CHAPTER 136 Application of Embryo Biotechnologies in Farmed Cervids
Farmed cervids represent newly adapted species to domestication possessing unique reproductive characteristics and husbandry requirements. High relative market value, demand for rapid genetic advancement, and international genetic exchange have all promoted significant interest in the development of embryo-based reproductive technologies in these species. Embryo biotechnologies have proved themselves as exceptionally valuable tools for use by progressive animal breeders in other domestic livestock species. Although a myriad of technologies currently exist by which embryos can be either created or modified, all embryo biotechnologies applied to the production of living progeny must end in the transfer of embryos to suitable recipients. The basic processes of embryo recovery, manipulation, and transfer referred to collectively as embryo transfer, brings with it powerful production and clinical benefits related to maximizing female reproductive capacity, advancing the rate of genetic progress through shortening of generation intervals, lessening the cost of genetic transport and threat of disease transfer, as well as the intentional elimination of specific environmental pathogens from infected donor populations. The adaptation of embryo biotechnologies for use in farmed cervids has been ongoing for a number of decades. Recent advances in transcervical artificial insemination, embryo recovery, and embryo transfer technologies in red deer, as well as the development of in vitro embryo production systems, is continuing the clinical development of embryo transfer in cervid species. This chapter will describe the current status of embryo biotechnology development and use in farmed red deer and wapiti (North American elk) and give effective clinical methodologies where available.
CERVID BIOLOGY, REPRODUCTION, AND EMBRYOLOGY
Collectively, cervids have experienced a long and complex association with humans, inspiring study and pursuit as a primary food source over the millennia. Only in recent history have we attempted to domesticate and selectively breed a limited number of cervid species for agricultural purposes. Chief among those selected for domestication have been members of the Cervus elaphus family. Members of the Cervus elaphus family, including European red deer (Cervus elaphus elaphus), North American elk (Cervus elaphus nelsoni, manitobensis, and roosevelti), and Siberian elk (Cervus elaphus siberi), while broadly spread both in terms of their geographic distribution and phenotypic appearance, interbreed readily, producing fertile hybrids. Wapiti and red deer exhibit a seasonally restricted polyestrous form of reproduction in which unmated females ovulate a single oocyte at intervals averaging 18 days in red deer and 21 days in wapiti (see Chapter 125). Wapiti and red deer have additionally evolved naturally to calve in sequence with the flush of grass growth in the spring. Calving in conjunction with spring grass growth requires successful conception to occur in September-October in North America, as gestation lengths average 233 days in red deer and 245 days in wapiti. A return to cyclicity in mature females and the initiation of cyclicity in yearlings occurs in response to decreasing daylight length. In North America, wapiti generally begin to exhibit fertile estrous cycles in the middle of September, while red deer hinds begin to cycle in early October. In unmated females, ovulations may continue to occur through March or April. Such a period of extended cyclicity rarely occurs, however, as the high natural fertility exhibited in wapiti and red deer receiving adequate nutritional support generally leads to a high percentage of females conceiving (>85%) on their first estrous cycle. In both wild and farmed wapiti and red deer, breeding activity is thus concentrated to a very restricted period in the early fall.
Embryonic development in wapiti and red deer takes place in the oviduct and the uterus. The first, second, third, fourth, and often fifth embryonic cell cycles transpire within the lumen of the oviduct in close association with the mucosal epithelium. Wapiti and red deer embryos appear to first enter the uterine lumen approximately 6 days following ovulation. Coincident or just prior to their entrance into the uterus, wapiti and red deer embryos undergo a process of cellular compaction during the fifth cell cycle, which leads to development of distinct inner and outer cell groups. Compaction is followed by the formation of the fluid-filled cavity known as the blastocelic cavity within the embryo. Formation of this blastocelic cavity coincides with the initial differentiation of epithelial cells from pluripotent embryonic cells and denotes transformation from the compact morula to blastocyst stage. Cellular differentiation and cavitation occur around 6.5 days after ovulation in both red deer and wapiti. Wapiti and red deer embryos are generally darker in color than bovine or ovine embryos at both the compact morula and blastocyst stages. Hatching of blastocyst stage embryos can occur as early as 7.5 days following ovulation, with elongation occurring between days 13 and 16.1 Elongated red deer blastocysts signal their presence to the maternal system through the secretion of antiluteolytic interferon between days 16 and 22.2 Attachment of blastocysts occurs by day 30 of gestation.1 Fetal growth in wapiti and red deer is linear between days 30 and 50 of gestation with significant differences in both fetal size and placentome development at each of these gestational time points readily apparent by ultrasound examination.3 Cervid artificial breeding programs involving group synchronization make use of these differences by delaying the introduction of backup bulls or stags for at least 10 days following artificial insemination (AI) or 4 days following the transfer of embryos so as to clearly distinguish between artificially and naturally sired conceptions.
APPLICATION OF ARTIFICIAL BREEDING TECHNOLOGIES IN WAPITI AND RED DEER
The wapiti and red deer breeding industries have been expeditious in their acceptance of reproductive biotechnologies. This rapid uptake of reproductive technology use has been encouraged by inherent factors unique to this industry, including a high level of natural fertility in red deer and wapiti, the co-advent of commercial wapiti and red deer farming with the evolution of commercially useful reproductive biotechnologies, as well as the ubiquitous pursuit of genetic advancement by breeders and the resulting competitive nature of the respective industries. The principal reproductive technology in clinical use within this species, as with other livestock species, is artificial insemination (see Chapter 134). Unique to the wapiti and red deer industries, however, is the universal use of a single timed insemination of cryopreserved semen at the beginning of the breeding season. As a carryover from their recent adaptation from the wild state, reproductive function in red deer hinds and wapiti cows suffers significantly from excessive handling. A single timed insemination allows for a reduction in handling stress to a minimum and the simultaneous concentration of management resources. The remarkable natural fertility of this species has permitted the evolution of a breeding system in which the timed insemination of a single straw of cryopreserved semen results in mean calving rates above 75%. The evolution of a timed single insemination system in wapiti and red deer has proved itself as an invaluable asset to the pursuit of genetic advancement and will serve as a model system for the development of all future reproductive technologies in this species. Of particular interest to the wapiti breeding industry is the production and clinical concern related to the successful covering of cows as early in the breeding season as possible. Though, as discussed previously, wapiti cows may exhibit fertile estrous cycles from September through April if left unmated, the natural condition of this species is for a very high percentage of wapiti cows to conceive at their first ovulation if covered successfully. Wapiti breeders generally wish to mimic this natural pattern of reproduction as closely as possible in farmed animals, as the value of calves is at least partially determined by their birth dates, with late-born calves (calves born after June) severely discounted. A further physiologic complication, adding to the restricted breeding season, is the rapid accumulation of internal fat that can occur in wapiti cows if left unbred. Prolonged intervals between weaning and breeding can lead to excessive deposition of pelvic fat that can negatively impact both the ability of cows to be artificially inseminated and dystocia rates at parturition. These combined factors are of such importance to the industry that all reproductive technologies applied to this species must be designed to be implemented in the 5-week period beginning in the middle of September. In the case of embryo-based biotechnologies, this concentration of breeding activity has acted to severely restrict the time available to the practitioner for donor manipulation, as breeders generally wish for donors themselves to conceive successfully within this same 5-week period. This has proved a most challenging restriction, as the hormonal manipulation of donors in preparation for embryo production often proves stressful and disruptive to donor cyclicity over an extended period following treatment. Reproductive technologies intended for use in cervids must thus be designed from their onset to be both limited in treatment duration and minimally invasive so as not to disrupt normal estrous cyclicity in the manipulated females immediately following treatment.
Embryo Biotechnologies
Prehatching stage embryos possess several unique characteristics that make them effective for use in advancing genetic change and health-related biosecurity. Chief among these beneficial characteristics are that they are very small (averaging ∼150 μm in diameter) and are encased within an impermeable protective shell. This glycoprotein shell, referred to as the zona pellucida, is equivalent to the shell on the familiar chicken egg, in that it completely encases the developing embryo and is impermeable to bacterial and viral pathogens. Because of the impermeability of the zone pellucida, zona intact embryos can be washed free of contaminating pathogens and thereafter be certified free of disease.4 While it is confined within the zona pellucida, mammalian embryos remain very small (∼150 µm in diameter) and are therefore very economical to transport to distant locations. It is the significant size advantage of the embryo that in fact grants embryo transfer systems their principal economic advantage over conventional live animal transport systems. Many hundreds and even thousands of embryos can be transported for less than the cost of a single airline ticket. An entire herd of deer or wapiti could be shipped using these methods to any location across the globe. These combined economic and health advantages make embryo transfer a logical choice for the safe international exchange of cervid genetics.
The potential power of embryo transfer within cervid production rests within two technologic pivot points on which both the genetic makeup and health status of selected animals can be manipulated. The first pivot point exists within the relationship between the biologic donor and the embryos that she produces. Within this relationship, while there is full genetic linkage between the biologic dam and her embryos, there is no health status relationship. By this we mean that although embryos inherit all their genes from their sire and dam, they inherit none of their biologic parents’ contaminating pathogens over the first week of life. The second pivot point exists within the relationship between the recipient female and the embryo transfer derived progeny that she produces. In this relationship, there is no genetic relationship between the recipient and the transferred implanted embryo offspring, but there is complete (or at least partial) health status linkage. This implies that by intentionally selecting recipientsof a specific health status, the breeder may thereby select the desired health status of the offspring she will produce. By putting these two pivot points together within an integrated production system, embryo transfer permits cervid breeders to intentionally, sustainably, and simultaneously manipulate both the genetic composition and health status of animals under selection.
Multiple Ovulation and Embryo Transfer Systems
Multiple ovulation and embryo transfer systems involve a three-step process for the in vivo production and recovery of embryos. The initial step in the MOET process involves the synchronization and superovulation of donor females. Superovulation is followed by the breeding of donor females (either by natural cover or artificial insemination) to allow for in vivo fertilization of oocytes. The final step of in vivo embryo production encompasses the recovery of embryos from the donor’s uterus or oviducts. MOET has been applied to both red deer and wapiti over the past two decades. Even though red deer and wapiti are part of the same genetic family (Cervus elaphus) their response to MOET treatment, and particularly their response to gonadotropin-stimulated superovulation, differs significantly.5 Superovulation is the process by which females are stimulated with exogenous gonadotropins to increase their ovulation rate above that normally occurring in the species under investigation. During a typical estrous cycle both red deer and wapiti cows normally ovulate only a single oocyte. Superovulation in these species would thus be defined as any treatment that would increase the ovulation rate above a single oocyte. A limited number of experimental studies have addressed superovulation in either red deer hinds or wapiti cows. These studies have demonstrated that while red deer will respond at moderate rates to superovulation treatment with commercially available purified gonadotropin, wapiti cows do not.5,6 This difference is intriguing because red deer and wapiti interbreed readily, have very similar reproductive patterns, and are considered to be members of the same species.6 Although earlier attempts to superovulate wapiti utilizing impure preparations of follicle-stimulating hormone (FSH) and miniosmotic pumps were at times successful,6,7 more recent attempts involving highly purified FSH preparations currently available have met with failure. Wapiti cows treated with purified FSH preparations appear not to respond to exogenous gonadotropin either in the growth of supernumerary follicles or in the ovulation of multiple oocytes. The nonresponsiveness of wapiti to superovulatory treatments may be based either in the biologic incompatibility of commercially available gonadotropin hormones with wapiti FSH receptors, or in yet undiscovered powerful intraovarian control mechanisms that inhibit simultaneous growth in follicular cohorts to ovulatory stages in mature wapiti. Whatever the underlying mechanism, the inability to superovulate wapiti cows has been the primary limiting factor in the development of MOET in farmed wapiti. Of interest to the application of advanced embryo biotechnologies in wapiti is our recent finding that juvenile wapiti donor calves (3- to 4-month-old calves) are responsive to gonadotropin treatment, averaging 7.3 and 15.7 cumulus-oocyte complexes recovered per control and superstimulated wapiti calf, respectively.
In red deer, early superovulation protocols included equine chorionic gonadotropin (eCG) within the treatment regimen, and more modern methodologies have made use of highly purified FSH alone (purified ovine FSH [oFSH, Ovagen, ICP, Inc., Auckland, NZ] has become the commercial gonadotropin of choice in red deer). A synchronization and superovulation protocol that has been extensively tested by our clinical group in the commercial production of red deer embryos in presented in Table 136-1. In brief, the first controlled intravaginal drug release (CIDR-G; Interag, Hamilton, NZ) device is inserted into the vagina of each donor hind on day 0 of treatment. On the ninth day of treatment the initial CIDR-G is replaced by a new CIDR-G in conjunction with the initiation of FSH treatment. The total FSH dosage used for superovulation in red deer is dependent upon the genetic background of the donors in question. English strains are generally very responsive to gonadotropin stimulation, but eastern European strains are not. For English strains a total dose of 0.4 to 0.5 unit Ovagen is commonly prescribed, but a full 1.0 unit of oFSH (Ovagen) may be required for German, Croatian, or Romanian lines. Regardless of the strain in question, FSH is administered to each donor in eight equal doses or in a declining dose regimen. Injections are administered at 12-hour intervals over a 4-day period. On day 11 of treatment, the second CIDR-G is removed in conjunction with the administration of the sixth FSH injection. Following the final injection of FSH on day 12, donor hinds either are placed with selected stags for natural cover or are artificially inseminated. Although natural cover provides some advantages in terms of maximizing sperm number and viability, it is disadvantaged by the limited selection intensity afforded by resident stags on individual farms, by the number of hinds that can be covered by an individual stag, and by the tendency of red deer stags to focus their attention on individual favored hinds while leaving other unfavored hinds unbred. Alternatively, superovulated red deer hinds may be artificially inseminated using either laparoscopic or transcervical methods. The use of artificial insemination affords significant benefits to breeders, including increased intensity and variety of stag selection, the ability to breed large numbers of hinds to individual stags, and the capacity to assure semen deposition in the uterus of each treated hind. Although laparoscopic insemination has been the traditional technique of choice in red deer (and may still be necessary for smaller yearling hinds), highly effective transcervical insemination techniques have recently been developed in red deer (see Chapter 134).5 In previous studies, laparoscopic insemination of superovulated hinds was found to decrease both fertilization and transferable embryo recovery rates when compared to naturally covered hinds. In contrast, we have found a single timed (48 hours after CIDR removal) transcervical insemination to be highly effective. Table 136-2 presents embryo production data from red deer donors superovulated utilizing the protocol presented in Table 136-1 and inseminated transcervically with a single dose of cryopreserved red deer semen 48 hours after removal of the second CIDR device. Asingle timed transcervical insemination allows for a minimum of donor handling and does not require either general anesthesia or abdominal invasion. Overall, fertilization was observed in 89% of the transcervically inseminated superovulated donors with embryo production averaging 4.6 transferable embryos per donor.
Table 136-1 Superovulation Protocol for Red Deer Hinds Utilizing a Single Timed Transcervical Insemination
Treatment Day | Treatment |
---|---|
Day 0 | Insert CIDR-G |
Day 9 | Replace CIDR-G; AM and PM injections of oFSH* |
Day 10 | AM and PM injections of oFSH |
Day 11 | CIDR-G removal; AM and PM injections of oFSH |
Day 12 | AM and PM injections of oFSH |
Day 13 | Artificial insemination at 48 h after CIDR removal |
Day 20 | Embryos flushed 228 h after CIDR removal |
CIDR, controlled intravaginal drug release; FSH, follicle-stimulating hormone.
* Ovagen (total dosage dependent upon genetic strain).
Table 136-2 In Vivo Embryo Production in Superovulated Red Deer Hinds Bred by a Single Timed Transcervical Insemination
Factor | Number and Response |
---|---|
Total number of donors | 51 |
Total number of donors responding* | 45 |
Total number of donors with fertilized embryos | 40 |
Total number of recovered embryos | 346 |
Mean number of embryos recovered per donor | 7.68 |
Total number of transferable embryos† recovered | 209 |
Mean number of transferable embryos† recovered per donor | 4.64 |
* Donors with 1 or more ovulations.
† Grade 1 morulae and blastocysts.
Embryos may be recovered from red deer hinds utilizing either surgical or transcervical flushing methodologies and commercial media. Surgical flushing has been effectively employed in red deer for several decades and remains the procedure of choice for use with small yearling hinds or in programs that demand oviductal stage embryos. Transcervical flushing methods have now been developed for red deer, which are equally efficacious to surgical procedures (Table 136-3). Transcervical procedures may soon displace surgical methods as they are significantly less stressful on donor animals and do not require general anesthetics.
Table 136-3 Comparison of Embryo Recovery Rates Following Either Surgical or Transcervical Flushing of Superovulated Red Deer Hinds
Feature | Surgical Recovery | Transcervical Recovery |
---|---|---|
Total number of donors | 19 | 26 |
Total number of recovered embryos | 144 | 202 |
Mean number of embryos recovered per donor | 7.58 | 7.77 |
Total number of transferable embryos* recovered | 100 | 109 |
Mean number of transferable embryos* recovered per donor | 5.26 | 4.19 |
Total number of corpora lutea† | 197 | 252 |
Recovery rate (%) | 73.1 | 80.2 |
* Grade 1 morulae and blastocysts
† Corpora lutea number determined by visual observation in surgical recoveries and by rectal palpation in nonsurgical recoveries.
The surgical recovery of red deer embryos is accomplished using methods similar to those used for other small domestic ruminants. Surgical flushing is conducted under general anesthesia (water and feed are removed from donor hinds for at least 12 hours prior to surgery) with the reproductive tract exteriorized through a midventral laparotomy. A very effective general anesthetic protocol that has proved to be exceptionally safe for use with both red deer and wapiti is the mixture of xylazine (Bayer AG, Germany) and carfentanil citrate (Wildife Pharmaceuticals, Inc., Fort Collins, CO, US).6 The mixture of xylazine and carfentanil is administered as an intramuscular injection and is reversed by an intravascular injection of tolazine hydrochloride (Lloyd Laboratories, Shenandoah, IA) and naltrexone hydrochloride (Wildlife Pharmaceuticals, Inc., Fort Collins, CO, US). Once exteriorized, the lumen of each uterine horn is cannulized using a silicon molded Foley catheter (12 F for yearling hinds; 14 F for mature hinds). The catheter is inserted through a blunt puncture made at the base of each uterine horn with the catheter’s balloon subsequently inflated with 4 to 7 ml of air or saline. The endometrium of red deer is quite friable and great care must be taken not to split or damage this tissue during either balloon inflation or medium infusion. With the Foley catheter secured, the infundibulary os is cannulated using a tom-cat catheter attached to a 10-ml syringe. Medium is then gently flushed through the length of the oviduct into the uterus and out the Foley catheter into a collecting dish. As the red deer oviduct is a rather delicate structure, flush medium should be gently pulsed through the tom-cat catheter so as not to rupture the ampullary wall. After the oviduct is flushed with 10 ml of medium, a blunted 20-gauge needle is passed through the uterine wall just caudal to the uterotubal junction. Another 40 ml of medium is then flushed through the cannulated uterine horn. The entire procedure is repeated on the contralateral oviduct and uterine horn. Puncture sites where Foley catheters have been passed through the uterine wall must be secured with inverting sutures, or endometritis may result. The exteriorized reproductive tract should be continually irrigated with warmed saline to reduce the incidence of surgically induced adhesions.
Nonsurgical embryo recovery was initially developed and tested by our working group during the 1999 breeding season. The evolved procedure is identical to transcervical methods ubiquitously used in bovine embryo recovery, save for the size and composition of the flushing catheter and requisite size of the operator’s hands. Essential to the recent development of transcervical methods for artificial insemination, embryo recovery, and embryo transfer in red deer was the entrance of highly creative female clinicians possessing diminutive hands talented in palpation. Nonsurgical embryo recovery in red deer is accomplished using a 14-gauge metal flushing catheter passed transcervically into the uterine lumen under the guidance of rectal palpation. After the catheter’s balloon is carefully inflated at the base of the respective uterine horn, flush fluid is injected and then withdrawn from the uterine lumen in a manner similar to that used in cattle. A total of 500 ml of medium is flushed per uterine horn. An epidural anesthetic is used to facilitate passage of the flushing catheter through the cervix. As transcervical procedures access the uterine lumen alone, only embryos at or beyond the compact morula stage can be recovered by these procedures. This is generally adequate for commercial embryo recovery programs, however, as compact morulae and blastocysts are the embryonic stages of choice for cryopreservation and transfer. In direct comparisons, transcervical embryo recovery methods have been shown to be as effective and productive as traditional surgical procedures (see Table 136-3) when flushing 9.5 days following CIDR device removal.
Methods for the in vivo production of embryos in fallow deer have also been developed.8 Embryo production from MOET technologies in fallow deer appear intermediate to that of red deer and wapiti. Although fallow deer appear more responsive than wapiti to superovulation, the production of embryos following gonadotropin treatment falls significantly short of that obtained in red deer. Embryo recovery rates have averaged 2.1 embryos per superovulated doe.8 Superovulation protocols have often included the addition of a low dose of eCG (100–200 IU) to the purified oFSH treatment. Fallow does may be laparoscopically inseminated using a single dose of semen, with embryos recovered surgically using procedures similar to that described previously for red deer. Recovered embryos are transferred to synchronized does using the laparoscopically based minilaparotomy method. Pregnancy rates following the transfer of fresh embryos in fallow deer have been similar to those obtained in red deer. Transcervical embryo recovery and transfer methods have not been developed to clinically productive states in fallow deer due to their diminutive size.
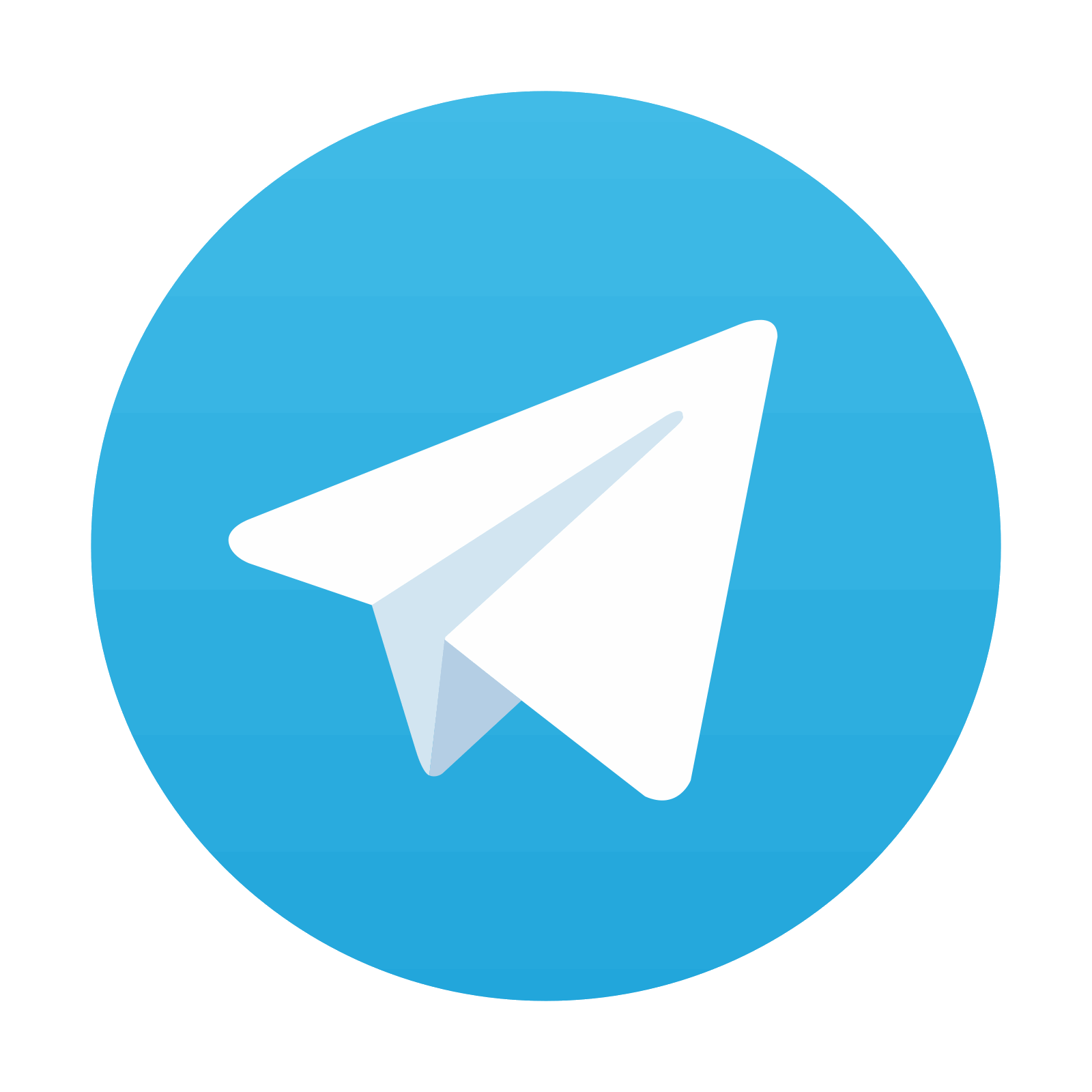
Stay updated, free articles. Join our Telegram channel
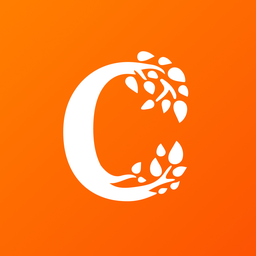
Full access? Get Clinical Tree
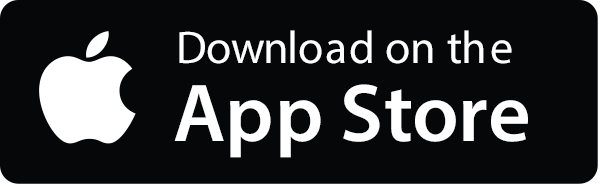
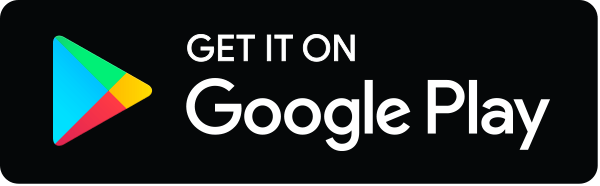