12 Applied Andrology in Cattle (Bos taurus) Leonardo F.C. Brito* Application of knowledge on bovine andrology can increase reproductive efficiency, which is an imperative for the cattle sector sustainability in a world with increasing protein demand and ever more scarce resources. Within this context, the objective of this chapter is to provide a summary of the available literature to aid dedicated professionals in their quest to feed the world. The process of sexual development in bulls involves complex maturation mechanisms of the hypothalamic-pituitary-testicular axis. Sexual development can be divided into three periods according to changes in gonadotrophins and testosterone concentrations, namely the infantile, prepubertal and pubertal periods. The infantile period is characterized by low gona dotrophin and testosterone secretion and extends from birth to approximately 2 months of age. A transient increase in gonadotrophin secretion occurs from approximately 2 to 6 months of age; this so-called ‘early gonadotrophin rise’ characterizes the prepubertal period, during which testosterone concentrations also begin to rise. The pubertal period corresponds to the period of accelerated reproductive development that occurs after 6 months of age and until puberty. During this period, gonadotrophin secretion decreases, whereas testosterone secretion continues to increase (Rawlings et al., 1978; Lacroix and Pelletier, 1979; McCarthy et al., 1979a,b; Amann and Walker, 1983; Amann et al., 1986). Although the timing of sexual development is determined primarily by the hypothalamus and gonadotrophin-releasing hormone (GnRH) secretion, the mechanisms regulating GnRH secretion during sexual development in bulls are poorly understood. Gonadotrophin concentrations during the infantile period are low mainly due to reduced GnRH secretion. Maturation changes within the hypothalamus increase the pulse secretion of GnRH, driving the transition from the infantile to the prepubertal period of development through increased secretion of gonadotrophins. Increased GnRH secretion is dependent on either the development of central stimulatory inputs or the removal of inhibitory inputs. The weight of the hypothalamus and its GnRH content do not increase during the infantile period, but hypothalamic concentrations of oestradiol receptors decrease after 1 month of age, leading to suggestions that reduced sensitivity to sex steroids could be involved in the augmented GnRH secretion in bulls (Amann et al., 1986). The direct evaluation of blood samples from the hypophyseal portal system demonstrated that GnRH pulsatile secretion increased linearly from 2 weeks (3.5 pulses/10 h) to 12 weeks of age (8.9 pulses/10 h) in bull calves. Although GnRH secretion into hypophyseal portal blood was detected at 2 weeks, pulsatile LH secretion was not detected in jugular blood samples before 8 weeks of age. In addition, GnRH pulses were not necessarily accompanied by LH secretion until 8–12 weeks of age, when all GnRH pulses resulted in LH pulses. The increase of pulsatile GnRH release from 2 to 8 weeks of age without a concomitant increase in LH secretion may represent a reduced ability of the pituitary gland to respond to stimulus by GnRH (Rodriguez and Wise, 1989). The period in which GnRH pulses did not stimulate LH secretion corresponded to the period during which there was an increase in pituitary weight, GnRH receptor concentration and LH content (Amann et al., 1986). Moreover, frequent GnRH treatments during the infantile period in calves increased pituitary mRNA for the LH beta subunit (LH-β), LH content and GnRH receptors, with resulting increases in LH pulse frequency and mean concentrations (Rodriguez and Wise, 1991), indicating that increased GnRH pulse frequency results in increased pituitary sensitivity to GnRH. With time, the increased GnRH secretion results in the increased LH pulse frequency observed during the prepubertal period. During the early gonadotrophin rise that is characteristic of the prepubertal period, there is a transient increase in LH and follicle-stimulating hormone (FSH) concentrations from approximately 2 to 6 months of age (Figs 12.1 and 12.2). Concentrations decrease thereafter and remain at levels only slightly greater than those observed during the infantile period. The main factor responsible for increased gonadotrophin concentrations is the increase in GnRH pulse secretion, as demonstrated by a dramatic increase in LH pulse frequency. The number of LH pulses increased from less than one a day at 1 month of age to approximately 12/day (≥1 pulse/2 h) at approximately 4 months of age. Changes in pulse amplitude during this period were not consistent among reports; amplitude was either reduced, unchanged or augmented. Pulsatile discharges of FSH have been observed in bulls, but are much less evident than those of LH (Amann and Walker, 1983; Amann et al., 1986; Evans et al., 1995, 1996; Aravindakshan et al., 2000). LH binding sites in testicular interstitial tissue were present in bulls at birth and at 4 months of age, and pulsatile LH secretion is an essential requirement for Leydig cell proliferation and differentiation, and for the maintenance of fully differentiated structure and function (Schanbacher, 1979). Hypophysectomy, the suppression of gonadotrophins by steroid administration, or neutralization of GnRH/LH by specific antibodies, caused Leydig cell atrophy and loss of cellular volume, reduction in the number of LH receptors and steroidogenic enzyme activity, and a decrease in the ability to secrete testosterone in response to LH. The characteristic pulsatile nature of LH secretion is important for testosterone production, because continuous exposure of Leydig cells to LH resulted in reduced steroidogenic responsiveness owing to the downregulation of LH receptors (Saez, 1994). The initiation of Leydig cell steroidogenesis is characterized by increased androstenedione secretion, which decreases as the cells complete maturation and begin secreting testosterone. During the first 3 to 4 months of age, testosterone concentrations are low and secretion does not necessarily accompany LH pulses. After this age, LH pulses are followed by testosterone pulses and mean testosterone concentrations began to increase (Fig. 12.1). Various studies have shown that the number of testosterone pulses increased from 0.3–2.3 pulses/24 h at 1–4 months of age to 9–7.5 pulses/24 h at 5 months of age (Rawlings et al., 1972, 1978; Lacroix and Pelletier, 1979; McCarthy et al., 1979b). FSH binding sites in seminiferous tubules were detected in bull calves at birth and at 4 months of age, and increased FSH concentrations stimulated the proliferation of undifferentiated Sertoli cells and gonocytes (Schanbacher, 1979). While there is considerable evidence that FSH is essential for normal Sertoli cell function, the period of Sertoli cell differentiation coincides with the initiation of testosterone secretion by the Leydig cells, indicating that testosterone may be involved in promoting the maturation of undifferentiated Sertoli cells. Maturation of Sertoli cells and increased testosterone secretion are probably also involved in the differentiation of gonocytes into spermatogonia. The end of the prepubertal period is marked by completion of Sertoli cell differentiation, with the establishment of the blood–testis barrier, formation of the tubular lumen and initiation of germ cell meiosis (Amann, 1983). In Holstein calves, circulating inhibin concentrations either increased around 3 months of age before decreasing, or decreased continuously after birth until 6.5–8 months of age (MacDonald et al., 1991; Matsuzaki et al., 2001). The crucial role of the early gonadotrophin rise (especially the LH secretion pattern) in regulating sexual development in bulls has been demonstrated in several studies, using a variety of approaches. Prolonged treatment with a GnRH agonist in calves from 1.5 to 3.5 months of age decreased LH and FSH pulse frequency, pulse amplitude and mean concentrations at 3 months of age, delayed the peak mean LH concentration from 5 to 6 months of age, and reduced FSH and testosterone concentrations from 3.5 to 4.5 months of age. These hormonal alterations were associated with delayed puberty and reduced paired testes weight and number of germ cells in tubular cross sections at 11.5 months of age. Conversely, treatment with GnRH every 2 h to mimic pulsatile secretion from 1 to 1.5–2 months of age increased LH pulse frequency and mean concentration during the treatment period, and also resulted in greater scrotal circumference (SC), paired testes weight, seminiferous tubule diameter, and number of germ and Sertoli cells in tubular cross sections at 12 months of age (Chandolia et al., 1997a,b; Madgwick et al., 2008). The LH secretion pattern during the prepubertal period is associated with age at puberty in bulls raised in contemporary groups, suggesting that this is the physiological mechanism by which genetics affects sexual development. Studies have shown that LH pulse frequency was greater at around 2.5 to 5 months of age and that mean LH concentrations increased earlier and reached greater maximum levels in early- than in late-maturing Hereford bulls (age at puberty 9.5 and 11 months, respectively) (Evans et al., 1995; Aravindakshan et al., 2000). Moreover, other studies have indicated that the effects of nutrition on sexual development are mediated through effects on LH secretion patterns (Brito et al., 2007b,c,d). Reduced gonadotrophin secretion marks the end of the prepubertal period and the beginning of the pubertal period. The rapidly increasing testosterone secretion and, possibly, increased hypothalamic sensitivity to negative feedback from androgens are probably responsible for the decrease in LH secretion, whereas inhibin produced by Sertoli cells may act on the gonadotrophs to limit FSH secretion, because immunization with inhibin antiserum resulted in a marked increase in FSH concentrations in prepubertal bulls (Kaneko et al., 1993; Rawlings and Evans, 1995). Testosterone pulse frequency did not increase after the peri-pubertal period and remained at approximately 4.5 to 6.8 pulses/24 h from 6 to 10 months of age. However, pulse amplitude increased during the pubertal period, with consequent increase in testosterone mean concentrations until approximately 12 months of age. Elevated testosterone secretion is essential for increasing the efficiency of spermatogenesis that eventually leads to the appearance of sperm in the ejaculate (Rawlings et al., 1972, 1978; McCarthy et al., 1979a,b; Rodriguez and Wise, 1991). The mechanisms controlling reproduction and energy balance are intrinsically related and have evolved to confer reproductive advantages and guarantee the survival of species. The neural apparatus, which is designed to gauge metabolic rate and energy balance, has been denoted the body ‘metabolic sensor’. This sensor translates signals provided by circulating (peripheral) concentrations of specific hormones into neuronal signals that ultimately regulate the GnRH pulse generator and control the reproductive process. Metabolic indicator hormones may serve as signs to the hypothalamic-pituitary-gonadal axis and affect sexual development. The patterns of some of these hormones have been studied in growing beef bulls (Fig. 12.1). In contrast to those species in which circulating concentrations of growth hormone (GH) continue to increase until after puberty, GH concentrations decreased during the pubertal period in bulls (Brito et al., 2007a,d). Differences in the stage of body development at which each species attains puberty are likely to be responsible for the different GH profiles among species. Accordingly, the GH profile in bulls seems to indicate that a relatively advanced stage of body development must be attained before the gonads produce sperm efficiently. The differences in GH secretion among species may be due to the regulatory role of steroids on GH secretion. In some other species, steroids stimulate GH secretion, but GH concentrations did not differ between intact bulls and castrated steers (Lee et al., 1991). Furthermore, decreasing GH concentrations during the sexual development of bulls are observed along with increasing testosterone concentrations, indicating that steroids do not have a positive feedback on GH secretion in bulls, as they do other species (Brito et al., 2007a,d). Circulating concentrations of insulin-like growth factor I (IGF-I) in calves increased continuously and only reached a plateau (or decreased slightly) after sexual development was mostly completed after 12–14 months of age; increasing circulating concentrations of IGF-binding protein 3 and decreasing concentrations of IGF-binding protein 2 were also observed during sexual development (Renaville et al., 1993, 1996, 2000; Brito et al., 2007a,b,c,d). The concomitant decrease in circulating GH concentrations and increase in IGF-I concentrations during sexual development in bulls indicates that there are either drastic changes in liver sensitivity to GH or that other sources are responsible for IGF-I production. A possible IGF-I source might be the testes, as Leydig cells are capable of secreting this hormone in other species. Observations that intact bulls tended to have greater IGF-I concentrations than castrated steers at 12 months of age further support the hypothesis that the testes might contribute substantially to circulating IGF-I concentrations during the peri-pubertal and pubertal periods in bulls (Lee et al., 1991). Close temporal associations observed in a series of nutrition studies strongly suggest that circulating IGF-I might be involved in regulating the GnRH pulse generator and the magnitude and the duration of the early gonadotrophin rise in beef bulls (Brito et al., 2007b,c,d). A possible effect of IGF-I on testicular steroidogenesis in bulls has also been suggested. Leydig and Sertoli cells both produce IGF-I, indicating the existence of paracrine/autocrine mechanisms of testicular regulation involving IGF-I (Bellve and Zheng, 1989; SpiteriGrech and Nieschlag, 1992). It is assumed that most of the IGF-I in the testes is produced locally, and that circulating IGF-I may play a secondary role in regulating testicular development and function. However, the temporal patterns and strong associations among circulating IGF-I concentrations, testicular size and testosterone secretion observed in bulls receiving different nutrition argue for a primary role for this hormone (Brito et al., 2007b,c,d). This primary role of increased circulating IGF-I during the pubertal period may be to promote the increase in testosterone concentrations by regulating Leydig cell multiplication, differentiation and maturation. Because testosterone has been shown to upregulate IGF-I production and IGF-I receptor expression by Leydig and Sertoli cells (Cailleau et al., 1990), the establishment of a positive feedback loop between IGF-I secretion and testosterone production may be important for sexual development. Circulating leptin and insulin concentrations also increased during the pubertal period in bulls. Notwithstanding, developmental and nutritional differences in LH pulse frequency were not related to differences in leptin or insulin concentrations in beef bulls (Brito et al., 2007b,d). Other studies have also demonstrated that leptin did not stimulate in vitro GnRH secretion from hypothalamic explants or gonadotrophin secretion from adenohypophyseal cells collected from bulls and steers maintained at an adequate level of nutrition (Amstalden et al., 2005). These results indicate that the role of these hormones in regulating GnRH secretion, if any, might be purely permissive in bulls. The testicular growth curve in bulls is sigmoidal with an initial period of little growth followed by a rapid growth phase and then by a plateau. Figure 12.3 illustrates the changes in testicular length and width, as well as in SC in young beef (Angus and Angus cross) bulls on adequate nutrition. Figure 12.4 shows regression curves for SC and paired testes weight (PTW) in dairy (Holstein and Jersey) bulls. Although the overall pattern of testicular growth is similar in all breeds, the characteristics of the growth curve are greatly affected by genetics. In general, the rapid growth phase is shorter and testicular growth plateaus sooner in bulls from breeds that mature faster (reach puberty earlier) than in bulls from late-maturing breeds, resulting in marked differences in the slope of the curve. In addition, the asymptotic value of the curve, i.e. adult testicular size, also differs considerably among breeds (Coulter and Keller, 1982; Coulter et al., 1987b; Gregory et al., 1991; Pratt et al., 1991; Johnson et al., 1995; Bell et al., 1996; Lunstra and Cundiff, 2003). These same differences can be observed within breed between early- and late-maturing bulls, which emphasizes the effects of genetics on bull testicular growth (Evans et al., 1995). Testicular growth is accompanied by marked histological changes. The testicular intertubular cell population is composed of mesenchymal-like cells, fibroblasts, Leydig cells, peri-tubular cells and mononuclear cells. At around 1 to 2 months of age, mesenchymal-like cells made up the majority of the cells in the testicular interstitial tissue. These pluripotent cells proliferated by frequent mitoses and were the precursors of Leydig cells, contractile peri-tubular cells and fibroblasts. Approximately 20–30% of all intertubular cells at all ages were mononuclear cells, including lymphocytes, plasma cells, monocytes, macrophages and light inter-calated cells (monocyte-derived, Leydig cell-associated typical cells of the bovine testis). The thickness of the tubular basal lamina was approximately 3 μm at 4 months of age, but decreased continuously to 1.2 μm at 5 months of age. Mesenchymal-like cells transformed into peri-tubular cells with elongated nuclei at 4 months of age, while these, in turn, transformed into contractile myofibroblasts at 6 months of age (Wrobel et al., 1988). Leydig cells are present in the testes from birth to adulthood. At approximately 1 month of age, most (70%) intertubular cells were mesenchymal-like cells with a high mitotic rate. Typical Leydig cells constituted about 6% of all intertubular cells and a number of these cells were found in an advanced degenerative state, probably as remnants of the fetal Leydig cell population. Degenerating fetal and newly formed Leydig cells coexisted until 2 months of age, but only Leydig cells formed postnatally were observed thereafter. At 2 months of age, Leydig cells accounted for approximately 20% of all intertubular cells and comprised 10% of the total testicular volume. At approximately 4 months of age, the mesenchymal-like cells ceased proliferation and transformed into contractile peri-tubular cells or Leydig cells, thus decreasing the proportion of mesenchymal-like cells to approximately 20%. At 5 months of age, undifferentiated mesenchymal-like cells were rare and the Leydig cell population increased by mitotic proliferation. Newly differentiating, intact and degenerating Leydig cells were observed in close proximity from 4 to 7 months of age. The Leydig cell population found in the adult bull was present at approximately 7 months of age, as mitosis after this age was rare (Wrobel, 1990). Leydig cell mass increased from 0.15 g/testis at 1 month to 5.8 g/testis at 7–8 months of age. After this, Leydig cell mass increased slowly but continuously to reach about 10 g in the young adult testis at 24 months of age. Nuclear and whole Leydig cell volumes increased from 1 to 4 months of age, but then remained unchanged up to 12 months of age. However, from approximately 12 to 24 months of age there was a considerable increase in nuclear and whole Leydig cell volumes. Leydig cell numbers per testis increased from 1 to 7 months of age (0.42 to 6 billion, respectively) and remained unchanged after that. Therefore, the increase in Leydig cell mass after 7 months of age was a result of hypertrophy and not hyperplasia. Leydig cell mitochondrial mass increased from 1 to 4 months of age and remained relatively constant up to 10 months of age, but then more than doubled from that age until approximately 24 months of age (Wrobel, 1990). Functional maturation of Leydig cells was observed to involve the expression of steroidogenic enzymes and the production of testosterone, although the age-dependent expression of steroidogenic enzymes did not parallel changes in the numbers of Leydig cells in the testis (Waites et al., 1985). At approximately 1 month of age, undifferentiated Sertoli cells were characterized by round or oval nuclei with numerous flakes of heterochromatin dispersed throughout the nucleoplasm. Lateral cell membranes of neighbouring presumptive Sertoli cells contained few interdigitations and no special junctional complexes. Between 4 and 5 months of age, opposing cell membranes of adjacent undifferentiated Sertoli cells started to develop extended junctional complexes above the spermatogonia and in the basal portion of the tubules. The nuclei of these cells became more elongated and irregular, a vacuolar nucleolus characteristic of the mature cell developed, and the clumps of heterochromatin present at younger ages disappeared. Most Sertoli cells had completed their morphological differentiation and attained adult structure by 6 to 7 months of age. Junctional complexes consisting of many serially arranged points or lines of fusion involving neighbouring Sertoli cell membranes could be observed. These junctions formed a functional blood–testis barrier and divided the tubular epithelium into a basal compartment containing spermatogonia and an adluminal compartment (connected to the lumen) containing germ cells at later stages of spermatogenesis (Abdel-Raouf, 1960; Sinowatz and Amselgruber, 1986; Wrobel, 2000). In Holstein bulls, the number of adult-type Sertoli cells increased dramatically from 202 to 8862 million cells/testis between 5 and 8 months of age (Curtis and Amann, 1981). Intense germ cell proliferation occurred from 50 to 80 days post conception, but the germ cells entered a prolonged G1- or G0-phase and no mitotic activity was observed until after birth in bulls. At birth, the germ cell population was composed solely of gonocytes (also referred to as prespermatogonia or prepubertal spermatogonia). Gonocytes were usually centrally located and had a large nucleus (~12 μm in diameter) with a well-developed nucleolus (Fig. 12.5). Germ cell proliferation slowly resumed between 1 and 2 months of age, when seminiferous tubule diameter was approximately 50–80 μm. Gonocytes were gradually displaced to a position close to the basal lamina and divided by mitosis, originating A-spermatogonia. Differentiation and degeneration resulted in the complete disappearance of gonocytes from the seminiferous tubules by 5 months of age. Germ cell proliferation reached a maximum between 4 and 8 months of age (tubule diameter 80–120 μm), representing the expansion of the spermatogonial stem cell. In Holstein bulls, the number of spermatogonia increased from 1.8 × 106 cells/testis at 4 months of age to 3.8 × 109 cells/testis at 8 months of age; the number of spermatogonia continued to increase until approximately 12 months of age (Curtis and Amann, 1981). A-spermatogonia divided mitotically to form In- and B-spermatogonia, which, in turn, entered meiosis at around 4–5 months of age, when primary spermatocytes were first observed. The numbers of primary spermatocytes increased slowly until 8 months of age, when they exceeded the number of spermatogonia. Secondary spermatocytes and round spermatids first appeared at approximately 6–7 months of age, whereas elongated spermatids appeared at around 8 months of age. The number of spermatids increased rapidly and, after 10 months of age, spermatid numbers exceeded the numbers of any other germ cell. Mature sperm appeared in the seminiferous tubules at approximately 8–10 months of age (Fig. 12.5). Testes weighing more than 100 g in Swedish Red-and-White bulls, or more than 80 g in Holstein bulls, were likely to be producing sperm (Abdel-Raouf, 1960; Curtis and Amann, 1981; Evans et al., 1996; Wrobel, 2000; Bagu et al., 2006). Testicular cellular development was accompanied by a progressive increase in the proportion of the testicular parenchyma occupied by seminiferous tubules until approximately 8 months of age. Seminiferous tubule diameter increased gradually from 2 to 5 months of age, and more rapidly from 6 to 10 months of age, increasing fivefold from birth until adulthood. Around 6 months of age, ‘cracking’ of the tubular cytoplasm was first detected, indicating formation of the tubular lumen. Formation of the tubular lumen was evidence of a functional blood–testis barrier and preceded the appearance of primary spermatocytes and more advanced germ cells. Tubular lumen diameter continued to increase until approximately 8 months of age. Total seminiferous tubule length increased from 830 m/testis at 3 months of age to 2010 m/testis at 8 months of age in Holstein bulls. Therefore, increases in the proportion of parenchyma that was occupied by seminiferous tubules, as well as increases in the tubular diameter and the total length of seminiferous tubules per testis, accounted for initial testicular growth up to 8 months of age; thereafter, testicular growth was primarily the result of increasing total seminiferous tubule length (Abdel-Raouf, 1960; Curtis and Amann, 1981). Testicular echogenicity increased at about 6 and 10 months of age in beef bulls, accompanying the testicular histological changes. When data were analysed according to age at puberty, testicular echogenicity started to increase 16 to 12 weeks before puberty and reached maximum values 4 weeks before or at puberty. These observations indicate that a certain developmental stage of the testicular parenchyma must be reached before puberty and that the composition of the parenchyma remained consistent after puberty (Brito et al., 2012a). The accelerated testicular growth observed after 6 months of age in bulls occurs when circulating gonadotrophin concentrations are decreasing, which points to the existence of important GnRH-independent mechanisms regulating testicular development. The period of accelerated testicular growth coincides with increasing circulating IGF-I and leptin concentrations, and strong associations between these hormones and testicular size have been observed in growing beef bulls (Brito et al., 2007a,d), indicating that metabolic hormones may be involved in regulating GnRH-independent testicular development. As there was no association between circulating metabolic hormones and gonadotrophin concentrations, the possible effects of metabolic hormones on testicular growth were apparently direct and independent of the hypothalamus and pituitary. Although IGF-I and leptin concentrations were associated with testicular size, there were no associations between these hormones and seminiferous tubule diameter and area, seminiferous epithelium area, or volume occupied by the seminiferous tubules (L. Brito, unpublished). These observations suggest that increased circulating IGF-I and leptin concentrations were associated with increased length of the seminiferous tubules and probably with overall increases in the total number of testicular cells. Considering the cellular events in the testis during the pubertal period, the temporal patterns of metabolic hormone concentrations in bulls indicated that circulating IGF-I and leptin could be involved in regulating Leydig cell multiplication and maturation, Sertoli cell maturation and germ cell multiplication during the period of accelerated GnRH-independent testicular growth. Testicular concentrations of LH and FSH receptors in beef bulls decreased around 5 to 6 months of age, but increased thereafter until at least approximately 13 months of age; this might act to increase the sensitivity of Leydig and Sertoli cells to the low concentrations of gonadotrophins that occur during the rapid testicular growth phase (Bagu et al., 2006). Other mechanisms that might be associated with GnRH-independent testicular growth include changes in testicular concentrations and bioavailability of growth factors such as transforming growth factor (TGF-alpha and TGF-beta 1, 2 and 3) and interleukins (IL-1 alpha, IL-1 beta and IL-6) (Bagu et al., 2010a,b). In addition, experiments evaluating the effect of nutrition on sexual development have demonstrated that the impact of gonadotrophins on target tissues during the prepubertal period have long-term effects on testicular development in bulls. Bulls with either greater LH pulse frequency or more sustained increased LH pulse frequency during the early gonadotrophin rise had a more prolonged period of increased testicular growth and greater testicular size at 15–16 months of age, even when no differences in metabolic hormones or testosterone concentrations were observed after 6 months of age. These results indicate that the putative effects of circulating metabolic hormones, gonadotrophins, local growth factors and other unknown factors during the period of accelerated testicular growth might be dependent on the previous LH exposure during the prepubertal period. The LH secretion pattern during the early gonadotrophin rise seems to ‘prime’ testicular development, dictating maximum adult testicular size in bulls (Brito et al., 2007b,c,d). After spermatogenesis is established, there was a gradual increase in the number of testicular germ cells supported by each Sertoli cell and an increase in the efficiency of the spermatogenesis, i.e. an increase in the number of more advanced germ cells resulting from the division of precursor cells. The yields of different germ cell divisions, low during the onset of spermatogenesis, increased progressively to the adult level (Macmillan and Hafs, 1968; Curtis and Amann, 1981). The eventual appearance of sperm in the ejaculate was the result of the increasing sperm production efficiency after initiation of spermatogenesis. In general terms, puberty is defined as the process of changes by which a bull becomes capable of reproducing. This process involves the development of the gonads and secondary sexual organs, and the development of the ability to breed. For research purposes however, puberty in bulls is usually defined as an event instead of a process. Most researchers define attainment of puberty by the production of an ejaculate containing ≥50 million sperm with ≥10% motile sperm (Wolf et al., 1965). The interval between the first observation of sperm in the ejaculate and puberty as defined by these criteria was approximately 30 to 40 days (Lunstra et al., 1978; Jiménez-Severiano, 2002). Age at puberty determined experimentally can be affected by the age that semen collection attempts are performed, the interval between attempted collections, the method of semen collection (artificial vagina or electroejaculator), the response of the bull to the specific semen collection method and the experience of the collector(s). Moreover, age at puberty is affected by management, nutrition (see below) and genetics. Table 12.1 describes age, weight and SC at puberty in different breeds. Although data from large trials comparing bulls of different breeds raised as contemporary groups are scarce, some liberties could be taken to make some generalizations from these data. Dairy bulls usually mature faster and attain puberty earlier than beef bulls. Bulls from continental beef breeds, with the possible exception of Charolais, usually attain puberty later than bulls from British beef breeds, especially Angus bulls. Bulls from double-muscled breeds are notorious for being late maturing. Puberty is also delayed in bulls from tropically adapted B. taurus breeds and in non-adapted bulls raised in the tropics. There is a large variation in age and body weight at puberty across breeds and within breeds. Although, on average, bulls attain puberty at an SC of between 28 and 30 cm, regardless of the breed, the fact that there is still considerable variation in SC at puberty is sometimes overlooked. Interesting observations have been reported in studies evaluating differences between early- and late-maturing bulls. Bulls that attained puberty earlier were generally heavier and had greater SC than bulls that attained puberty later; however, both weight and SC were smaller at puberty in early-maturing bulls (Evans et al., 1995; Aravindakshan et al., 2000). These observations not only indicate that precocious bulls develop faster, but also suggest that sexual precocity is not simply related to the earlier attainment of threshold body or testicular development. In fact, these thresholds seem to be lower in early-maturing bulls, and late-maturing bulls must reach a more advanced stage of body and testicular development before puberty is attained. Similar observations have also been reported in B. taurus × B. indicus crossbred bulls (Brito et al., 2004b). Semen quality in pubertal bulls was generally poor with a gradual improvement characterized by an increase in sperm motility and a marked reduction in morphological sperm abnormalities being observed after puberty. The most prevalent sperm defects observed in pubertal bulls were proximal cytoplasmic droplets and abnormal sperm heads (approximately 30–60% and 30–40% at puberty, respectively) (Lunstra and Echternkamp, 1982; Evans et al., 1995; Aravindakshan et al., 2000). In a series of studies with beef bulls, age at puberty and age at satisfactory semen quality (≥30% sperm motility, ≥70% morphologically normal sperm) were 308 and 372 days, respectively. Moreover, 10% of the bulls did not have satisfactory semen quality by the end of the experimental period at 15–16 months of age (L. Brito, unpublished data and Fig. 12.6). The results of another study with beef bulls in Western Canada indicated that the proportions of bulls with satisfactory sperm morphology at 11, 12, 13 and 14 months of age were approximately 40, 50, 60 and 70%, respectively (Arteaga et al., 2001). Similarly, only 48% of beef bulls 11 to 13 months old in Sweden had <15% proximal cytoplasmic droplets and <15% abnormal sperm heads (Persson and Söderquist, 2005). These observations have profound implications for the ability of producers to use yearling bulls and the ability of artificial insemination (AI) centres to produce semen for progeny testing at the youngest possible age. Table 12.1. Age, weight and scrotal circumference (SC) at puberty (ejaculate with ≥50 million sperm and ≥10% sperm motility) in different breeds. For crossbred bulls, the first breed noted indicates the sire’s breed. The epididymis continued to grow until at least 6 years of age in Holstein bulls, with epididymal weight increasing from 9 g at 8 months of age to 15, 23, 27 and 38 g at 12, 18, 25–48 and 73–96 months of age, respectively (Almquist and Amann, 1961; Killian and Amann, 1972). The tube-like vesicular glands in newborn calves increased in length and became lobulated during development. The weight of the vesicular glands increased until approximately 4 years of age in Holstein bulls – from 13 g at 8 months of age to 26, 35, 54 and 78 g at 12, 18, 25–48 and 73–96 months of age, respectively (Almquist and Amann, 1961; Killian and Amann, 1972). The sigmoid flexure of the penis began to develop at about 3 months of age. Penis length increased by up to five times by the onset of puberty, and length continued to increase until sexual maturity (Coulter, 1986). The penis in Friesian bulls 13 to 19 months old measured 73 to 89 cm (Ashdown et al., 1979a); in contrast, the penis in Holstein bulls ≥25 months old measured 95 to 106 cm (Almquist and Amann, 1961). First protrusion of the penis during mounting was observed at approximately 8 months of age, with complete separation of the penis and sheath observed at approximately 8.5 months of age in Angus, Charolais and Hereford bulls (Wolf et al., 1965; Barber and Almquist, 1975). Complete sheath–penile detachment evaluated during electroejaculation (EE) was observed at around the same time of puberty, whereas first completed service – when evaluated during libido testing – was only observed approximately a month after puberty, indicating that bulls started producing sperm before complete sheath–penile detachment (Lunstra et al., 1978). Very few studies have evaluated the effect of nutrition from birth to maturity on sexual development and reproductive function in bulls. Compared with Holstein bulls receiving control nutrition (100% of requirements), bulls receiving low nutrition (approximately 60–70% of requirements) from birth were older at the time the first ejaculates containing motile sperm were collected and also had smaller testes, but bulls receiving high nutrition (approximately 160% of requirements) had earlier puberty and larger testes (Bratton et al., 1959; Flipse and Almquist, 1961). These observations have been corroborated and expanded in a series of more recent experiments that have also shown that the most pronounced effects of nutrition occur during the prepubertal period. The recent studies have demonstrated that the adverse effects of low nutrition during the prepubertal period cannot be compensated for by improved nutrition during the pubertal period, and that the beneficial effects of high nutrition during the prepubertal period are sustained even if maintenance diets are fed thereafter. Low nutrition (75% of requirements) during the peri-pubertal period in beef bulls reduced LH pulse secretion, delayed the increase in circulating testosterone concentrations, delayed puberty and resulted in decreased testicular size at 16 months of age; high nutrition (125% of requirements) produced the opposite results (Brito et al., 2007b,c,d). The effects of nutrition on the sexual development and reproductive function of bulls were mediated through the hypothalamic-pituitary-testicular axis. Nutrition affects the GnRH pulse generator in the hypothalamus, because differences in LH pulse secretion in bulls receiving different nutrition can be observed even in the absence of differences in pituitary LH secretion capability, as determined by GnRH challenge (Brito et al., 2007c,d). Interestingly, when low nutrition was imposed on bulls by limiting the amount of nutrients in a ration fed ad libitum, only reduced LH pulse frequency was observed; but when nutrition was restricted by restricting food availability, reduced LH pulse frequency, mean and peak concentrations and secretion were observed after GnRH challenge, (Brito et al., 2007b). These results seem to indicate that the inhibitory effects of limited nutrient availability on LH secretion appear to be exerted on the hypothalamus only, while the combination of limited availability of nutrients combined with hunger sensation experienced by bulls with restricted intake affected both hypothalamic and pituitary function, producing a much more severe inhibition of LH secretion. The effect of nutrition on Leydig cell number and/or function in bulls receiving different diets was demonstrated by differences in testosterone secretion after GnRH challenge, even in the absence of differences in LH secretion after the challenge (Brito et al., 2007c,d). Differences in yearling SC due to age of the dam in beef bulls could also be interpreted as an indication that nutrition during the pre-weaning period affects sexual development, although possible in utero effects cannot be completely ruled out. SC in beef bulls increases as age of the dam increases up to 5 to 9 years of age and then decreases as dams get older. Adjustment factors of 0.7–1.4 cm, 0.2–1.0 cm, 0.1–1.0 cm and 0.3–0.75 cm for yearling SC have been suggested for bulls raised by 2-, 3-, 4- and ≥10-year old dams, respectively (Bourdon and Brinks, 1986; Nelsen et al., 1986; Lunstra et al., 1988; Kriese et al., 1991; Evans et al., 1999; Crews and Porteous, 2003). In these studies, the inclusion of weight as a covariate in the models describing SC resulted in decreased effects of age of the dam, indicating that the effect of dam age on testicular growth seems to be primarily the result of dam age effects on the bull’s body weight, probably related to differences in milk production. This theory is also supported by reports that, as observed in bulls receiving low nutrition, LH secretion after GnRH challenge was greater from 3.5 to 6 months of age in bulls raised by multiparous rather than by primiparous females (Bagu et al., 2010c). Several studies have described the effects of nutrition during the pubertal period only, i.e. after the initial hormonal changes regulating sexual development have occurred. In general, these studies indicate that low nutrition has adverse effects on growth and sexual development. In one study, bulls receiving one third of the amount supplied to their twin controls had lower body and vesicular gland weights, vesicular gland fructose and citric acid contents, and circulating and testicular testosterone concentrations, whereas circulating androstenedione concentrations were increased (Mann et al., 1967). In other experiments, beef bulls 8 to 12 months old receiving diets with low levels of crude protein (8, 5 and 1.5%) for periods of 3 to 6 months had markedly reduced weights of the testes, epididymis and seminal glands compared with control bulls fed diets containing 14% crude protein. Moreover, seminiferous tubule diameter and seminiferous epithelium thickness were smaller in bulls that were on restricted protein intake (Meacham et al., 1963, 1964). Though low nutrition during the pubertal period has adverse effects on reproductive function, the potential beneficial effects of high nutrition after weaning are questionable, at best. Effects of energy on sexual development were not consistent in a study with Simmental and Hereford bulls fed diets with low, medium or high energy content (approximately 14, 18 and 23 Mcal/bull daily, respectively) from 7 to 14 months of age. Dietary energy affected sexual development in Simmental bulls, but not in Hereford bulls. Simmental bulls in the high-energy group were heavier and had greater SC and testosterone concentrations than bulls in the low-energy diet group (in general, the medium energy group had intermediate values). However, increased dietary energy did not hasten age at puberty. The only semen trait affected by dietary energy was semen volume, which was depressed in Simmental bulls in the medium-energy group. Serving capacity was greater for Hereford bulls in the high-energy diet; in contrast, medium- and high-energy diets were associated with a decrease in the number of services between two testing periods in Simmental bulls. There was a trend for lower sperm motility and a lower proportion of normal sperm in Simmental bulls fed the low-energy diet (Pruitt and Corah, 1985; Pruitt et al., 1986). In Holstein bulls producing semen for AI, high energy intake was associated with visual evidence of weakness of the feet and legs and increased reaction time after 3 years of age (Flipse and Almquist, 1961). Under field conditions, post-weaning high-energy diets are frequently associated with impaired reproductive function in bulls, which is most likely related to altered testicular thermoregulation as a result of excessive fat deposition above and around the testes in the scrotum. In one report, sperm motility decreased and the proportion of sperm defects increased with age in Hereford bulls fed to gain >1.75 kg/day, and significantly differed from bulls fed to gain approximately 1 kg/day (control). Even after the high nutrition diet was changed to a control diet, bulls previously receiving high nutrition continued to have lower semen quality. There was greater deposition of fat around the testicular vascular cone in the scrotal neck in bulls in the high nutrition group, and the difference between body and testes temperature was reduced in this group compared with that of bulls in the control group. This difference was still present after the high-energy diet was changed and the bulls had lost a considerable amount of weight, indicating that fat that has accumulated in the scrotum is more difficult to lose than other body fat (Skinner, 1981). In another series of experiments, Angus, Hereford and Simmental bulls were fed high nutrition (80% grain and 20% forage) or medium nutrition (forage only) from approximately 6.5 until 12–24 months of age. In general, bulls receiving high nutrition had greater body weight and back fat than did the medium nutrition group, although PTW was not affected by diet. Furthermore, bulls receiving high nutrition had lower daily sperm production and epididymal sperm reserves, and a greater proportion of sperm abnormalities. These authors indicated that increased dietary energy may adversely affect sperm production and semen quality owing to fat deposition in the scrotum, which reduces the amount of heat that can be radiated from the scrotal skin, thereby increasing the temperature of the testes and scrotum (Coulter and Kozub, 1984; Coulter et al., 1987a, 1997; Coulter and Bailey, 1988). Observations from another study indicated that bulls fed high-nutrition diets had greater SC and scrotal weight than did bulls fed medium-nutrition diets, though PTW was not different between the two groups (Seidel et al., 1980). Growth rate between 6 and 16 months of age did not affect sexual development and reproductive function in Angus and Angus × Charolais bulls. However, greater body weight at various ages was associated with reduced age at puberty and maturity, and with larger testes at 16 months of age, indicating that improved nutrition might be beneficial, but only when offered before 6 months of age. Average daily gains of 1 to 1.6 kg/day did not result in excessive fat accumulation in the scrotum, or in increased scrotal temperature or reduction in sperm production and semen quality, and could be considered ‘safe’ targets for growing beef bulls (Brito et al., 2012b). This summary of the literature supports the intuitive assumption that low nutrition has adverse effects on sexual development and reproductive function regardless of a bull’s age. Then again, most research seems to indicate that high nutrition is only beneficial during the first 6 months of life, which presents a challenge to bull producers. Beef bull calves are often nursed until 6 to 8 months of age, with very little attention being paid to their nutrition, whereas nutrition offered to dairy bull calves is often suboptimal. Efforts to obtain maximum weight gain during the first months after birth by offering highly nutritious diets and adopting management practices such as creep feeding should be compensated by reduced age at puberty and greater sperm production capacity in adult bulls. It is also clear that, although high nutrition diets after 6 months of age might be associated with greater SC, this effect is likely to be caused by fat accumulation in the scrotum and is not actually the result of greater testicular size. Moreover, sperm production, semen quality and serving capacity are all compromised in bulls receiving excessive nutrition after this age. Adjusting diets accordingly to maximize growth, but prevent over-conditioning after the peri-pubertal period, is advisable. Testes in bulls are maintained at 4–5.5oC below body temperature and this lower temperature is essential for normal spermatogenesis (Kastelic et al., 1995, 1997). Adequate testicular temperature is maintained by complex physiological mechanisms that involve the scrotum, the testicular vascular cones and the testes proper. The temperature is determined primarily by the heat conveyed to the testes by the arterial blood, and the temperature of the arterial blood reaching the testes is determined by the amount of heat lost in the testicular vascular cone. This, in turn, is largely dependent on the temperature of the venous blood leaving the testes. Because the testicular veins run on the surface of the testes just below the tunica albuginea, the temperature of the testicular venous blood is greatly affected by the temperature of the scrotum. Therefore, the scrotum, which is equipped with thermoreceptors, is responsible for actively regulating testicular temperature by controlling vascular flow, sweat gland activity and muscle contractility (Waites, 1970; Setchell, 1978; Sealfon and Zorgniotti, 1991). Increased testicular temperature results in reduced vasoconstrictor tone of the smooth muscles of the skin arteries, which increases blood flow to the scrotum and heat loss by irradiation. Increased testicular temperature also results in increased scrotal sweat production and heat loss by evaporation; scrotal sweat gland density and sweat production are greater than in the skin of other parts of the body (Blazquez et al., 1988). The cremaster muscle and the dartos tunic become completely relaxed when testicular temperature rises, thus maintaining the testes as far away as possible from the abdomen, maximizing the contact of the testes with the scrotum and increasing the scrotal surface area for heat irradiation. Scrotal surface temperature is lower than both body and intra-testicular temperatures, and a positive temperature gradient is also observed between the proximal and distal parts of the scrotum (Plate 32). Scrotal surface temperatures of approximately 30–31oC at the proximal portion and 28–29oC at the distal portion have been reported in beef bulls (Cook et al., 1994; Kastelic et al., 1995, 1996a, 1997). The testicular vascular cone is a specialized anatomical structure that plays an important role in cooling testicular arterial blood before it reaches the testes and, conversely, in warming venous blood before it re-enters the abdomen. The cone is formed mainly by the very sinuous testicular artery juxtaposed between the fine networks of testicular veins that form the pampiniform plexus. Heat transfer in the testicular vascular cone occurs through a countercurrent mechanism that involves heat transfer between fluids of different temperatures flowing in opposite directions inside adjacent vessels. The degree of heat exchange is determined exclusively by the temperature gradient between the arterial and venous blood, but the efficiency of the mechanism is determined by the length and volume of the artery, the area of the artery’s surface contacted by veins, and the distance between the arterial and venous blood (Sealfon and Zorgniotti, 1991). Heat loss by irradiation through the scrotal skin in the vascular cone area is also important, because this is the warmer part of the scrotum and insulation of this area results in increased testicular temperature (Kastelic et al., 1996b). Anatomical changes of the testicular vascular cone as the bull ages and starts to produce sperm are indications that the efficiency of the countercurrent mechanism needs to improve in order to couple with increasing testicular metabolism. In Angus and Angus × Charolais bulls, the testicular vascular cone diameter measured by ultrasonography increased until approximately 13.5 months of age, or until 1 to 8 weeks before the SC reached a plateau (Brito et al., 2012c). In crossbred beef bulls, the length and diameter of the testicular artery in the vascular cone increased from 6 to 12 months of age (1.8 m and 1.9 mm versus 3.1 m and 3.5 mm, respectively), but did not increase significantly thereafter (Cook et al., 1994). In 15-month-old Angus bulls, the testicular artery length and volume in the vascular cone were 1.6 m and 6 ml, respectively (Brito et al., 2004a). Other studies have reported that the testicular vascular cone length was approximately 10 to 15 cm and that the testicular artery length varied from 1.2 to 4.5 m in adult beef bulls of several breeds (Kirby, 1953; Kirby and Harrison, 1954; Hofmann, 1960). In addition to the lengthening of the testicular artery, the distance between the arterial and venous blood in the testicular vascular cone also decreased with age. This occurred via thinning of the arterial wall (from 317 to 195 μm thick at 6 and 36 months of age, respectively) and reduction of the distance between the artery and the closest veins (Cook et al., 1994). Another interesting observation was that the artery wall thickness and distance between the artery and the veins also decreased from the proximal to the distal portions of the testicular vascular cone (Hees et al., 1984; Cook et al., 1994; Brito et al., 2004a). The reduction of the distance between the warmer arterial blood and the cooler venous blood may facilitate heat transfer and compensate for the gradual reduction in the arterial–venous temperature gradient that occurs as blood flows through the vascular cone. Evaluation of intra-artery temperature on the dorsal portion of the testis indicated that the arterial blood was cooled down by 3–4.5°C after passing through the testicular vascular cone in 15–18 month-old beef bulls (Kastelic et al., 1997; Brito et al., 2004a). The testicular artery reaches the dorsal pole of the testis and runs under the tunic albuginea, in close relation to the epididymal body, until it ramifies at the ventral pole. After ramification, several arterial branches run dorsally under the tunic until they penetrate into the testicular parenchyma. The temperature of the arterial blood decreases only slightly between the testicular dorsal pole and the ventral pole, but decreases significantly between the ventral pole and the point of penetration. This arrangement results in a negative sub-tunic temperature gradient between the dorsal and ventral portions of the testis. The combination of the opposing ‘top-to-bottom’ temperature gradients of the scrotum (positive gradient) and the testicular surface (negative gradient) results in a constant temperature throughout the entire testicular parenchyma. Intra-testicular temperature in beef bulls 15–18 months old was approximately 33.5–35oC (Kastelic et al., 1996a, 1997; Brito et al., 2004a). Daily sperm production is defined as the total number of sperm produced per day by the testes, whereas spermatogenesis efficiency is the number of sperm produced per gram of testicular parenchyma. Spermatogenesis efficiency increased with age and reached adult levels at approximately 12 months of age in Holstein bulls (Macmillan and Hafs, 1968; Killian and Amann, 1972). Individual variation in spermatogenesis efficiency was relatively small and was not affected by ejaculation frequency, with values between 10 and 14 million sperm/g parenchyma being reported for dairy and beef bulls (Macmillan and Hafs, 1968; Killian and Amann, 1972; Amann et al., 1974; Weisgold and Almquist, 1979; Almquist, 1982; Johnson et al., 1995; Lunstra and Cundiff, 2003). As sperm production per gram of testicular parenchyma is somewhat constant among bulls, the daily sperm production of a bull is largely dependent on the weight of the testes. Considering testicular weight at different ages, yearling bulls are expected to produce around 4–5 billion sperm per day, whereas adult bulls are expected to produce around 7–9 billion sperm per day. Sperm output (number of sperm in the ejaculate) in bulls ejaculated frequently (i.e. when extragonadal reserves are stabilized) is essentially the same as sperm production (Amann et al., 1974). One important difference between young and older bulls is the capacity of the epididymis to store sperm. Evaluation of sperm numbers in the tail of the epididymis of 15–17 month-old Holstein bulls demonstrated that sperm available for ejaculation corresponded to approximately 1.5–2 days of sperm production; in older (2–12 year-old) bulls, stored sperm numbers corresponded to the larger amount of approximately 3.5–5 days of sperm production (Amann and Almquist, 1976; Amann, 1990). These observations are especially important for AI centres and indicate that more frequent semen collection is necessary to maximize sperm harvest from young bulls; in older bulls, semen collection intervals of less than 2–3 days have smaller effects on increasing sperm harvest. Sperm output increases with increased ejaculation interval up to the number of days required for epididymal storage capacity to reach its limit. Sperm that are not ejaculated are eliminated with urine or during masturbation. No differences were found in testicular development, spermatogenesis efficiency or extragonadal sperm reserves at 7 years of age between Holstein bulls that had been submitted to semen collection frequencies of either once or six times a week from 1 year of age using artificial vaginas, indicating that ejaculation at high frequency was not detrimental to either testicular development or sperm physiology. More importantly, the lack of adverse effects on sperm production and semen quality supported the long-term use of a high rate of ejaculation to maximize sperm harvest from bulls in AI centres (Almquist, 1982). With increased weekly ejaculation frequency there was a decrease in ejaculate volume, though sperm concentration was not affected (Almquist and Cunningham, 1967; Almquist et al., 1976). Although ejaculate volume either remained unchanged or decreased with multiple ejaculations on the same day, there was a gradual decrease in sperm concentration and total sperm per ejaculate. In Angus bulls from which seven consecutive ejaculates were obtained, total sperm number decreased continuously from the first to the third ejaculate, but did not change significantly thereafter. The first, first two and first four ejaculates contained 31, 55 and 77%, respectively, of the total sperm output obtained in the seven ejaculates (Foster et al., 1970). A small but significant increase in the proportion of motile sperm in consecutive ejaculates was observed in some studies (Almquist and Cunningham, 1967; Foster et al., 1970; Almquist et al., 1976). In 10–18 month-old Holstein bulls, volume and concentration of the first and second ejaculates were 4 and 3.3 ml and 1.14 and 0.63 billion/ml, respectively (Diarra et al., 1997), whereas in Holstein bulls of various ages, volume, sperm concentration and total sperm in the first and second ejaculates were 8.2 and 7.3 ml, 1.5 and 0.95 billion/ml and 12.0 and 6.8 billion, respectively (Everett and Bean, 1982). Ejaculate volume, sperm concentration and total sperm in dairy and beef B. taurus bulls in Brazil were 6.9 to 8.2 ml, 1.2 billion/ml and 8.2 to 9.1 billion, respectively (Brito et al., 2002a,b). Sperm output is greatly affected by sexual preparation, which has been defined as prolonging the period of sexual stimulation beyond that adequate for mounting and ejaculation (Amann and Almquist, 1976). Optimal sexual preparation ensures that the ejaculate contains the greatest possible number of sperm. The techniques used for sexual preparation include restraint and false mounts, i.e. mounting without ejaculation. While restraint can be effective, it is important to ensure that the bull is actually stimulated and not simply standing close to the stimulus animal; in practice, this can be a subjective assessment. In contrast, false mounting is evident to the observer and therefore is always recommended, except for bulls with severe physical disability. Sperm output increases with both increased restraint time and number of false mounts. In one study with dairy bulls, total sperm numbers in the ejaculate were 7.1, 11.7, 14.2 and 13.5 billion with zero, one, two and three false mounts, respectively. When 5 min of restraint was also used, total sperm numbers were 14.1, 13.8, 15.1 and 17.4 billion, respectively. Greater sperm output was mainly associated with increased semen volume and less so with increased sperm concentration (Hafs et al., 1962). However, dairy and beef bulls respond differently to sexual preparation. In one study, sperm harvest from Angus and Hereford bulls using three false mounts as sexual preparation did not differ from that obtained by using one false mount only and 5 min of restraint, or using no false mounts at all (Foster et al., 1970). Another study demonstrated that total sperm output in two ejaculates from Angus bulls increased significantly if three false mounts were used before collection of the first ejaculate, although the increment in output was minimal when the same sexual preparation was used for the second ejaculate (Almquist, 1973). A good understanding of the dynamics of spermatogenesis is crucial for understanding abnormal sperm production. Total duration of spermatogenesis is 61 days in bulls. After spermiation, sperm are transported through the epididymis in a process that takes approximately another 10 days. Thus, sperm present in the ejaculate began being produced 71 days earlier and semen quality is a reflection of those events in the previous 2 months that influenced spermatogenesis, sperm maturation and transport. Different testicular germ cell lines have different sensitivities to different insults. For example, spermatocytes and spermatids are particularly sensitive to increased testicular temperature, but spermatogonia are more resistant (Setchell, 1998). Therefore, particular changes in semen quality are manifested after an interval that varies according to the developmental stage of the germ cells at the time of the insult, and the time required for the damaged cells to be released into the seminiferous tubules and transported through the epididymis. If exposure to the insult is limited, a consistent sequence of appearance of sperm defects in the ejaculate is expected, whereas if the exposure is prolonged, a variety of sperm defects may be simultaneously present in the ejaculate. Semen quality improves as the spermatogonia that resisted the insult enter mitosis and meiosis and restart generating normal sperm. The mildest form of testicular degeneration produces no grossly detectable changes in the testicular tissue and is manifested exclusively by increased production of abnormal sperm. Elevated testicular temperature and endocrine disruption are probably the most common causes of mild testicular degeneration. Normal spermatogenesis in bulls depends upon maintenance of optimal testicular temperature, with increased testicular temperature having detrimental effects on sperm production and semen quality. Metabolic rate and oxygen demand increase as a result of augmented testicular temperature. However, the long and extremely coiled testicular artery limits the blood supply to the testes. As blood flow to the testes either does not increase at all, or does not increase sufficiently to match the increased metabolic rate of the heated tissue, testicular hypoxia develops (Setchell, 1998). Causes of increased testicular temperature include increased whole body temperature (high ambient temperature, pyrexia), increased local temperature (scrotal trauma or dermatitis, orchitis, periorchitis, epididymitis), decreased local heat irradiation (hydrocele, scrotal oedema, fat accumulation around the spermatic cords), and alteration of normal testicular mobility (tunic adhesions, inguinal and scrotal hernias). Scrotal insulation for more than 24 h results in decreased sperm motility and an increased proportion of abnormal sperm in the ejaculate 7–12 days afterwards. The proportion of abnormal sperm peaks at around 18 to 21 days after insulation, and sperm motility and morphology return to pre-insulation levels only after about 45 days (Fig. 12.7). The sequential appearance of sperm defects after scrotal insulation included an increase of detached sperm heads between 7 to 14 days, midpiece defects between 7 and 21 days, cytoplasmic droplets between 7 and 28 days, nuclear vacuoles between 18 and 21 days, and abnormal sperm head and acrosome defects between 21 and 28 days. There was usually marked individual variation in the proportion of specific sperm defects that were observed after scrotal insulation (Austin et al., 1961; Vogler et al., 1993; Barth and Bowman, 1994). While the increase in abnormal sperm seems to be mainly a reflection of insult to spermatids, decreased sperm production is also often observed after longer periods of scrotal insulation, indicating that spermatocytes are also affected by elevated temperature, but are more likely to degenerate than to develop into abnormal sperm. Exposure of bulls to high temperatures in controlled-environment chambers has profound physiological effects that might also interfere with testicular function. Nevertheless, the observed changes in semen quality resemble those occurring after scrotal insulation (Casady et al.
ABS Global, Inc., DeForest Wisconsin, USA
Introduction
Sexual Development
Hormonal control of sexual development
Postnatal testicular development and establishment of spermatogenesis
Puberty
Effects of nutrition on sexual development
Testicular Thermoregulation
Sperm Production and Semen Quality
Stay updated, free articles. Join our Telegram channel
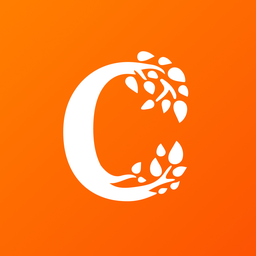
Full access? Get Clinical Tree
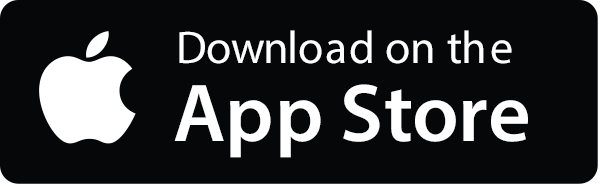
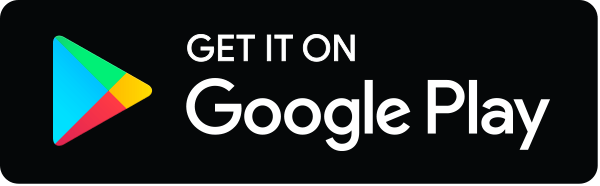