Fig. 1.
Dynamic imaging of BBB permeability in the rat neocortex (a and b). Averaged fluorescent images from the surface of the rat neocortex during tracer injection before (a) and after (b) perfusion with a solution containing high (K+) (30 mM). Clear leakage of the fluorescent dye is seen following treatment suggesting BBB breakdown. (c) Results of image analysis are shown for maximal (max) value in each pixel. Note the robust increase in tracer intensity within the extravascular region following treatment. (d) Intensity-time curves created for each compartment (i.e., arterial, venous, and extravascular) before (black ) and after treatment (red ) using cluster analysis revealed increase in incline and max parameters through the vessels compartments, indicating for increase in flow. In the extravascular compartment, treatment with high (K+) led to increase in max value following by slowing decay of the signal intensity, suggesting for accumulation of the tracer in this compartment after BBB breakdown. (e) Injection of additional tracer with higher molecular weight, FITC-Albumin, and detection of the signal behavior during the post-injection phase (decline phase) results with faster signal-decay of the NF (red ), suggesting for accumulation of the FITC-Albumin in the extravascular elements.
While accumulated data from experimental animals and human patients point to the complexity of BBB breakdown in extent, spatial and temporal domains, and to the key role of BBB breakdown to the pathophysiology of brain damage and repair mechanisms, quantitative and reliable methods for measuring BBB permeability in the living brain still do not exist. Here, we describe a new dynamic imaging method for quantification of local changes in the blood flow and permeability of small pial and surface cortical vessels under physiological and pathological conditions (26). We demonstrate the advantages and disadvantages of the approach and present alternative established methodologies for the identification of BBB breakdown.
2 Dynamic In Vivo Imaging Method for Measuring Regional Blood Flow Changes and BBB Permeability Changes
This method is based on in vivo real-time fluorescence imaging of small cortical vessels followed by quantitative image analysis. The advantages of the method include high spatial and temporal resolution, allowing the study of distinct anatomically and functionally defined vessels (i.e., arterioles and venules) while maintaining a high sensitivity for detecting changes in vessels permeability and blood flow (26). In addition, the method allows following dynamic changes in regional blood flow and vessels permeability in the living brain. Limitations of the method are its invasiveness and its limited use to anesthetized animals. Another disadvantage is that to this end, the method has been tested only for surface vessels. It should be pointed that although surface pial vessels appear to share many morphological and functional features with deeper intracerebral vessels, there are some important differences (e.g., the distribution of the TJs between ECs (27)). While we could never detect any apparent difference in permeability between pial and deep vessels in response to experimental manipulations (using established histological methods for detection leakage of proteins in fixed tissue), the future use of other imaging techniques (e.g., two-photon microscopy, see refs. (5, 28)) may allow the implementation of the new analysis methods to deeper cortical vessels. Figure 1 shows a typical experiment demonstrating the effect of elevated extracellular K+ on vessels’ diameter, regional cerebral blood flow, and BBB permeability.
2.1 Main Considerations
Choosing the Fluorescent tracer. The method is based on fast imaging of signal intensity following the peripheral injection of a fluorescent molecule (tracer). Thus, the injected tracer should be nontoxic and under normal conditions not permeable through the BBB. To allow repeated imaging, the ideal tracer should have a short half life in the circulation, preferentially secreted by the kidneys. We use small molecules, including Lucifer Yellow (LY, FW = 521.58 Da) and Na-Fluorescein (NF, MW = 376 Da)—both showing a relatively short half time (<10 min) within the circulation. In an alternative approach, large molecules with a long serum half life are injected and permeability changes are monitored during treatment (e.g., FITC-labeled albumin, MW = 66 kDa); however, in such experiments repeated injections cannot be performed. The use of tracers with different properties (e.g., molecular weight, charge) allows a more detailed characterization of permeability changes to specific agents, including brain to blood clearance under healthy and pathological conditions (see below).
2.2 Materials and Instruments
2.2.1 Solutions
1.
Anesthesia: a mixture of ketamine (100 mg/ml, 0.08 ml/100 g, Ketaset®) and xylazine (20 mg/ml, 0.06 ml/100 g, VMD). Some reports suggest that isoflurane may be a potent BBB opener and thus should probably be avoided (29).
2.
Artificial cerebrospinal fluid (ACSF): 129 mM NaCl, 21 mM NaHCO3, 1.25 mM NaH2PO4, 1.8 mM MgSO4, 1.6 mM CaCl2, 3 mM KCl, and 10 mM glucose. The ACSF is equilibrated with a gas mixture comprising 95% O2 and 5% CO2 (pH 7.4). All Chemicals are purchased from Sigma-Aldrich.
3.
Elevated K+ solution: For experiments with elevated K+ (e.g., to imitate some aspects of red blood cells hemolysis following subarachnoid hemorrhage), KCl was added to the ACSF in exchange with NaCl to maintain osmolarity within the physiological range.
4.
Sodium fluorescein (MW = 376 Da, 1 mg/ml, Novartis), in saline.
5.
FITC-Albumin (MW = 66 kDa, 2 mg/ml, Sigma-Aldrich), in saline.
6.
Bone cement (UNIFAST Trad, GC AMERICA INC).
2.2.2 Instruments
Surgical Procedure
1.
A stereotactic frame
2.
A florescence stereomicroscope (Zeiss, SteReO Lumar V12)
3.
Heating pad for monitoring body temperature
4.
A driller (DREMEL)
5.
Perfusion pump or an automatic injector
Imaging
1.
EMCCD camera (Andor Technology, DL-658 M-TIL)
2.
Computer monitor
2.2.3 Methods
Animal Preparation
1.
Adult SD rats (weighing 200–300 g) are deeply anesthetized by intraperitoneal (IP) injection of ketamine and xylazine mixture.
2.
The tail vein is catheterized with a sterile canula (BD Neoflon, 24 G).
3.
The animal is placed in a stereotactic frame under the microscope and body temperature is continuously monitored and maintained at 37.0 ± 0.5°C.
4.
A bone window is drilled over the motor-somatosensory cortex (4 mm caudal, 2 mm frontal, 5 mm lateral to bregma) of one hemisphere.
5.
An inflow and outflow tubes are inserted under the bone cement rim (created around the bone window), allow continuously superfusion of the brain cortex with ACSF.
6.
The dura is carefully opened.
BBB Opening
As positive control, potent BBB opening can be achieved using a direct perfusion of the cortical surface with ACSF containing 1–2 mM sodium deoxycholate (Sigma-Aldrich, see refs. (26, 30, 31)). Below, we show the effect of high (K+) solution to imitate the effect of delayed hemolysis of blood products within the subarachnoid space as in the case of intracerebral hemorrhage (see refs. (32, 33)).
Real-Time Fluorescence Imaging
1.
Baseline signal images are obtained for 3–5 s, followed by intravenously injection of BBB non-permeable fluorescent dye.
2.
Full resolution (658 × 496) images of cortical surface vessels are obtained at 20–30 images/s before, during, and after injection of the tracer (total of ca. 25 s).
3.
Additional florescence images can be taken for longer periods at a lower sampling rate (1 image/s) to follow late changes in tracer behavior.
4.
Depending on the tracer half life in blood, injections can be repeated.
5.
To compare vessels permeability to different molecules, animal can also be injected with other BBB non-permeable tracer (see Fig. 1e).
6.
Image analysis is performed off line.
Image Analysis
For detailed description of the analysis methods, see ref. (26) and see Fig. 1.
1.
Preprocessing can include image resizing (to reduce memory usage and computation time) using 2D bicubic interpolation (34) and subpixel image registration to overcome artifacts due to small movements. This is performed by applying a single step discrete Fourier transform (35) to each image. To increase the signal-to-noise ratio, each frame is registered according to a moving average of several preceding images.
2.
Signal intensity changes over time and space are then analyzed.
3.
Physiologically measured parameters: baseline, time to incline, incline (averaged slope), time to maximal intensity, maximal intensity, and decline (averaged slope). Maps are performed for the parameter of interest (e.g., Fig. 1c).
4.
Cluster analysis is performed on the measured parameters to separate between compartments with different signal kinetics—usually allowing a good separation of arterioles, venous, and extravascular tissue. To overcome small changes in signal intensity in the extravascular compartment under normal conditions (probably due to nonspecific effect from underlying vessels), a correction to a “near zero” change based on the intravascular signal is optional.
5.
Signal intensity time onset, slope, maximal intensity, and duration reflect the flow of the tracer within the compartment are measured and followed during the experiment (Fig. 1d).
3 Detection of Protein Leakage into the Brain
3.1 Morphological Examination of Evans Blue-Albumin Complex Extravasation into the Brain
Evans Blue (tetrasodium diazo salt, EB)—is a small, BBB-permeable molecule (MW = 960.81) which rapidly and selectively binds to albumin (MW = ∼66 kDa) when injected into a peripheral vein, therefore confers to its properties of a protein tracer (36). It has been thus traditionally used as a reliable tracer to detect significant BBB breakdown ((37–39) and Fig. 2a). In addition, the complex EB-albumin exhibits a red-orange fluorescence and thus can be detected within the CNS using a standard fluorescent microscope and analysis techniques ((31) and Fig. 2b–d). Traditionally, measuring leakage of EB-albumin complex is done in the “steady state” condition on tissue removed from the experimental animal. Although EB binds rapidly and stably to the protein and the amount of free dye in serum is usually negligible, it is important to note that EB injection into the brains’ arterial circulation (or left heart) may result in overestimation of BBB permeability due to leakage of nonbound molecules. Here, we present two alternative methods to measure EB-albumin leakage across the BBB. We describe a semiquantitative method to assess microscopically the extravasation of the EB to the parenchyma.
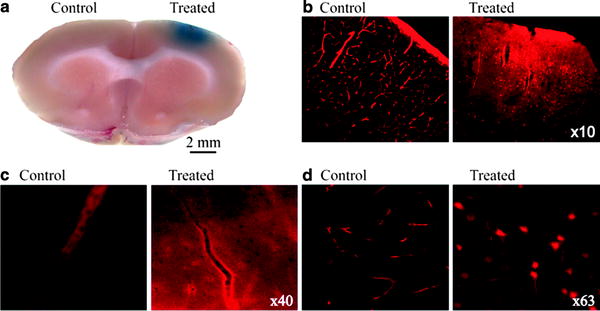
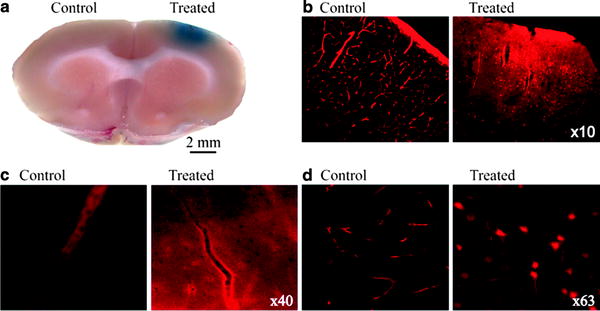
Fig. 2.
Detection of EB-albumin complex within the brain: Rats are injected with the albumin-binding dye Evans blue, perfused with PFA; the brain is then sliced to ca. 500 μm thin sections (a). The treated region of the brain is showing BBB breakdown (blue). Fluorescence histological sections show albumin-EB complex in red ; in the healthy cortex fluorescent staining is limited to blood vessels (b–d control). In brain regions where the BBB is compromised clear extravascular staining can be seen, including uptake by cellular elements (b–d, treated.).
3.1.1 Solutions
1.
Evans-blue (EB) (2% in saline)
2.
Paraformaldehyde (4% in PBS)
3.1.2 Instruments
1.
Fluorescence microscope (Zeiss, Axioskop 2 plus)
2.
Camera (e.g., Cool Snap, Photometrics)
3.
Vibrotome
3.1.3 Method
1.
Animals are injected intravenously (2.4 ml/kg) or intraperitoneally (5 ml) with EB.
2.
At a fixed time after injection (>30 min is recommended if EB is injected intravenously or >60 min for intraperitoneally) rats are transcardially perfused with 4% paraformaldehyde fixation solution.
3.
The brain is quickly removed and sliced (ca. 500 μm thick) using a vibrotome.
4.
Sections are incubated in 4% paraformaldehyde solution for 24 h, followed by incubation in store-solution (PFA 0.02% in PBS). Thin slices (∼10–40 μm) are prepared and assessed under fluorescence microscope (562 nm) for extravascular leakage of the fluorescence injected dye.
5.
Tissue concentrations of the fluorescent albumin-EB complex are measured by microscopically reading color intensity in low-power field cortical imaging (10–20×) using home-made Mathlab Scripts.
3.2 Spectrometric Measurements of Evans Blue-Albumin Complex in Brain Homogenates
In this method, the fluorescent intensity of the EB-albumin complex is measured in a tissue homogenate following a peripheral injection of EB (as in Sect. 2.1).
3.2.1 Solutions
1.
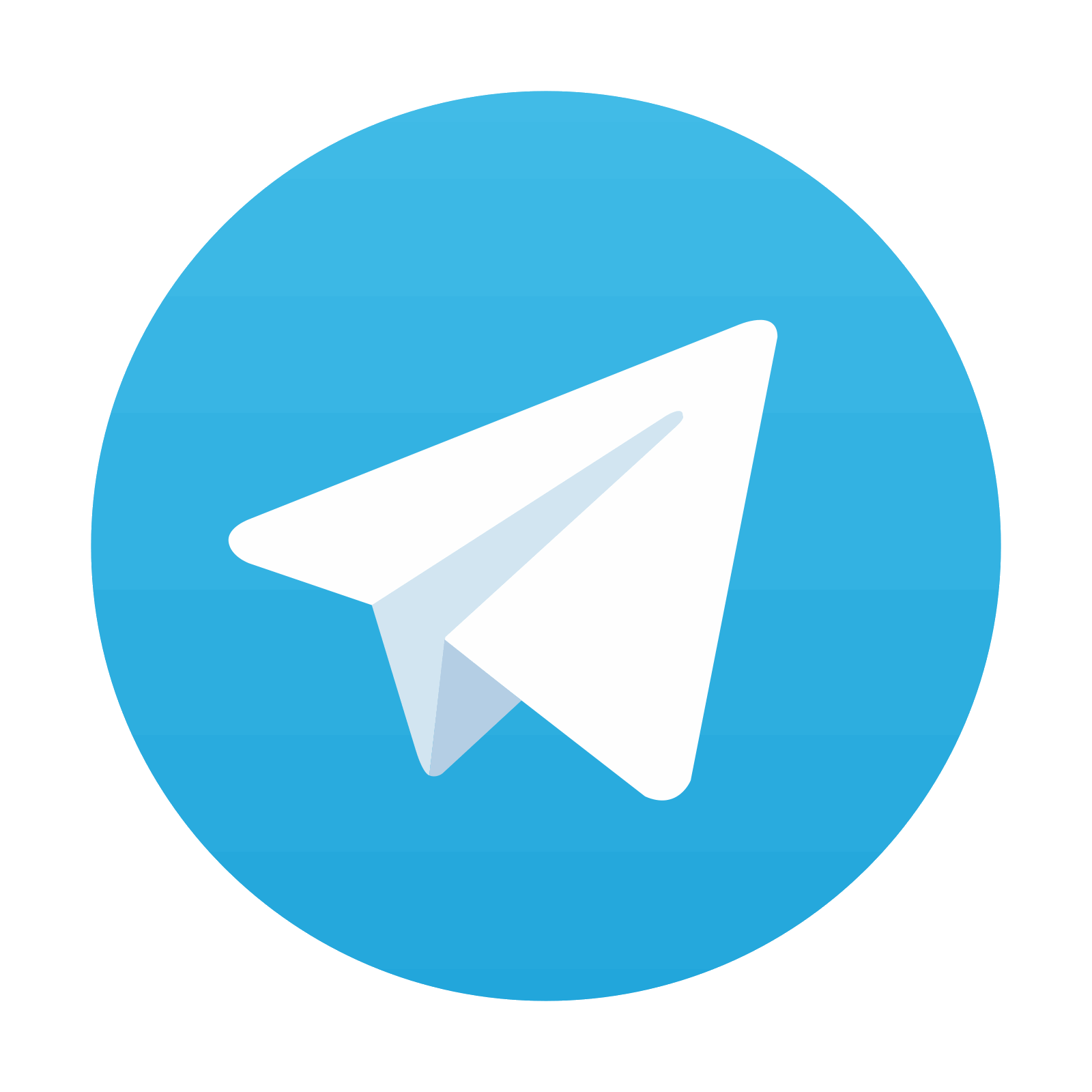
Evans-blue (EB) (2% in saline)
< div class='tao-gold-member'>
Only gold members can continue reading. Log In or Register a > to continue
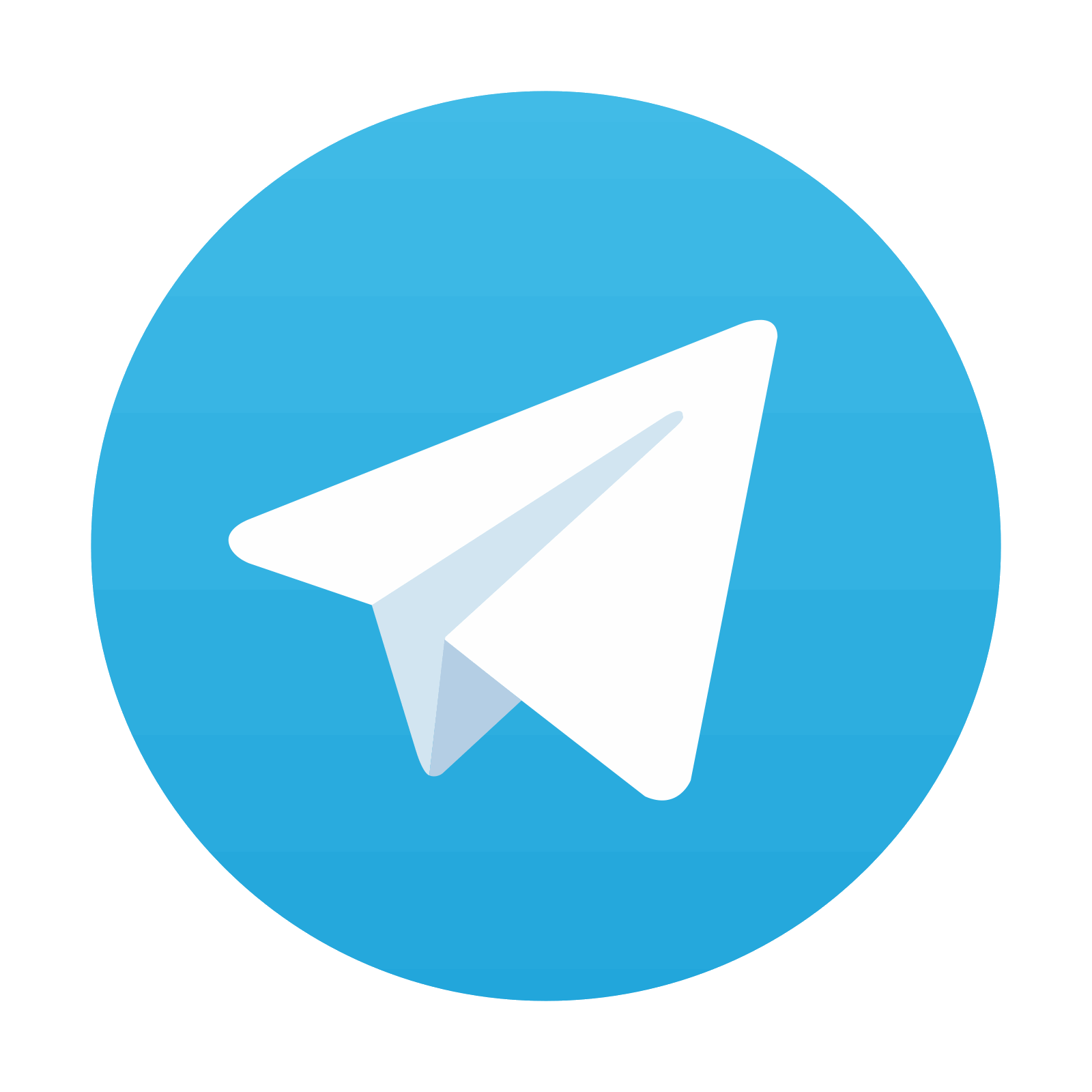
Stay updated, free articles. Join our Telegram channel
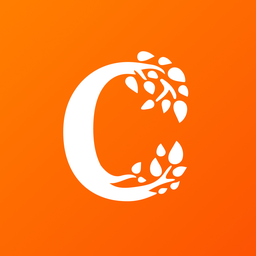
Full access? Get Clinical Tree
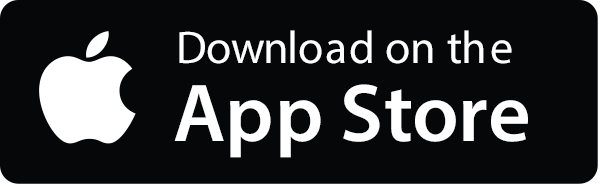
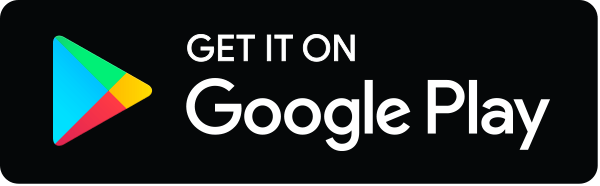
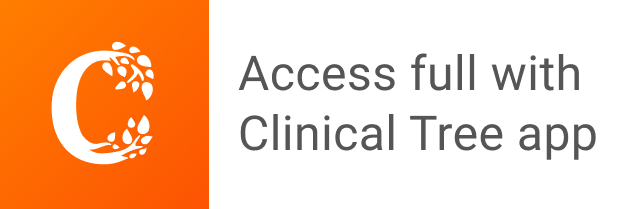