to evaluate gliosis is provided below.
1.3.1 Novel Functional Techniques
1.
Bioluminescence imaging in brain of Gli-luc transgenic reporter mouse.
The glioma-associated oncogene homologue Gli was originally characterized as a gene up-regulated in gliomas and was later discovered to be an element of the sonic-hedgehog (SHH) signaling pathway. Holland and colleagues genetically engineered a mouse model to evaluate the role of SHH signaling in glioma-induced and non-neoplastic brain injury. Details on the creation and characterization of this mouse model are found in their recently published work (12, 13). Briefly, this mouse model is based on pGL3B/8GliBS-luc plasmid that was upstream of the firefly luciferase gene, and chimeric founder mice were generated by pronuclear microinjection of the linearized Gli-luc construct into fertilized oocytes. The Gli-luc transgenic reporter mouse has been used to evaluate reactive astrogliosis after freeze lesion brain injury (13). In this study, transgenic mice were subjected to brain injury, and euthanized at the appropriate post-injury time point. Immediately prior to euthanasia, mice were given a retro-orbital injection of luciferin. Luciferin–luciferase luminescence was detected in whole mouse brain using an in vivo bioluminescence/fluorescence detection system (Xenogen IVIS-200 Optical Imaging System, Xenogen Corp. Alameda, CA). This system is based on the emission spectrum of luciferase (at 37°C) being mainly above 600 nm and the resultant high efficiency of tissue penetration. Additionally, since no excitation light is required for luciferase bioluminescence, the signal-to-noise is relatively high and not obfuscated by autofluorescence (14). In the use of this mouse model to evaluate reactive gliosis after CNS injury, Holland’s research team reported a bioluminescence ratio of bioluminescence values from the injured to the uninjured cortex. They showed that the SHH pathway is expressed by reactive astrocytes and is associated with proliferation of Olig2+ cells (13). Although this technique requires access to specialized equipment and a transgenic reporter mouse line, it can be used to qualitatively and quantitatively assess reactive astrogliosis in whole brain.
2.
Manganese-enhanced magnetic resonance imaging to detect gliosis.
In experimental studies, manganese chloride (MnCl2) is increasingly being used as a positive contrast agent in manganese-enhanced magnetic resonance imaging (MEMRI) that is sensitive to biological brain processes. Several applications of MEMRI have been recently developed. Since the Mn2+ ion can enter cells via calcium channels, imaging protocols have been developed to examine activation-induced regional brain activity. Also, direct injection of MnCl2 into specific brain regions enables the use of MEMRI for anterograde mapping and this protocol has been used to detail connections of several brain pathways. The third application of MEMRI is as a whole brain contrast agent after peripheral injection as a new method of molecular imaging to characterize anatomical changes in brain regions (15). Recently, an MEMRI protocol using whole brain contrast was developed to evaluate reactive astrocytosis after ischemic brain injury in rats (16). In this report, it was hypothesized that since glutamine synthetase, an enzyme located primarily in astrocyte, requires Mn that reactive astrocytes would accelerate Mn uptake and accumulation that could be detected using Mn-enhanced T1 (longitudinal relaxation time) weighted MRI. They demonstrated that MEMRI reliably detected reactive gliosis and confirmed the MRI findings with corresponding histochemical evaluation of GFAP-immunoreactivity. The MEMRI was particularly reliable in detecting gliosis at 11 and 22 days post-infarct (16). Since Mn, particularly at high concentrations, is toxic to brain tissue, MEMRI is not anticipated to be developed as a clinical imaging technique. However, the experimental studies demonstrate good agreement between the MEMRI signal and GFAP-positive reactive astrocytes after experimental brain injury which suggests that MEMRI could be used as a new experimental technique to measure astrogliosis that potentially could be applied to repeated evaluations or longitudinal studies in living animals.
3.
Electrophysiology evaluation of inwardly rectifying potassium currents Kir4.1 in astrocytes.
As mentioned above, the precise signaling pathways that regulate reactive astrogliosis remain unknown; yet, a growing body of evidence suggests that alteration in potassium (K+) influx in astrocytes is critical. It is well established that the highly negative resting membrane potential and high K+ permeability of astrocytes is maintained by the inwardly rectifying K+ channel Kir4.1 (17, 18). Moreover, Kir4.1 is the channel that supports K+ spatial buffering and homeostasis, both vital astrocyte functions that maintain normal neuronal firing (18). Clues to the role of Kir4.1 in reactive astrogliosis come from the relationship between channel function and cell proliferation/differentiation. Previous work has shown that dividing and immature cells have a relatively positive resting membrane potential that becomes more negative as cells mature and terminally differentiate and this transition is associated with the increased expression of Kir4.1 channels (18). Several lines of evidence substantiate this relationship between Kir4.1 expression and cell division. For example, blockade of the Kir4.1 channel delays differentiation and exit from the cell cycle (19), and glia in Kir4.1 knock-out mice display depolarized resting membrane potentials and immature morphologies (20, 21). Also, malignant human glioma cells maintain a relatively depolarized membrane potential and have mislocalized/nonfunctional Kir4.1 channels, but transfection with functional Kir4.1 channels hyperpolarizes the cell membrane and arrests cell division (22). With regard to injury, in vitro injury to spinal cord astrocytes decreased Kir4.1 activity and induced proliferation (23). TBI was shown to induce abnormal K+ accumulation that was similar to that achieved by blockade of Kir4.1 channels (24). Taken together, these data suggest that mature astrocytes express Kir4.1 which is crucial for K+ spatial buffering, extracellular K+ homeostasis, glutamate uptake, and maintaining astrocyte-neuronal interactions in normal firing. Furthermore, injury decreases Kir4.1 expression and function which shifts cells to an immature state and induces hypertrophy and cell division which are characteristics of reactive astrocytosis.
We recently evaluated the expression and function of Kir4.1 after SCI in rats and found that Kir4.1 protein expression was significantly down-regulated following SCI. To examine whether these changes in Kir4.1 expression are also reflected in the biophysical properties of astrocytes, we performed whole-cell voltage-clamp recording from control and injured animals at the epicenter of the lesion at 7 days post-SCI. Cell type was determined by responses to linear voltage ramps and voltage steps with astrocytes displaying time and voltage sensitive currents, relatively low input resistances (25–300 MΩ), and a lack of spontaneous electrical activity and spiked in responses as previously described (25). Kir4.1 mediated currents in spinal cord astrocytes were isolated and we found that Kir4.1 currents density values fell within a narrow range of −10 to −45 pA/pF in control animals. Surprisingly however, injured animals showed a large degree of scatter in current density values with some cells showing barely recordable currents yet other expressing currents at densities exceeding that of control cells by over fourfold. These data suggest that injured cells do not show a homogenous reduction in Kir4.1. In light of the overall reduction in Kir4.1 protein observed by Western blot which samples the entire affected tissue, it is plausible that many cells in which Kir4.1 expression had been lost are not included in our recordings. Thus to fully understand the complex relationship between Kir4.1 expression, function, and astrocyte pathology after SCI, it is crucial to analyze astrocytes at several locations relative to the lesion and relative to the degree of reactive astrogliosis as indicated by morphological assessments.
1.3.2 Novel Genetic Approaches to Assess Functional Role of Reactive Astrogliosis
1.
Evaluation of transcriptional control of the GFAP gene.
One approach to evaluating the functional consequences of reactive astrogliosis has been to produce GFAP-deficient mice. Although GFAP-null mice appear normal in development, reproduction, and life span, subtle differences between GFAP-knockout and wild type control mice have been observed, including alterations in long-term potentiation and depression, increased susceptibility to autoimmune encephalomyelitis and age-induced deficits in myelination (5, 26). Brenner and colleagues induced acceleration/deceleration TBI using a weight drop device in GFAP-null mice. They reported that moderate weight drop injury (20 g weight from a height of 45 cm or 900 g-cm impact force) caused acute post-injury death or paralysis in all GFAP-null mice as compared to no death or overt deficits in wild type controls. Evaluation of overt pathophysiology in the brain and cervical spinal cord revealed contusion and hemorrhage in the cervical spinal cord of GFAP-null mice but not in wild type controls (27). Interestingly, the spinal cord pathology was observed in the absence of overt brain pathology and was not observed when the mouse head was impacted with the weight but fixed, suggesting that the cervical spinal cord was most sensitive to the acceleration/deceleration forces. An additional line of work by the Brenner research team is exploring the transcriptional regulation of the GFAP gene by evaluation of specific promoter regions in transgenic mice. For example, the gfa2 promoter, which comprises of 2.2 kb of 5′-flanking DNA of the human GFAP gene was joined to an E. coli β-galactosidase reporter gene (lacZ) to evaluate the role of this promoter in development and reactive astrogliosis. They demonstrated up-regulation of the gfa2-lacZ transgene in response to stab wound injury (28). These data suggest that regulation of injury-induced reactive astrogliosis could be achieved by greater understanding of the reactive sites on regulatory elements altered by injury.
2.
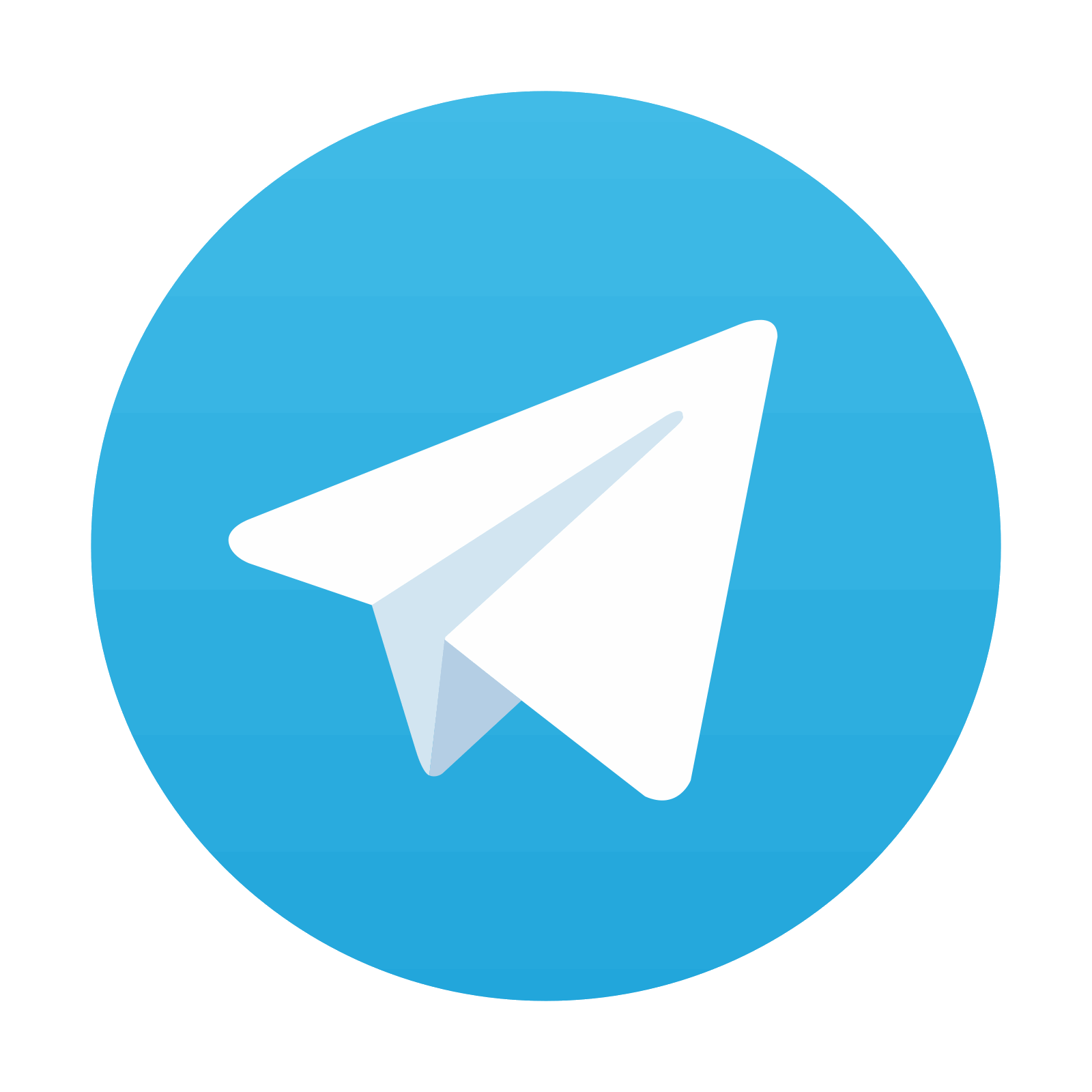
Transgenic mouse model for selective ablation of reactive astrocytes.
In a series of pioneering studies, Sofroniew and colleagues evaluated the effect of selective ablation of proliferating reactive astrocytes after CNS injury (29–31). To do this, they generated a transgenic mouse in which the expression of the enzyme herpes simplex virus-thymidine kinase (HSV-TK) is targeted to astrocytes via the mouse GFAP promoter. Ablation of proliferating cells is achieved by administration of the thymidine analogue and antiviral agent ganciclovir, which inhibits DNA synthesis and kills dividing cells (32). Ganciclovir was administered peripherally by osmotic minipump beginning immediately after the traumatic CNS injury. Using this genetic tool to evaluate the role of proliferating reactive astrocytes after traumatic SCI, they reported that ablation induced failure of blood-brain barrier repair, extensive leukocyte infiltration, and increased tissue disruption, demyelination, cell death, and motor deficits as compared to mice without ablation of reactive astrogliosis (29). Similarly in a model of TBI, moderate controlled cortical impact in HSV-TK mice treated immediately post-injury with ganciclovir induced a significant increase in cell death and inflammation that resulted in an increased loss of cortical tissue as compared to control mice. Interestingly, with severe TBI, substantial cell death and tissue loss was seen in HSV-TK ganciclovir and control mice, but no significant differences between these groups were found. Taken together, these data suggest that ablation of proliferating reactive astrocytes is protective in moderate TBI but has little effect on the large lesion that develops after severe TBI (30). Thus, this mouse model of selective ablation of proliferating reactive astrocytes has great utility in studying the functional role of reactive astrogliosis after CNS injury.
< div class='tao-gold-member'>
Only gold members can continue reading. Log In or Register a > to continue
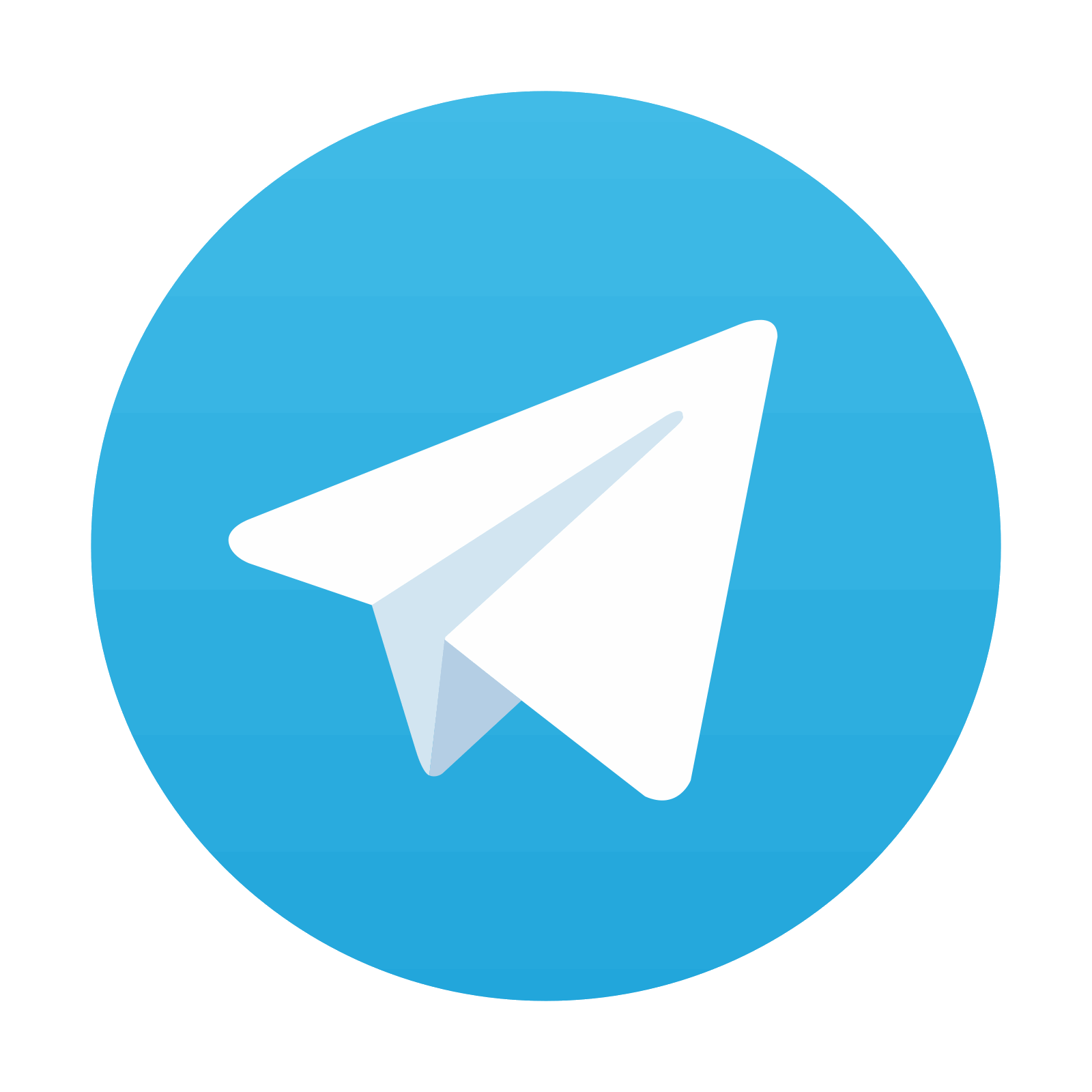
Stay updated, free articles. Join our Telegram channel
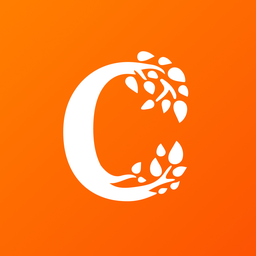
Full access? Get Clinical Tree
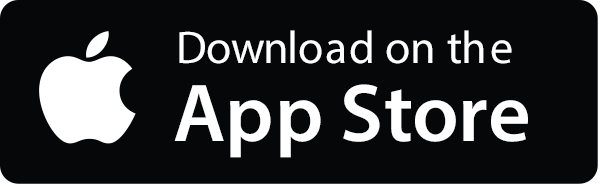
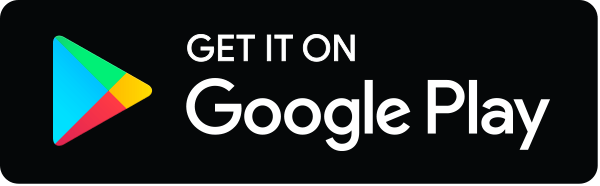
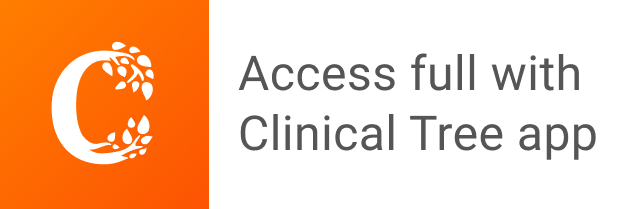