Cardiovascular anatomy and physiology
The uptake, distribution, and elimination of anesthetic drugs depend on blood flow. The cardiovascular system, which is composed of the heart, blood vessels, lymph vessels, and blood, is designed to supply a continuous flow of blood to all tissues of the body.
Heart
The heart is composed of four chambers: two thin-walled atria separated by an interatrial septum, and two thick-walled ventricles separated by an interventricular septum. The atria receive blood returning from the systemic circulation (right atrium [RA]) and pulmonary circulation (left atrium [LA]), and to a limited degree act as storage chambers. The ventricles, the major pumping chambers of the heart, are separated from the atria by the tricuspid valve on the right side and the mitral valve on the left side. The ventricles receive blood from their respective atria and eject it across semilunar valves (the pulmonic valve between the right ventricle [RV] and pulmonary artery and the aortic valve between the left ventricle [LV] and aorta) into the pulmonary circulation and systemic circulation, respectively.
Once the process of cardiac contraction is initiated, almost simultaneous contraction of the atria is followed by nearly synchronous contraction of the ventricles, which results in pressure differences between the atria, ventricles, and pulmonary and systemic circulations. Cardiac contraction produces differential pressure changes that are responsible for atrioventricular (AV) and semilunar valve opening and closing and the production of heart sounds. Chordae tendineae originating from papillary muscles located on the inner wall of the ventricular chambers are attached to the free edges of the AV valve leaflets and help to maintain valve competence and prevent regurgitation of blood into the atrium during ventricular contraction. Alteration in heart chamber geometry (e.g., stretch or hypertrophy) produced by changes in blood volume, deformation (pericardial tamponade), or disease can have profound effects on myocardial function, as do the effects produced by neurohumoral, metabolic, and pharmacological perturbations.
Blood vessels
The large and small vessels of the pulmonary and systemic circulations facilitate the delivery of blood to the exchange sites in the pulmonary and systemic capillary beds and return blood to the heart. The aorta and other large arteries compose the high-pressure portion of the systemic circulation and are relatively stiff compared to veins, possessing a high proportion of elastic tissue in comparison to smooth muscle and fibrous tissues. The flow of blood to peripheral tissues throughout the cardiac cycle (contraction – relaxation – rest) has been termed the Windkessel effect. The Windkessel effect is believed to be responsible for as much as 50% of peripheral blood flow in most species during normal heart rates (HRs). Tachyarrhythmias and vascular diseases (stiff nonelastic vessels) hamper the Windkessel effect and produce distinctive changes in the arterial pressure waveform. More distal larger arteries contain greater percentages of smooth muscle compared to elastic tissue and act as conduits for the transfer of blood under high pressure to tissues. The most distal small arteries, terminal arterioles, and arteriovenous anastomoses contain a predominance of smooth muscle, are highly innervated, and function as resistors that regulate the distribution of blood flow, aid in the regulation of systemic blood pressure (BP), and modulate tissue perfusion pressure. The capillaries are the functional exchange sites for oxygen, nutrients, electrolytes, cellular waste products, and other substances. Capillaries are of three different types: continuous (lung and muscle), fenestrated (kidney and intestine), and discontinuous (liver, spleen, and bone marrow).
Postcapillary venules are composed of an endothelial lining and fibrous tissue and function to collect blood from capillaries. Some venules act as postcapillary sphincters, and all venules merge into small veins. Small and larger veins contain increasing amounts of fibrous tissue in addition to smooth muscle and elastic tissue, although their walls are much thinner than comparably sized arteries. Many veins contain valves that act in conjunction with external compression (contracting muscles and pressure differences in the abdominal and thoracic cavities) to facilitate venous return of blood to the RA. The venous system also acts as a major blood reservoir. Indeed, 60 – 70% of the blood volume may be stored in the systemic venous vasculature during resting conditions (Figure 2.1).
Two additional structural components that are important during normal circulatory function are arteriovenous anastomoses and the lymphatic system. Arteriovenous anastomoses bypass capillary beds. They possess smooth muscle cells throughout their entire length and are located in most, if not all, tissue beds. Most arteriovenous anastomoses are believed to be important in regulating blood flow to highly vascular tissue (skin, feet, and hooves). Their role in maintaining normal homeostasis, however, is speculative other than for thermoregulation.
Figure 2.1.The cardiovascular system is comprised of the heart, blood, and two parallel circulations (pulmonary and systemic). Pulmonary circulation: The pulmonary artery carries blood from the right ventricle (RV) to the lungs, where carbon dioxide is eliminated and oxygen is taken up. Oxygenated blood returns to the left atrium (LA) via the pulmonary veins. Systemic circulation: Blood is pumped by the left ventricle (LV) into the aorta, which distributes blood to the peripheral tissues. Oxygen and nutrients are exchanged for carbon dioxide and other by-products of tissue metabolism in capillary beds, after which the blood is returned to the right atrium (RA) through the venules and large systemic veins.
Sources: Modified from Shepherd J.T., Vanhoutte P.M. 1979. The Human Cardiovascular System; Facts and Concepts, 1st ed. New York: Raven; and Muir W.W. 2007. Cardiovascular system. In: Lumb and Jones’ Veterinary Anesthesia and Analgesia, 4th ed. W.J. Tranquilli, J.C. Thurmon, and K.A. Grimm, eds. Ames, IA: Blackwell Publishing, Ames, IA, p. 62.

The peripheral lymphatic system is not anatomically part of the blood circulatory system. Nevertheless, it is integrally involved in maintaining normal circulatory dynamics, especially interstitial fluid volume (approximately 10% of the capillary filtrate). Lymphatic capillaries collect interstitial fluid—lymph—which is eventually returned to the cranial vena cava and RA after passing through a series of lymph vessels, lymph nodes, and the thoracic duct. Lymph vessels have smooth muscle within their walls and contain valves similar to those in veins. Contraction of skeletal muscle (lymphatic pump) and lymph vessel smooth muscle, in conjunction with lymphatic valves, are responsible for lymph flow.
Blood
Blood is a suspension of red (erythrocytes) and white (leukocytes) blood cells and platelets (thrombocytes) in plasma. The most essential function of blood is to deliver oxygen to tissues. Oxygen is relatively insoluble in plasma (0.003 mL oxygen per 100 mL blood per 1 mm Hg partial pressure of oxygen [PO2]; approximately 0.3mL oxygen per 100 mL blood at PO2 = 100 mm Hg). The erythrocytes transport much larger amounts of oxygen than can be carried in solution, and functionally the amount that can be carried depends on the amount of hemoglobin (Hb) in the erythrocytes. The affinity of Hb for oxygen depends on the partial pressure of carbon dioxide (PCO2), pH, body temperature, the intraerythrocyte concentration of 2,3-diphosphoglycerate, and the chemical structure of Hb (Figure 2.2). Once the amount of deoxygenated Hb (unsaturated Hb) exceeds 5 g/100 mL of blood, the blood changes from a bright red to a purple-blue color (cyanosis). Some of the carbon dioxide produced by metabolizing tissues binds to deoxygenated Hb and is eliminated by the lungs during the Hb oxygenation process prior to the blood returning to the systemic circulation and the cycle repeating itself.
Figure 2.2.The oxyhemoglobin dissociation curve illustrates the relationship between the blood partial pressure of oxygen (PO2) and the saturation of hemoglobin (Hb) with oxygen (O2). Note that this curve is shifted to the right (Hb has less affinity for O2) by acidosis, increased body temperature, and the enzyme 2,3-diphosphoglycerate (2,3-DPG). This effect helps to unload O2 from Hb in tissues and increases the Hb affinity for O2 in the lungs. The total arterial oxygen content (CaO2) is determined by the total blood Hb concentration, its percent saturation (%SaO2), and the PaO2.
Source: Muir W.W. 2007. Cardiovascular system. In: Lumb and Jones’ Veterinary Anesthesia and Analgesia, 4th ed. W.J. Tranquilli, J.C. Thurmon, and K.A. Grimm, eds. Ames, IA: Blackwell Publishing, p. 64.

Maintaining adequate tissue oxygenation depends on oxygen uptake by the lungs, oxygen delivery (DO2) to and oxygen extraction (OE) by tissues, and oxygen use by the metabolic machinery within cells. The factors that determine the supply of oxygen to tissues are Hb concentration, the affinity of Hb for oxygen (P50), the saturation of Hb with oxygen (SaO2), the arterial oxygen partial pressure (PaO2), the cardiac output (CO), and the tissue oxygen consumption (VO2). The Fick equation (VO2 = CO [CaO2 − CvO2]) contains all the essential components of this relationship. Arterial blood oxygen content (CaO2) is calculated by CaO2 = Hb × 1.35 × SaO2 + (PaO2 × 0.003). Arterial blood (Hb = approximately 15g/dL at packed cell volume [PCV] = 45%), for example, contains approximately 20–21 mL of oxygen/dL of blood when the SaO2 = 100% and the PaO2 = 100 mm Hg (room air). The venous blood oxygen content (CvO2) is generally 14–15 mL/dL, yielding an OE ratio of 0.2–0.3 (20–30%). An increase in arterial blood lactate concentration is the cardinal sign of inadequate oxygen delivery to metabolizing tissues and suggests that oxygen consumption has become delivery dependent or that some defect in tissue OE or use has developed.
Pressure, resistance, and flow
In electric circuits, current flow (I) is determined by the electromotive force or voltage (E) and the resistance to current flow (R); according to Ohm’s law:
The flow of fluids (Q) through nondistensible tubes depends on pressure (P) and the resistance to flow (R). Therefore, Q = P/R.
The resistance to blood flow is determined by blood viscosity (η) and the geometric factors of blood vessels (radius and length). The steady, nonpulsatile, laminar flow of Newtonian fluids (homogenous fluids in which viscosity does not change with flow velocity or vascular geometry), like water, saline, and, under physiological conditions, plasma, can be described by the Poiseuille–Hagen law, which states:
where P1 − P2 is the pressure difference, r4 is the radius to the fourth power, L is the length of the tube, η is the viscosity of the fluid, and π/8 is a constant of proportionality. The maintenance of laminar flow is a fundamental assumption of the resistance offered to steady-state fluid flow in the Poiseuille – Hagen equation.
The relationship between vessel (or chamber when describing the heart)-distending pressure, vessel diameter, vessel wall thickness, and vessel wall tension is described by Laplace’s law:
where T is wall tension, P is developed pressure, r is the internal radius, and h is the wall thickness. This relationship is important because it relates pressure and vessel dimension to changes in developed tension, which is known to be an important determinant of ventricular–vascular coupling (afterload), myocardial work, and myocardial oxygen consumption.
Blood pressure
BP in arteries, whether measured directly or indirectly, is frequently assessed during anesthesia. Arterial BP measurement is one of the fastest and most informative means of assessing cardiovascular function and provides an accurate indication of drug effects, surgical events, and hemodynamic trends.
The factors that determine arterial BP are HR, stroke volume (SV), vascular resistance, arterial compliance, and blood volume. Mean arterial BP is a key component in determining tissue perfusion pressure and the adequacy of tissue blood flow. Perfusion pressures greater than 60 mm Hg are generally thought to be adequate for perfusion of tissues. Structures like the heart (coronary circulation), lungs (pulmonary circulation), kidneys (renal circulation), and the fetus (fetal circulation) contain special circulations where changes in perfusion pressure can have immediate effects on organ function. Clinically, arterial BP is generally measured as mean arterial pressure. When mean arterial BP cannot be directly assessed, it is estimated by this formula:
where Pm, Ps, and Pd are mean (m), systolic (s), and diastolic (d) BPs, respectively (Figure 2.3). Both Ps and P d can be measured (estimated) indirectly using either Doppler or oscillometric techniques. Most drugs used to produce anesthesia decrease CO and peripheral vascular resistance. However, vasoconstricting drugs (e.g., alpha2 adrenergic agonists) can increase peripheral vascular resistance and maintain BP in physiological ranges while dramatically decreasing CO and blood flow to certain tissues (e.g., skin and skeletal muscle) (Figure 2.4).
The arterial pulse pressure (Ps – Pd) and pulse-pressure waveform analysis can provide valuable information regarding changes in vascular compliance and vessel tone. Generally, drugs (phenothiazines) or diseases (endotoxic shock) that produce marked arterial dilation increase vascular compliance, causing a rapid rise, short duration, and rapid fall in the arterial waveform while increasing the arterial pulse pressure. Situations that produce vasoconstriction decrease vascular compliance, producing a longer duration pulse waveform and a slower fall in the systolic BP to diastolic values. The pulse pressure may contain secondary and sometimes tertiary pressure waveforms, particularly if the measuring site is in a peripheral artery some distance from the heart.
Nervous, humoral, and local control
Regulation of the cardiovascular system is integrated through the combined effects of the central and peripheral nervous systems, the influence of circulating (humoral) vasoactive substances, and local tissue mediators that modulate vascular tone. These regulatory processes maintain blood flow at an appropriate level while distributing blood flow to meet the needs of tissue beds that have the greatest demand.
Figure 2.3. Arterial BP is determined by both physiological and physical factors. The mean arterial pressure (Pm) represents the area under the arterial pressure curve divided by the duration of the cardiac cycle and can be estimated by adding one-third the difference between the systolic arterial pressure (Ps) and diastolic arterial pressure (Pd) to Pd. Ps minus Pd is the pulse pressure.
Sources:Modified from Berne R.M., Levey M.N. 1990. Principles of Physiology, 1st ed. St. Louis, MO: Mosby; and Muir W.W. 2007. Cardiovascular system. In: Lumb and Jones’ Veterinary Anesthesia and Analgesia, 4th ed. W.J. Tranquilli, J.C. Thurmon, and K.A. Grimm, eds. Ames, IA: Blackwell Publishing, p. 92.

Cardiac electrophysiology
Normal cardiac electrical activity is essential for normal cardiac contractile function (excitation–contraction coupling). The cardiac cell membrane (sarcolemma) is a highly specialized lipid bilayer that contains protein-associated channels, pumps, enzymes, and exchangers in an architecturally sophisticated, yet fluid (reorganizable and movable), medium. Most drugs and many anesthetic drugs produce important direct and indirect effects on the cell membrane and intracellular organelles, ultimately altering cardiac excitation-contraction coupling (Figure 2.5).
Figure 2.4. CO is equal to heart rate (HR) times stroke volume (SV), or arterial blood pressure (BP) divided by peripheral vascular resistance (PVR). Increases in HR, cardiac contractility, and preload, and decreases in afterload can all increase CO. Preload and afterload are considered to be coupling factors because they depend on vascular resistance, capacitance, and compliance.
Source: Muir W.W. 2007. Cardiovascular system. In: Lumb and Jones’ Veterinary Anesthesia and Analgesia, 4th ed. W.J. Tranquilli, J.C. Thurmon, and K.A. Grimm, eds. Ames, IA: Blackwell Publishing, p. 81.

The cardiac pacemaker (sinoatrial or SA node) normally suppresses the automaticity of slower or subsidiary pacemakers (overdrive suppression), preventing more than one pacemaker from controlling HR. Initiation of an electric impulse in the SA node is followed by rapid electrochemical transmission of the impulse through the atria, giving rise to the P wave. Repolarization of the atria gives rise to the Ta wave, which is most obvious in large animals (horses and cattle), where the total atrial tissue mass is substantial enough to generate enough electromotive force to be electrocardiographically recognizable. Repolarization of the atria in smaller species (dogs and cats) and depolarization of the SA and AV nodes do not generate a large enough electric potential to be recorded at the body surface except in some cases of sinus tachycardia. Once the wave of depolarization reaches the AV node, conduction is slowed because of the AV node’s low resting membrane potential. Increased parasympathetic tone can produce marked slowing of AV nodal conduction, leading to first-degree, second-degree, and, rarely, third-degree heart block. Many drugs used in anesthesia, including opioids, alpha2 adrenergic agonists, and occasionally acepromazine, increase the parasympathetic tone, predisposing patients to heart block and bradyarrhythmias. The use of antimuscarinic drugs such as atropine and glycopyrrolate is generally effective therapy in these situations unless the block is caused by structural disease (e.g., inflammation, fibrosis, or calcification).
Under normal conditions, conduction of the electric impulse through the AV node produces the PR or PQ interval of the electrocardiogram (ECG) and provides time for the atria to contract prior to activation and contraction of the ventricles. This delay is functionally important, particularly at faster HRs, because it enables atrial contraction to contribute to ventricular filling. Once the electric impulse has traversed the AV node it is rapidly transmitted to the ventricular muscle by specialized muscle cells commonly referred to as Purkinje fibers. Bundles of Purkinje cells—the right and left bundle branches—transmit the electric impulses to the ventricular septum and the right and left ventricular free walls, respectively. Their distribution accounts for differences in the pattern of the ECG (ventricular depolarization) among species. Purkinje fibers have much longer action potentials and refractory periods than do ventricular muscle cells, which normally prevents reentry of the electric impulse and reactivation of the ventricles.
Figure 2.5. The cardiac cycle diagrammatically illustrates the relationship between mechanical, acoustical, and electrical events as a function of time. Isovol. contract., isovolumetric contraction; Isovol. relax, isovolumetric relaxation.
Sources: Modified from Berne R.M., Levey M.N. 1990. Principles of Physiology, 1st ed. Louis, MO: Mosby; and Muir W.W. 2007. Cardiovascular system. In: Lumb and Jones’ Veterinary Anesthesia and Analgesia, 4th ed. W.J. Tranquilli, J.C. Thurmon, and K.A. Grimm, eds. Ames, IA: Blackwell Publishing, p. 78.

The configuration and magnitude of the T wave vary considerably among species and are influenced by changes in HR, temperature, and the extracellular potassium concentration. Hyperkalemia, for example, produces an increase in membrane conductance to potassium. This shortens repolarization and produces T waves that are of large magnitude, generally spiked or pointed, and of short duration (short QT interval).
The interval beginning immediately after the S wave of the QRS complex (J point) and preceding the T wave is referred to as the ST segment and is important clinically. Elevation or depression of the ST segment (±0.2 mV or greater) from the isoelectric line is usually an indication of myocardial hypoxia or ischemia, low CO, anemia, pericarditis, or cardiac contusion, and suggests the potential for arrhythmia development.
Determinants of performance and output
Clinically, M-mode and color-flow Doppler echocardiography are used to assess ventricular function. These techniques provide a dynamic temporal representation of cardiac function and, when coupled with hemodynamic computer software analysis systems, a pictorial and quantitative assessment of cardiac performance.
The oxygen requirements of tissues are met by the continuous adjustment of CO, which is the product of HR and SV:
SV is the amount of blood ejected from the ventricle during contraction and therefore represents the difference between the end-diastolic and end-systolic ventricular volumes.
Preload
Preload is usually explained in terms of the Frank–Starling relationship or as heterometric autoregulation: increases in myocardial fiber length (ventricular volume) increase the force of cardiac contraction and CO. Whether or not individual sarcomeres actually increase in length (stretch) with increases in ventricular volume is controversial. Because of the difficulty in accurately determining ventricular volume in the clinical setting, ventricular diameter, ventricular end-diastolic pressure, pulmonary capillary wedge pressure, and, occasionally, mean atrial pressure are used as estimates of preload. The substitution of pressure for volume, although common, must be done with the understanding that there are many instances (open-chest procedures and stiff or noncompliant hearts) when pressure does not accurately represent changes in ventricular volume and therefore is not an accurate index of preload.
Afterload
The term afterload is used throughout the basic and clinical cardiology literature to describe the force opposing ventricular ejection. One major reason for the great interest in this physiological determinant of cardiac function is its inverse relationship with SV and its direct correlation with myocardial oxygen consumption. Afterload changes continuously throughout ventricular ejection and is more accurately described by the tension (stress) developed in the left ventricular wall during ejection or as the arterial input impedance (Zj). Ventricular wall stress or tension has traditionally been estimated from the Laplace relationship:
It is noteworthy that using this assessment of ventricular afterload assumes a spherical ventricular geometry.
The measurement of systemic vascular resistance (SVR) is used clinically as a measure of afterload and vascular tone because it is technically simple to obtain and intuitively easier to understand.
Inotropy
Cardiac contractility (inotropy) is the intrinsic ability of the heart to generate force. A decrease in cardiac contractility is a key factor in heart failure in patients with cardiac disease or following the administration of potent negative inotropic drugs (e.g., inhalant anesthetics). Ideal indexes of cardiac contractility should be independent of changes in HR, preload, afterload, and cardiac size—in other words, be load independent.
Lusitropy
A description of the relaxation phases following cardiac contraction is often omitted from textbooks of cardiovascular physiology, but is fundamentally important to an understanding of cardiac performance. Mechanical factors, loading factors, inotropic activity, HR, and asynchronicity (patterns of relaxation) are the major determinants of lusitropy.
Maintenance of adequate respiratory function is a requirement for successful anesthesia. Inadequate tissue oxygenation at a severe level may lead to acute death. Excessive elevations in arterial carbon dioxide (CO2) tensions (arterial CO2 partial pressure [PaCO2]) or sustained moderate hypoxemia may produce some level of organ dysfunction, which contributes to a less than optimal anesthetic recovery.
During general anesthesia, there is always a tendency for arterial oxygen tensions (PaO2) to be less than observed with the same species while conscious and breathing the same fraction of inspired oxygen concentration (FiO2). There is also a tendency for PaCO2 to be elevated above the conscious resting values if the anesthetized animal is breathing spontaneously, and for increases in airway resistance to occur unless an endotracheal tube is used. Some differences are seen, depending on the actual anesthetic regimen used, but the depth of anesthesia is often more of a factor.
Definitions
Respiration is the overall process whereby oxygen is supplied to and used by body cells and carbon dioxide is eliminated by means of partial pressure gradients. Ventilation is the movement of gas into and out of alveoli. The ventilatory requirement for homeostasis varies with the metabolic requirement of animals, and it thus varies with body size, level of activity, body temperature, and depth of anesthesia. Inadequate ventilation to meet the gas exchange requirements of metabolism is termed respiratory depression or hypoventilation. It is manifested clinically as an increase in PaCO2 and a respiratory acidosis. When breathing high FiO2, Hb saturation does not decrease unless hypoventilation is severe. Pulmonary ventilation is accomplished by thoracic cavity expansion and elastic contraction of the lungs. Several terms are used to describe the various types of breathing patterns that may be observed:
(1). Eupnea is ordinary quiet breathing.
(2). Dyspnea is labored breathing.
(3). Tachypnea is increased respiratory rate.
(4). Hyperpnea is fast and/or deep respiration, indicating “overrespiration.”
(5). Polypnea is a rapid, shallow, panting type of respiration.
(6). Bradypnea is slow regular respiration.
(7). Hypopnea is slow and/or shallow breathing, possibly indicating “underrespiration.”
(8). Apnea is transient (or longer) cessation of breathing.
(9). Cheyne-Stokes respirations increase in rate and depth, and then become slower, followed by a brief period of apnea.
(10). Biot’s respirations are sequences of gasps, apnea, and several deep gasps.
(11). Kussmaul’s respirations are regular deep respirations without pause.
(12). Apneustic respiration occurs when an animal holds an inspired breath at the end of an inhalation for a short period before exhaling. Apneustic breathing is commonly seen with dissociative anesthetic administration (e.g., ketamine or Telazol®).
To describe the events of pulmonary ventilation, air in the lungs has been subdivided into four different volumes and four different capacities (Figure 2.6). Only tidal volume (VT) and functional residual capacity (FRC) can be measured in conscious uncooperative animals:
(1) VT is the volume of air inspired or expired in one breath.
(2) Inspiratory reserve volume (IRV) is the volume of air that can be inspired over and above the normal VT.
(3) Expiratory reserve volume (ERV) is the amount of air that can be expired by forceful expiration after a normal expiration.
(4) Residual volume (RV) is the air remaining in the lungs after the most forceful expiration.
Figure 2.6. Lung volumes and capacities. TLC, FRC, and RV cannot be measured with spirometry. IRV, ERV, and IC are not included in the diagram.
Source: West J.B. 2001. Chronic obstructive pulmonary disease. In: Pulmonary Physiology and Pathophysiology: An Integrated, Case-Based Approach. Baltimore, MD: Lippincott Williams & Wilkins. Baltimore, p. 39.

Another term frequently used is the minute respiratory volume or minute ventilation (VE). This is equal to VT times the respiratory frequency (f). Occasionally, it is desirable to consider two or more of the aforementioned volumes together. Such combinations are termed pulmonary capacities:
(1) Inspiratory capacity (IC) is the VT plus the IRV. This is the amount of air that can be inhaled starting after a normal expiration and distending the lungs to the maximum amount.
(2) FRC is the ERV plus the RV. This is the amount of air remaining in the lungs after a normal expiration. From a mechanical viewpoint, at FRC the inward “pull” of the lungs due to their elasticity equals the outward “pull” of the chest wall.
(3) Vital capacity (VC) is the IRV plus the VT plus the ERV. This is the maximum amount of air that can be expelled from the lungs after first filling them to their maximum capacity.
(4) Total lung capacity (TLC) is the IRV plus the VT plus the ERV plus the RV, or the maximum volume to which the lungs can be expanded with the greatest possible inspiratory effort (or by full inflation to 30-cm H2O airway pressure when a patient is anesthetized).
Control of respiration
With the aid of the circulation, respiration maintains the oxygen, CO2, and pH of the cell. Respiratory function is controlled by central respiratory centers, central and peripheral chemoreceptors, pulmonary reflexes, and nonrespiratory neural input. The central neural “controller” includes specialized groups of neurons located in the cerebrum, brain stem, and spinal cord that govern both voluntary and automatic ventilation through regulation of the activity of the respiratory muscles. The respiratory muscles, by contracting, expand the chest cavity and produce alveolar ventilation (VA). Changes in VA affect blood gas tensions and hydrogen ion concentration. Blood gas tensions and hydrogen ion concentrations are monitored by peripheral and central chemoreceptors that return signals to the central controller to provide necessary adjustments in VA. Mechanoreceptors in the lungs and stretch receptors in the respiratory muscles monitor, respectively, the degree of expansion or stretch of the lungs and the “effort” of breathing, feeding back information to the central controller to alter the pattern of breathing. Adjustments also occur to accommodate nonrespiratory activities such as thermoregulation and vocalization.
Overall, this complex control system produces a combination of f and depth that is best suited for optimum ventilation with minimal effort for the particular species, and that adjusts oxygen supply and CO2 elimination so as to maintain homeostasis (reflected by stable arterial blood gas levels) over a wide range of environmental and metabolic situations. Sedatives, analgesics, anesthetics, and the equipment used for inhalational anesthesia may profoundly alter respiration and the ability of an animal to maintain cellular homeostasis.
The important factor in pulmonary ventilation is the rate at which alveolar gas is exchanged with atmospheric air. This is not equal to the alveolar minute ventilation volume because a large portion of inspired air is used to fill the respiratory passages (anatomic dead space, VDanat), rather than alveoli, and no significant gaseous exchange occurs in this air. The f and VT determine the VE. The “effective” volume, or portion of VT that contributes to gas exchange, is the alveolar volume, usually referred to as minute VA. Nonperfused alveoli do not contribute to gas exchange and constitute alveolar dead space (VDA). Physiological dead space (VD) includes VDanat and VDA, and is usually expressed as a minute value (VD) along with VA, or as a ratio of VD/VT (Figure 2.7).
Hypoxia refers to any state in which the PO2 in the lungs, blood, and/or tissues is abnormally low, resulting in abnormal tissue metabolism and/or cellular damage. Hypoxemia refers to insufficient oxygenation of blood to meet the metabolic requirement. In spontaneously breathing animals, hypoxemia is characterized by PaO2 levels lower than the normal for the species. Resting PaO2 levels in domestic species generally range from 80 to 100 mm Hg in healthy, awake animals at sea level. Some clinicians consider a PaO2 below 70 mm Hg (ca. 94% Hb saturation) as hypoxemia in animals at or near sea level, although the clinical significance of this degree of blood oxygen tension would vary depending on factors such as the health and age of an animal, Hb concentration, and the duration of low oxygen tension in relation to the rate of tissue metabolism (e.g., hypothermic patients would be at less risk).
Oxygen transport
Under normal conditions, oxygen is taken into the pulmonary alveoli and CO2 is removed from them at a rate that is sufficient to maintain the composition of alveolar air at a relatively constant concentration. In the lungs, gas is exchanged across both the alveolar and the capillary membranes. The total distance across which the exchange takes place is less than 1 |m; therefore, it occurs rapidly. Other than at high exercise levels, equilibrium almost develops between blood in the lungs and air in the alveolus, and the PO2 in the pulmonary venous blood almost equals the PO2 in the alveolus. While diffusion of oxygen across the alveolar-capillary space is a theoretical barrier to oxygenation, it is seldom a practical problem during veterinary anesthesia unless significant pulmonary edema or disease is present.
Figure 2.7. Schematic of uneven ventilation and blood flow. The alveolus on the left is ventilated, but not perfused, and thus is considered to be alveolar dead space, whereas the alveolus on the right is perfused, but not ventilated, and thus contributes to venous admixture or shunt flow. The center alveolus is perfused and ventilated equally and thus would have a V/Q ratio of 1.0. Relevant equations are shown, respectively, as Equations 1–4 for calculation of the dead space/VT ratio, the alveolar partial pressure of oxygen (PA02), the alveolar-to-arterial partial pressure of oxygen P(A – a)02 difference, and the venous admixture (Q/QT) fraction.
Source: Reproduced from Robinson NE. 1991. The respiratory system. In: Equine Anesthesia: Monitoring and Emergency Therapy. W.W. Muir and J.E. Hubbell, eds. St. Louis, M0: Mosby Year Book, pp. 7–38. With permission by Elsevier.

The normal average alveolar composition of respiratory gases in humans is listed below. At normal human body temperature, alveolar air is saturated with water vapor, which has a pressure of 48 mm Hg at 37°C. If the barometric pressure in the alveolus is 760 mm Hg (sea level), then the pressure due to dry air is 760–48 = 712 mm Hg. Knowing the composition of alveolar air, one can calculate the partial pressure of each gas in the alveolus:
The PO2 in the lungs at sea level is thus approximately 100 mm Hg at 37–38°C. Under these conditions, 100mL of plasma will hold 0.3 mL of oxygen in physical solution. Whole blood, under the same conditions, will hold 20 mL of oxygen, or about 60 times as much as plasma. CO2 is similarly held by blood. Thus, it is apparent that oxygen and CO2 in blood are transported largely in chemical combination, since both are carried by blood in much greater quantities than would occur if simple absorption took place. At complete saturation, each gram of Hb combines with 1.36–1.39mL of oxygen. This is the total carrying capacity of Hb, or four oxygen molecules combined with each Hb molecule. The ability of Hb to combine with oxygen depends on the PO2 in the surrounding environment. The degree to which it will become saturated at various PO2 values varies considerably. It is adjusted so that, even when ventilation is inefficient or the supply of oxygen is sparse at higher altitudes, the degree of saturation still approaches 100%. For instance, although it is probably not fully saturated until it is exposed to a PO2 of 250 mm Hg, Hb is approximately 94% saturated when the PO2 is only 70 mm Hg.
Carbon dioxide transport
Arterial CO2 levels are a function of both CO2 elimination and production, and under normal circumstances PaCO2 levels are maintained within narrow limits. During severe exercise, the production of CO2 is increased enormously, whereas during anesthesia, production likely decreases. Elimination of CO2 depends on pulmonary blood flow (CO) and VA. Normally, the production of CO2 parallels the oxygen consumption according to the respiratory quotient: R = VCO2/VO2. Although the value varies depending on the diet, usually R is 0.8 at steady state.
A CO2 pressure gradient, opposite to that of oxygen and much smaller, exists from the tissues to the atmospheric air: tissues = 50 mm Hg (during exercise, this may be higher); venous blood = 46 mm Hg; alveolar air = 40 mm Hg; expired air = 32 mm Hg; atmospheric air = 0.3 mm Hg; and arterial blood = 40 mm Hg (equilibrium with alveolar air). Carbon dioxide is carried from the mitochondria to the alveoli in a number of forms. In the plasma, some CO2 is transported in solution (5%), and some combines with water and forms carbonic acid, which in turn dissociates into bicarbonate and hydrogen ions (5%). Most (ca. 90%) of the CO2 diffuses into the red cells, where it is either bound to Hb or transformed (reversibly) to bicarbonate and hydrogen ions through the action of the enzyme carbonic anhydase. The formation of bicarbonate in the red blood cell is accompanied by the chloride shift (this accounts for approximately 63% of the total CO2 transport). Just as the amount of oxygen transported by the blood depends on the PO2 to which the blood is exposed, so is CO2 transport likewise affected; however, the CO2 dissociation curve is more or less linear. Thus, in contrast to the minimal effects on oxygen content, hyperventilation and hypoventilation may have marked effects on the CO2 content of blood and tissues.
Normal control mechanisms
As important as the detailed information referred to earlier is in helping us understand the respiratory adaptations to high altitude, disease, and exercise for the successful management of clinical anesthesia, a much simplified understanding of the control of respiration will suffice. In conscious animals, VE and VA are primarily determined by central chemoreceptor responsiveness to PaCO2 levels. The central chemoreceptors, located on the ventral surface of the medulla and bathed by cerebrospinal fluid (CSF), are exquisitely sensitive to changes in PaCO2 levels because CO2 is readily diffusible into CSF and the central chemoreceptor cell. The changes in PaCO2 are probably ultimately detected as a change in the pH within the chemoreceptor cell. A fall in arterial pH will also stimulate respiration through the central and peripheral chemoreceptors, as seen with metabolic acidosis. The peripheral chemoreceptors, which are located in the carotid and aortic bodies, generally play a significant part in respiratory drive only when PaO2 levels fall below 60 mm Hg.
The apneustic and pneumotaxic centers, and pulmonary and airway receptors, are primarily responsible for adjusting the balance between f and VT to achieve a given level of VA, usually in a way that minimizes the energy cost of breathing. Although the function of these receptors is generally not considered to be greatly influenced by the action of anesthetic and perianesthetic agents, they may play a part in some of the species differences we see in response to a particular drug or group of drugs.
Apneic threshold
The apneic threshold is the PaCO2 level where spontaneous ventilatory effort ceases. A PaCO2 reduction of 5–9 mm Hg from normal values through voluntary hyperventilation (a conscious human), or by artificial ventilation of sedated or anesthetized animals, produces apnea. The distance between the resting PaCO2 level and the apnea threshold is relatively constant (i.e., 5–9 mm Hg), irrespective of the anesthetic depth. Veterinary anesthetists use the apneic threshold to control respiration (i.e., abolish spontaneous efforts) when putting an animal on a ventilator, or to temporarily provide for a quiet surgical field without having to resort to the use of muscle relaxant drugs.
Drug effect on control of ventilation
Anesthetics and some perianesthetic drugs alter the central and peripheral chemoreceptor response to CO2 and oxygen in a dose-dependent manner. This has important clinical implications in terms of maintaining homeostasis during the perioperative period. There will also be a diminution in external signs in hypoxemic or hypercarbic anesthetized animals. Whereas unsedated animals usually demonstrate obvious tachypnea and an increase in VT or respiratory effort in response to serious hypoxemia or hypercapnia, these external signs of an impending crisis may well be absent or greatly diminished in anesthetized animals.
Inhalants and injectable drugs
All of the general anesthetic agents in current use produce a dose-dependent decrease in the response to CO2. With commonly used inhalant agents, the CO2 response is almost flat at a minimum alveolar concentration (MAC) of 2.0. The reduced sensory input and central sensitivity to CO2 produce a marked fall in VA, usually through a dose-related fall in VT, with f being reasonably well maintained. A proportional increase in VD/VT occurs because VDanat is more or less constant. As a result of these changes, PaCO2 levels increase as the anesthetic dose is increased when animals breathe spontaneously. In light anesthetic planes (e.g., MAC multiple of 1.2), PaCO2 will generally remain moderately elevated, but stable, over many hours of anesthesia, whereas at higher concentrations PaCO2 increases progressively over time. The degree of hypercarbia at equipotent doses of inhalant (and intravenous [IV]) anesthetic agents varies with the species and the degree of surgical stimulation.
Opioids
When given alone, opioids shift the CO2 response curve to the right with little change in slope, except at very high doses. This means that the resting PaCO2 level might be a little higher in an animal receiving a therapeutic dose of an opioid for premedication or postoperative recovery, but that the response to further CO2 challenge (from metabolism, airway obstruction, etc.) will not be abolished. Clinically, when opioids are used at high doses as part of a balanced anesthetic regimen, there is an additive effect of the opioid depression of the respiratory center and the general anesthetic, and considerable hypercarbia or even apnea may be produced. At the doses commonly employed for routine opioid premedication or postoperative analgesia in veterinary practice, severe respiratory depression is very rarely seen; but mild/moderate depression is common.
Tranquilizers
The phenothiazine and benzodiazepine sedatives often reduce the respiratory rate, especially if an animal is somewhat excited prior to administration, but they do not appreciably alter arterial blood gas tensions.
Sedatives and hypnotics
The alpha2 adrenoceptor agonists produce a more complicated effect on respiration. When used alone at sedative doses, the alpha2 agonists exhibit little evidence of true respiratory depression in healthy dogs or cats. There may be a decrease in respiratory rate and perhaps a small increase in PaCO2 levels, but PaO2 levels are well maintained. The peripheral cyanosis that has been reported in up to one-third of dogs sedated with medetomidine is believed to be caused by the low blood flow through mucous membrane capillary beds and venous desaturation, rather than a fall in arterial Hb saturation.
It is important to appreciate, however, that the degree of respiratory depression produced by any alpha2 agonist will be increased when the agonist is given along with other sedatives or anesthetic agents. A number of studies have clearly demonstrated that medetomidine produces elevated PaCO2 levels and PaO2 levels in the mildly hypoxic range (i.e., 60–70 mm Hg) when combined with opioids, propofol, or ketamine at clinical doses in healthy animals.
Ventilation-perfusion relationships during anesthesia
The onset of general anesthesia or a change in body position often produces lower PaO2 levels than expected for the delivered concentration of inspired oxygen. This change can occur even without hypoventilation and during both spontaneous and controlled breathing. Lower PaO2 is produced by altered ventilation/perfusion ratios within the lung. Much of what we know about this phenomenon of altered gas exchange is derived from studies of the human response to anesthesia, some experiments in dogs, and many studies on anesthetized horses. It is obvious when one looks at the collective results that there are important species differences, although the reason(s) for these differences are not always obvious.
Measurement of V/Q mismatch
When the barometric pressure, inspired oxygen concentration, PaCO.. and respiratory quotient are known, the PAO2 can be calculated by using one form of the alveolar air equation. The difference between this value and the PaO. (i.e., the alveolar-to-arterial gradient [P(A – a)O2]) provides a convenient and practical measure of the relative efficiency of gas exchange. The measured P(A – a)O2 value increases as FiO2 goes up for any given V/Q situation, and it is imperative that the FiO. level be taken into account when comparisons are made. In practice, most P(A – a)O2 determinations are made at oxygen concentrations of 21% or near 100%.
The amount of venous admixture or pulmonary shunt flow can be determined if mixed venous (pulmonary artery) and arterial blood oxygen contents are obtained along with a measurement of CO and calculated PaO2. The terms venous admixture and shunt flow do not mean exactly the same thing, although they are often used interchangeably in the literature, which causes some confusion. Venous admixture refers to the degree of admixture of mixed venous blood with pulmonary end-capillary blood that would be required to produce the observed difference between the arterial and the end-capillary PO2. The end-capillary PO2 is assumed to equal the alveolar PO2. Venous admixture is a calculated amount (i.e., a proportion of CO) and includes the PaO2 lowering effect of low V/Q areas, blood flow past nonventilated areas, and true anatomical shunt flow (bronchial and thebesian venous blood flow). When the inspired oxygen level is high, blood passing low V/Q areas will be oxygenated, and the P(A – a)O2 gradient and determination of venous admixture is a measure of the total blood flow not contributing to gas exchange, hence the term pulmonary shunt flow. Note that this flow includes both anatomical shunt flow and flow past nonventilated or collapsed alveoli. If one knows the inspired oxygen concentration and the PaO2, and assumes that the arterial-venous oxygen difference is normal, an isoshunt diagram can be used to provide a convenient and reasonably accurate estimate of the magnitude of pulmonary shunt flow.
Clinical implications of altered respiration during anesthesia
In reasonably healthy dogs and cats, the P(A – a)O2 gradient and the degree of venous admixture are less than in humans. Perhaps this is owing to the smaller lungs in these species or to the difference in the chest wall changes during anesthesia, or perhaps because there is excellent collateral pulmonary ventilation in these species. A high degree of collateral ventilation means that if an alveolus is not ventilated via the airway, it may well receive gas exchange through passages (pores of Kohn) leading to other alveoli that are ventilated. Despite the relatively favorable situation in regard to V/Q mismatch in these species, a minimum inspired oxygen level of 30–35% is still recommended. Obese, deeply anesthetized animals, animals with a distended abdomen (e.g., pregnancy or bowel obstruction), or those with pulmonary disease or space-occupying lesions of the thorax (tumor, pneumothorax, hemothorax, or diaphragmatic hernia) are particularly at risk. Oxygen supplementation is needed nearly as much in deeply sedated animals as in those receiving a general anesthetic (IV or inhalant). This is why simple maneuvers such as placing a face mask with oxygen on a high-risk patient before and during induction or use of a nasal oxygen catheter in the postoperative period are beneficial.
When 100% oxygen mixtures are used with the common inhalant anesthetics in dogs and cats free of serious cardiopulmonary disease, the arterial PaO2 level is generally 450–525 mm Hg whether the animal is breathing spontaneously or being ventilated and irrespective of body position. With such high inspired oxygen levels, hypoxemia usually occurs only through disconnection of the animal from the anesthetic machine, or with faulty placement of the endotracheal tube, cardiac arrest, or apnea for over 5 minutes. Nevertheless, even with such high PaO2 levels, tissue hypoxia can occur if Hb levels are low or circulation is inadequate (low CO). The decision to institute assisted or controlled intermittent positive pressure ventilation (IPPV) is generally made to prevent or treat hypercapnia, rather than to achieve oxygenation. Nearly all spontaneously breathing dogs and cats show some degree of hypoventilation and hypercapnia (PaCO2 of 45–55 mm Hg).
Here are a few guidelines relative to the respiratory component of anesthesia for dogs and cats:
(1) Nearly all canine anesthetics are better done with an endotracheal tube in place, and in many situations cats should be intubated.
(2) Use at least 30–35% inspired oxygen in all anesthetized dogs and cats, even those on an injectable anesthetic mixture, or when deeply sedated.
(3) Hypoxemia is rare in spontaneously breathing dogs and cats if they are breathing an oxygen mixture approaching 100%.
(4) After a prolonged period of anesthesia in cats and smaller dogs, and with shorter anesthetics in larger dogs with deep chests, it is advisable to inflate the lungs to 30 cm H2O of airway pressure (i.e., to “sigh” the lungs) periodically and at the end of anesthesia.
(5) Prolonged immobility and excessive fluid administration can lead to increased venous admixture and a fall in PaO2, in addition to that produced by anesthesia per se.
Nervous system anatomy and physiology
Generally, the nervous system can be separated into central and peripheral divisions, although they are integrated and function together. The central division, composed of the brain and spinal cord, contains all of the important nuclei and is essential for integrating sensory and motor functions. The peripheral division is composed of nerves (including cranial nerves) and ganglia that connect the organs and tissues to the central nervous system (CNS).
Brain anatomy
The brain is divisible into five regions based on embryological development: telencephalon, diencephalon, mesencephalon, metencephalon, and myelencephalon. Grossly, it can be divided into the cerebrum and the brain stem. The cerebrum consists of telencephalic and diencephalic portions. The telencephalic portion can be further divided into the cerebral hemispheres and basal ganglia (also known as the basal nuclei). Together, the right and left hemispheres comprise the cerebral cortex, which is organized into an outer cortical layer of gray matter that contains a high density of nerve cell bodies and an inner medulla of white matter consisting mainly of myelinated nerve fibers. The cerebral cortex is where sensory, motor, and associational activity occurs.
The diencephalic portion of the cerebrum, which is located below and between the cerebral hemispheres, is the central area through which most of the information traveling into and out of the hemispheres must traverse. It consists of the thalamus, subthalamus, epithalamus, and hypothalamus, and is positioned between the telencephalon and the brain stem. The brain stem is comprised of the portion of the brain caudal to the telencephalon, excluding the cerebellum (e.g., the midbrain, pons, and medulla oblongata). The midbrain contains sensory and motor pathways, the nuclei of the third and fourth cranial nerves (oculomotor and trochlear), and two major motor nuclei: the red nucleus and the substantia nigra.
Cranial nerves
These consist of a peripheral segment, a nuclear center in the brain stem (except olfactory and optic nerves), and communicating connections with other parts of the brain. All 12 cranial nerves are paired. Functionally, cranial nerves can be divided into motor (efferent), sensory (afferent), and mixed. Sensory and motor cranial nerves are associated with at least one nuclei, and mixed cranial nerves are associated with at least two nuclei) (Figure 2.8).
Brain physiology
The energy consuming processes of the brain are divided into those of neuroprocessing and maintenance of cellular integrity. Oxygen requirements for the conscious, healthy canine brain are approximately 5.5 mL/100 g/min, with 60% needed for neuroprocessing and 40% for maintenance of brain cell integrity. If cardiovascular function is normal, adequate oxygen delivery and normal electroencephalogram (EEG) function is expected with a PaO2 of approximately 100 mm Hg and cerebral blood flow (CBF) of 50mL/100g/min. Irreversible cerebral tissue damage is likely when PaO2 drops below 20–23 mm Hg and CBF below 10mL/100g/min. In humans, normal function of the awake brain requires approximately 3.5 mL O2/100 g/min, or a total of about 50 mL/min extracted from an average normal CBF of 57 mL/100 g/min. In the awake state, most of the brain’s cellular energy stores are depleted within 2–4 minutes after oxygen delivery is interrupted. During the anoxic period, cellular lactate concentrations can increase three-to fivefold. Anesthesia, hypothermia, or brain injury can alter cerebral metabolic requirements for oxygen (CMRO2) and must be considered when interpreting the adequacy of monitored cardiopulmonary parameters during anesthesia.
Figure 2.8. Ventral view of the canine brain and cranial nerves.
Source: Jenkins TJ. 1978. Functional Mammalian Neuroanatomy, 2nd ed. Philadelphia, PA: Lea and Febiger.

Effects of anesthetics on brain physiology
Anesthetic management of patients requires that CBF be maintained at a level sufficient to meet the brain’s metabolic demands. Autoregulation enables maintenance of a constant CBF over a wide range of systemic BPs. Autoregulation is not immediate; when systemic BP changes, it takes about 2 minutes for CBF to return to normal. There have been many studies on the effects of anesthetics on CBF and autoregulation, and the results have sometimes been confusing. All potent inhaled anesthetics tend to decrease cerebral metabolism and increase CBF and intracranial pressure (ICP), but vary in degree.
Spinal cord
Caudal to the medulla, the CNS continues as the spinal cord, which is contained in the spinal canal. The spinal cord is a complex collection of fibers organized into ascending and descending tracts, interneurons, neuron-supporting cells, blood vessels, and connective tissue. The cord is surrounded by the meninges, which support and protect it. From superficial to deep, they are the dura mater, arachnoid, and pia mater. In dogs, the cord and associated subarachnoid structures usually terminate at the level of L6–L7 and in cats, the cord terminates variably between L6 and the sacrum. Inadvertent subarachnoid administration of anesthetic or analgesic drugs is more likely in felines when the needle is inserted at the lumbosacral space.
The epidural space is not an empty cavitary space, but instead contains blood vessels, lymphatics, and epidural fat, and communicates with the paravertebral tissues via the intervertebral foramina. This communication may be interrupted in older animals by fibrous connective tissue and bony malformations associated with spinal arthritis, and in obese patients by fat. This is of clinical significance because epidural injection volumes are often reduced in older or obese animals to prevent excessive cranial spread of drugs.
The subarachnoid space is located between the arachnoidea and pia mater. The sub-arachnoid space contains CSF and is continuous between the cranial and vertebral segments. There is no direct communication between the epidural and subarachnoid spaces; however, drugs (especially lipophilic drugs) can diffuse across the arachnoidea and enter the CSF after epidural administration. The pia mater, which is one cell layer thick, lies directly on the brain and spinal cord. The pia probably does not present a significant barrier to drug diffusion. The meninges cover the dorsal and ventral spinal nerve roots until they fuse, at which point they merge with the spinal nerve and extend no farther peripherally.
CSF and ICP
Within the calvarium, CSF is found in both an internal (ventricular) system and an external (subarachnoid) system. The internal system consists of the bilaterally symmetrical lateral ventricles within the cerebral hemispheres, the third ventricle medially between the thalamus and hypothalamus, and the fourth ventricle lying beneath the cerebellum and within the medulla. CSF is produced by the choroid plexus, a fringelike fold of pia mater found on the floor of both lateral ventricles, in the fourth ventricle, and also by the ependymal lining of the ventricles. CSF is formed from the blood by secretory and filtration processes. Fluid in the lateral ventricles empties into the third ventricle through the paired foramina of Monro. The third ventricle, in turn, empties into the fourth through the aqueduct of Sylvius. The central canal of the spinal cord is continuous with the fourth ventricle. The external or subarachnoid system overlies the brain and spinal cord. The bilateral foramina of Luschka enable fluid to pass between the ventricular and subarachnoid systems. In most primates, the unpaired foramen of Magendie is present and enables an additional connection between the fourth ventricle and the subarachnoid space. The foramen of Magendie is not found in most common veterinary species (Figure 2.9).
Figure 2.9. Circulation of CSF in the human and subhuman primates. Nonprimate animals do not have the foramen of Magendie.
Source: Stoelting RK. 1991. Pharmacology and Physiology in Anesthetic Practice, 2nd ed. Philadelphia, PA: Lippincott.

CSF is absorbed at the arachnoid villi located primarily in the subdural venous sinuses. The arachnoid villi are fingerlike projections of the arachnoidal membrane that penetrate the venous sinuses. Their endothelium is porous and highly permeable, allowing free passage of water, electrolytes, proteins, and even red blood cells.
CSF cushions the brain and spinal cord. CSF normal pressure is approximately 10 mm Hg but can fluctuate within a narrow range. The composition of the CSF remains different from plasma because of the blood–brain barrier. The barrier is as much an enzymatic and cellular transporter barrier as an anatomical separation. The concentration of sodium is equal to that of plasma, the concentration of chloride in the CSF is 15% greater, and the concentration of potassium and glucose is 40% and 30% less than in plasma, respectively. The specific gravity of CSF is 1.002–1.009. The pH of CSF is closely maintained at 7.32.
Carbon dioxide, but not hydrogen ions, readily crosses the blood–brain barrier. Bicarbonate ions are actively transported. As a result, the pH of CSF is rapidly altered by changes in PaCO2 but not by changes in arterial pH. The pH of CSF, not the PCO2 directly, is the major mechanism regulating ventilation in most mammals.
The skull forms a noncompliant chamber filled with brain parenchyma, blood, and CSF. An increase in volume of one component must be accompanied by a compensatory decrease in the others or the ICP will increase (Monro-Kellie hypothesis). Normal ICP is less than 15 mm Hg. As the ICP increases, blood flow to the brain will decrease unless an increase in mean arterial pressure occurs to maintain cerebral perfusion pressure (cerebral perfusion pressure = mean arterial pressure – ICP). Arterial BP should always be measured in animals suspected of having intracranial hypertension, because anesthetic-induced decreases in mean arterial BP can lead to catastrophic cerebral ischemia even though arterial BPs were maintained above acceptable levels for healthy animals (e.g., mean arterial pressure of 60 mm Hg). Increased cerebral or spinal fluid pressure produces a reflex increase in HR and BP (Cushing’s response). It has been hypothesized that the increase in CSF pressure creates ischemia of neurons, and that this is the stimulus for increased sympathetic activity.
Drugs that reduce cerebral metabolic activity or CBF, osmotic diuretics, hypothermia, and mechanical normoventilation or hyperventilation may all be used to decrease ICP. It is imperative that anesthetic management focus on maintaining adequate cerebral perfusion pressure rather than on reducing CBF or ICP alone. Administration of analgesic drugs, especially opioids, must be carefully monitored in animals with intra-cranial disease, because of their potential to increase PaCO2, which could lead to increased CBF and ICP. Ventilatory support may be required if respiratory depression occurs.
Spinal nerves
These supply efferent and afferent innervation to most of the body, with the exception of the head and viscera. They also form part of the autonomic nervous system, which controls homeostatic functions. Spinal nerves vary in number, depending on species. Nerves can be classified by their size and degree of myelination, which determine speed of impulse transmission. Large, heavily myelinated fibers have the highest conduction velocities, whereas small nonmyelinated fibers have lower conduction rates. The largest fibers, type A, are subclassified as A-alpha, A-beta, A-gamma, and A-delta fibers. A-alpha fibers innervate skeletal muscles and also subserve proprioception. A-beta fibers normally subserve innocuous touch and pressure, but can be involved in nervous system “windup” that can result in hyperalgesia and/or allodynia following chronic nociceptor stimulation. A-gamma fibers innervate the skeletal muscle spindles to maintain muscle tone. A-delta fibers subserve temperature, fast pain, and touch. Type B fibers are preganglionic autonomic fibers. Type C fibers are small nonmyelinated fibers responsible for postganglionic sympathetic innervation and transmission of visceral and slow pain, touch, and temperature sensations. Abnormal nociceptive C fiber activity causes many chronic pain syndromes best described in humans, with diabetic neuropathy and postherpetic neuralgia being two examples. Similar C-fiber dysfunction is likely in a variety of chronic pain conditions in other mammalian species.
After leaving the intervertebral foramen, each spinal nerve divides into dorsal and ventral branches. The dorsal branches generally supply the muscles and skin of the back, whereas the ventral branches supply the muscles and skin of the thorax, abdomen, and extremities. Branches from several spinal nerves may combine to form plexuses such as the brachial plexus or major nerves such as the sciatic nerve.
Autonomic nervous system
In contrast with the somatic nervous system supplying the striated muscles, the autonomic nervous system requires no conscious control. The autonomic nervous system is composed of the efferent and afferent nerves innervating the viscera, glands, and other tissues required for homeostasis and the fight-or-flight response. Its primary homeostatic role is control of circulation, breathing, excretion, and maintenance of body temperature. These regulatory functions are subject to modification by input from higher brain centers, especially as a result of reactions to the environment.
Visceral autonomic efferent pathways consist of two neurons rather than a single motor neuron as occurs in the somatic system. The cell body of the first neuron is in the brain stem or spinal cord. Its axon terminates on the cell body of the second neuron, located in an autonomic ganglion. The axon of the ganglion cell terminates in the effector cell.
Autonomic visceral afferents are primarily sensory neurons similar to those in somatic tissues, although they tend to have wider receptive areas, leading to less ability to discriminate the anatomic origin of the afferent signals. They elicit reflex responses in viscera and a feeling of fullness of hollow organs, such as the stomach, large intestine, and bladder. Afferent impulses contribute to feelings of well-being or malaise and transmit signals from nociceptors. Visceral pain afferents are associated with the sympathetic division.
The hypothalamus is the primary area of the brain that controls the autonomic nervous system. The autonomic system can be subdivided into the craniosacral or parasympathetic system and the thoracolumbar or sympathetic system. A characteristic of the autonomic nervous system is that both divisions are constantly active, resulting in a basal level of sympathetic and parasympathetic activity. Thus, each division can increase or decrease its effect at a given organ to regulate function more closely.
Neuron function
Axonal conduction
Nerve impulses are electrochemical currents that pass along the axon to the presynaptic membrane. From a pharmacological standpoint, there is an important distinction between electric conduction of a nerve impulse along an axon and chemical transmission of this signal across the synapse. Most general anesthetics have little effect on nerve conduction velocity.
Neuroregulators
These play a key role in communication among nerve cells and may be subdivided into two groups. Small molecule neurotransmitters are synthesized in the cytosol of the pre-synaptic terminal, absorbed into the transmitter vesicles, and released into the synaptic cleft in response to the arrival of an action potential at the nerve ending. Release of neurotransmitters is voltage dependent and requires calcium influx into the presynaptic terminal. Following its release, the transmitter binds with the postsynaptic receptor. The postjunctional membrane is excited by increased sodium conductance and inhibited when potassium or chloride conductance is enhanced. Some transmitters bind to receptors that activate enzymes, thus altering cellular function.
Neuropeptide modulators are synthesized in the neuronal cell body and transported to the nerve terminal by axonal streaming. They are released in response to an action potential, but in much smaller quantities than are the small molecule transmitters. The neuropeptides induce prolonged effects to amplify or dampen neuronal activity. They exert their effects through a variety of mechanisms, including prolonged closure of calcium channels, alteration of cellular metabolism, activation or inactivation of specific genes, and prolonged alteration in the numbers of excitatory or inhibitory receptors. Although usually only a single small molecule neurotransmitter is released by each type of neuron (Dale’s law), the same neuron may release one or more neuropeptide modulators at the same time.
Sedative and anticholinergic pharmacology
Anticholinergic drugs
Anticholinergics are used perioperatively to manage bradycardia and AV block associated with surgical manipulation (oculovagal and viscerovagal reflexes) or with the administration of other anesthetic adjunctive drugs (e.g., alpha2 agonists or opioids). Occasionally, they are also used to control excessive oral and airway secretions. Anticholinergics should not be administered perioperatively on an indiscriminate basis. Rather, the risks and benefits associated with the administration of different drugs should be assessed, and the safest drugs chosen for each patient.
Anticholinergics are often called parasympatholytic drugs because they block the effects of the parasympathetic nervous system on other body systems, especially the cardiovascular and gastrointestinal systems. Atropine and glycopyrrolate are the anticho-linergics used most commonly in veterinary medicine. These two drugs do not block nicotinic cholinergic receptors and are more accurately classified as antimuscarinics. There are three major types of muscarinic receptors: M1, M2, and M3. M1 receptors are located on neurons in the CNS and on autonomic ganglia. M2 receptors are located in the SA and AV nodes and in the atrial myocardium. M3 receptors are located in secretory glands, vascular endothelium, and smooth muscle.
Atropine and glycopyrrolate are relatively nonselective muscarinic antagonists, but despite this lack of selectively the effectiveness of muscarinic blockade varies considerably from tissue to tissue. Salivary and bronchial glands are the most sensitive to muscarinic blockade. Cardiac tissues and smooth muscle are intermediate in sensitivity, and gastric parietal cells are the least sensitive to muscarinic blockade.
Anticholinergic administration routinely causes sinus tachycardia, which is problematic for many patients with cardiovascular disease. Tachycardia associated with administration of anticholinergics leads to an increase in myocardial work and a decrease in myocardial perfusion. Further, coadministration of anticholinergics and ketamine has been associated with the development of myocardial infarcts in, and the death of, young cats undergoing routine surgical procedures. At lower doses, a transient decrease in sinus rate and slowing of AV nodal conduction (AV blockade) can occur. This response appears to be due to blockade of presynaptic muscarinic receptors that normally inhibit acetylcholine (ACh) release. Once postsynaptic muscarinic blockade is established, this paradoxical increase in vagal tone usually resolves.
Anticholinergic administration also has dramatic effects on gastrointestinal function. At therapeutic doses, nonselective muscarinic antagonists like atropine and glycopyr-rolate reduce lower esophageal sphincter tone and have little effect on gastric pH. These two factors increase the incidence of gastroesophageal reflux and esophagitis in anesthetized dogs. Perioperative administration of anticholinergics also reduces intestinal motility and can lead to gastrointestinal complications postoperatively.
Atropine
Chemically, atropine consists of two components (tropic acid and an organic base) that are bound by an ester linkage. Atropine has approximately the same affinity for all three major types of muscarinic receptors. Relative to other synthetic muscarinic antagonists, atropine is very selective for muscarinic receptors and has little effect on nicotinic receptors.
Pharmacokinetics and pharmacodynamics
Atropine is rapidly absorbed after intramuscular (IM) administration. Onset of cardiovascular effects occurs within 5 minutes, and peak effects occur within 10–20 minutes. After IV administration at a dose of 0.03 mg/kg, onset of cardiovascular effects occurs within 1 minute, peak effects occur within 5 minutes, and HR increases by 30–40% for approximately 30 minutes. The effects of atropine on other body systems subside within a few hours, but ocular effects can persist for 1–2 days. Atropine is rapidly eliminated from the blood after parenteral administration. Some of the drug is hydrolyzed to inactive metabolites (tropine and tropic acid), and some of it is excreted unchanged in the urine. Rabbits and some other species (cats and rats) have a plasma enzyme (atropine esterase) that accelerates metabolism and clearance of the drug.
At therapeutic doses, atropine administration produces limited effects on the CNS. A mild sedative effect may be observed, and the incidence of vomiting mediated by the vestibular system may be reduced. Blockade of the pupillary constrictor muscle and the ciliary muscle produces long-lasting mydriasis and cycloplegia, respectively. Lacrimal secretions are also reduced, which may contribute to corneal drying during anesthesia unless artificial tears are applied concurrently. Atropine should be used with discretion in animals with acute glaucoma because its mydriatic effect may impede drainage from the anterior chamber.
Airway smooth muscle and secretory glands also receive parasympathetic input from the vagus nerves. Blockade of M3 receptors by therapeutic doses of atropine decreases airway secretions and increases airway diameter and anatomical dead space. In the past, atropine was given before administration of noxious inhaled anesthetics (ether) to reduce airway secretions and the potential for laryngospasm. Modern inhaled anesthetics do not cause the same degree of airway irritation, and routine preoperative administration of atropine for this reason is difficult to justify.
Clinical uses
Atropine can be given subcutaneously (SC), IM, or IV, but the IM and IV routes are preferred because uptake from subcutaneous sites can be erratic in patients with altered hydration and peripheral circulation. Doses for dogs and cats range from 0.02 to 0.04 mg/kg. Atropine is also effective when given endotracheally or endobronchially to dogs for cardiopulmonary resuscitation.
Glycopyrrolate
Glycopyrrolate is a synthetic quaternary ammonium muscarinic antagonist. Like atropine, the drug consists of two components (mandelic acid and an organic base) bound together by an ester linkage. Glycopyrrolate is four times as potent as atropine and has approximately the same affinity for all three major types of muscarinic receptors. The drug’s polar structure (quaternary amine) limits diffusion across lipid membranes and into the CNS and fetal circulation.
Pharmacokinetics and pharmacodynamics
Absorption, metabolism, and elimination of glycopyrrolate are similar to that of atropine. Absorption is rapid after IM administration. Onset of cardiovascular effects occurs within 5 minutes, peak effects occur within 20 minutes, and HR remains elevated for approximately 1 hour. Glycopyrrolate is rapidly eliminated from the blood after parenteral administration, and most of the drug is excreted unchanged in the urine.
At therapeutic doses, glycopyrrolate produces few, if any, effects on the CNS. Unlike atropine administration, sedation is not observed, and recovery times are not prolonged. Administration of glycopyrrolate to conscious dogs with normal intraocular pressure does not alter pupil diameter and intraocular pressure, and intraoperative administration of glycopyrrolate to dogs with glaucoma and increased intraocular pressure appears to be safe.
Glycopyrrolate administration produces effects on the heart that are comparable to those of atropine. Studies in people suggest that glycopyrrolate produces less tachycardia than atropine, but the two drugs produce similar increases in HR when administered IV to sedated or anesthetized dogs. The typical response to IV or IM administration of therapeutic doses (5-10μg/kg) of glycopyrrolate is an increase in sinus rate, acceleration of AV nodal conduction, and an increase in atrial contractility. At lower doses, a transient decrease in sinus rate and slowing of AV nodal conduction can occur. Glycopyrrolate can also be given intraoperatively to correct bradycardia.
Like atropine, glycopyrrolate affects the gastrointestinal system. Intestinal motility is reduced for at least 30 minutes in anesthetized dogs.
Clinical uses
Glycopyrrolate is used perioperatively to prevent severe bradycardia caused by surgical manipulation (vagal reflexes) or by administration of other anesthetic drugs. Doses for dogs and cats range from 5 to 10 μg/kg.
Sedatives
Behavioral responses to different classes of sedatives vary considerably among species. The phenothiazines and alpha2 agonists are effective sedatives in dogs and cats. Conversely, the benzodiazepines are effective sedatives in ferrets, rabbits, and birds but are not reliable sedatives in cats and young dogs.
Phenothiazines and butyrophenones
Phenothiazines and, to a lesser extent, butyrophenones produce a wide variety of behavioral, autonomic, and endocrine effects. The behavioral effects of these drugs are mediated primarily by blockade of dopamine receptors in the basal ganglia and limbic system. At therapeutic doses, phenothiazines and butyrophenones inhibit conditioned avoidance behavior and decrease spontaneous motor activity. At higher doses, extrapyramidal effects (tremor, rigidity, and catalepsy) can occur. These sedatives also have significant binding affinity for adrenergic and muscarinic receptors. For example, phenothiazines bind with great affinity to, and act as antagonists at, alpha1 adrenergic receptors, which may result in hypotension that is typically associated with perioperative use of these drugs. Blockade of dopamine receptors in the chemoreceptor trigger zone of the medulla produces an antiemetic effect, and depletion of catecholamines in the thermoregulatory center of the hypothalamus leads to a loss of thermoregulatory control.
Acepromazine (a phenothiazine) is one of the most widely used sedatives in veterinary medicine. The chemical name of acepromazine is 2-acetyl-10-(3-dimethylaminopropyl) phenothiazine. Acepromazine is often given in combination with an opioid as a preanesthetic to facilitate the placement of IV catheters and to reduce the dose of injectable and inhalational anesthetics required to induce and maintain anesthesia. Acepromazine can also be given postoperatively to smooth recovery, provided that patients are hemodynamically stable and that pain has been managed effectively. IM doses for cats and small dogs range from 0.01 to 0.2 mg/kg, and those for larger dogs range from 0.01 to 0.05 mg/kg. Generally, doses less than 0.05 mg/kg are adequate for mild to moderate sedation in the pre- or postanesthetic period.
Alpha2 adrenergic agonists
In most species, alpha2 agonists produce reliable dose-dependent sedation, analgesia, and muscle relaxation that can be readily reversed by administration of selective antagonists. Xylazine has been used in both small and large animals for over three decades. In small animals, medetomidine, and more recently its purified isomer dexmedetomidine, have been used.
The alpha2 receptors are located in tissues throughout the body, and norepinephrine is the endogenous ligand for these receptors. The alpha2 receptors exist presynaptically and postsynaptically in neuronal and nonneuronal tissues, and extrasynaptically in the vascular endothelium and in platelets. Within the nervous system, alphai receptors are located presynaptically on noradrenergic neurons (autoreceptors) and on nonnoradrenergic neurons (heteroceptors). The sedative and anxiolytic effects of alphai agonists are mediated by activation of supraspinal autoreceptors or postsynaptic receptors located in the pons (locus ceruleus), and some of the analgesic effects are mediated by activation of heteroceptors located in the dorsal horn of the spinal cord. Supraspinal alpha2 receptors located in the pons also play a prominent role in descending modulation of nociceptive input.
Three distinct alpha2 receptor subtypes (A, B, and C) have been identified. The cellular response to activation of these receptor subtypes is mediated by several different molecular mechanisms.
Medetomidine and dexmedetomidine
Medetomidine, (±)-4-(1-[2,3-dimethylphenyl] ethyl)-1H-imidazole monohydrochloride is a racemic mixture of levo- and dextrorotory enantiomers. All or nearly all of the pharmacological action is due to the dextrorotory enantiomer, dexmedetomidine. Shortly after medetomidine was approved for use in dogs as a sedative–analgesic in North America, dexmedetomidine was approved for use in people as a postoperative sedative in the United States. As expected, dexmedetomidine is approximately twice as potent as the racemic mixture that is available for use in animals. In dogs and cats, both dexmedetomidine and medetomidine have a rapid onset of action and can be administered IV or IM. After IM administration, the drug is rapidly absorbed, and peak plasma concentrations are reached within 30 minutes. Elimination occurs mainly by biotransformation in the liver, and inactive metabolites are excreted in the urine.
Onset of sedation, analgesia, and muscle relaxation is rapid after IM administration of medetomidine to dogs and cats, and the intensity and duration of these effects depend on dose. When medetomidine is given IM to dogs at a dose of 30 μg/kg, significant sedation is apparent within 5 minutes and persists for 1–2 hours. Similarly, when medetomidine is given to cats at a dose of 50 μg/kg, significant sedation is apparent within 15 minutes and persists for 1–2 hours. At these doses, analgesia peaks within 30 minutes and persists for 1–2 hours.
Medetomidine administration decreases injectable and inhalational anesthetic requirements dramatically (by over 50% in dogs). Administration of medetomidine IM at doses of 10, 20, and 40 μg/kg decreases the amount of thiopental required for intubation to 7.0, 4.5, and 2.4mg/kg, respectively. Similarly, administration of medetomidine IM at a dose of 20 μg/kg decreases the amount of propofol required for intubation to 1.8 mg/kg. Administration of medetomidine IM also reduces the dose of ketamine required to induce anesthesia in dogs and cats. Administration of medetomidine IV at a dose of 30 μg/kg decreased the MAC of isoflurane by 47%. Additionally, administration of medetomidine IM at a dose of 8 μg/kg consistently reduced the bispectral index value (an index of anesthetic depth) in dogs anesthetized with isoflurane (1.0, 1.5, and 2.0 MAC).
As with other alpha2 agonists, medetomidine administration produces changes in commonly monitored cardiovascular parameters. Cardiovascular effects are best described in two phases: an initial peripheral phase characterized by vasoconstriction, increased BP, and reflex vagal bradycardia; and a subsequent central phase characterized by decreased sympathetic tone, sympathetically driven HR, and BP. Occasionally, AV blockade occurs secondary to the initial increase in BP and reflex (baroreceptor-mediated) increase in vagal tone. In conscious dogs, mean arterial pressure increases transiently, and HR and cardiac index decrease by approximately 60% after IV administration of medetomidine at doses ranging from 5 to 20 μg/kg. At these doses, changes in mean arterial pressure, central venous pressure, and vascular resistance are dose dependent, whereas changes in HR and cardiac index are not. In conscious cats, mean arterial pressure does not appear to change (perhaps related to higher baseline stress levels) and HR and cardiac index decrease by approximately 50% after IM administration of medetomidine at a dose of 20 μg/kg. In cats anesthetized with isoflurane (2%), mean arterial pressure increases from 77 to 122 mm Hg, HR decreases from 150 to 125 beats per minute, and mean arterial flow decreases from 578 to 325mL/min, 20 minutes after the IM administration of medetomidine at a dose of 10 μg/kg.
In conscious animals, the decrease in CO is caused primarily by the decrease in HR and increase in vascular resistance, and not by a direct depression of myocardial contractility. Although CO decreases after medetomidine or dexmedetomidine administration, blood flow to the heart, brain, and kidneys is maintained by redistribution of flow from less vital organs and tissues. In patients with good cardiopulmonary reserve, the concurrent administration of an anticholinergic agent will prevent bradyarrhythmias while slightly improving CO at the expense of a rather large increase in myocardial work and O2 consumption. Thus, the use of an anticholinergic preoperatively with alpha2 agonists to prevent bradycardia and AV blockade continues to be somewhat controversial. The use of an anticholinergic has been recommended for the following reasons: First, even at low preanesthetic doses, significant bradycardia can occur if an anticholinergic is not administered concurrently. Second, the potential for severe vagotonic responses and profound bradycardia, secondary to surgical manipulation and administration of other anesthetic drugs (opioids), is higher during the perioperative period. Third, while the concurrent administration of anticholinergics with high doses of medetomidine can cause dramatic increases in vascular resistance and myocardial work, these increases can be minimized and are generally well tolerated by healthy patients given low doses of medetomidine prior to inhalant (vasodilatory) anesthesia.
Medetomidine administration has little effect on pulmonary function. Respiratory rate and VE decrease after medetomidine administration, but this decrease in VE appears to parallel a decrease in metabolic CO2 production, and arterial blood gas values remain stable.
Medetomidine administration has significant effects on gastrointestinal function in animals. Vomiting occurs in 10% of dogs and over 50% of cats administered medetomidine IM at mean doses of 40 and 80 μg/kg, respectively. Vomiting dramatically increases intraocular pressure, which is a potential problem for some patients with ocular injury or disease. Medetomidine administration decreases gastrin release and intestinal and colonic motility in dogs. These effects are mediated by activation of visceral alpha2 receptors and inhibition of ACh release.
Medetomidine administration has significant effects on renal and urogenital function in animals. In dogs, administration of medetomidine (10–20 μg/kg IV) decreases urine specific gravity and increases urine production for approximately 4 hours. Apparently, alpha2 agonists interfere with the action of antidiuretic hormone on the renal tubules and collecting ducts, which increases the production of dilute urine.
Preoperative administration of alpha2 agonists attenuates the stress response associated with surgical trauma. In dogs undergoing ovariohysterectomy, preoperative administration of medetomidine reduces catecholamine and cortisol concentrations postoperatively. Similarly, preoperative administration of medetomidine (20 μg/kg IM) attenuates perioperative increases in norepinephrine, epinephrine, and cortisol concentrations to a greater degree than does acepromazine. Administration of xylazine or medetomidine activates alpha2 receptors on pancreatic beta cells and inhibits release of insulin for approximately 2 hours, resulting in an increase in plasma glucose concentrations.
As a general rule, medetomidine should not be administered to pediatric or geriatric animals, or to animals with significant neurological, cardiovascular, respiratory, hepatic, or renal disease. Once preanesthetic and anesthetic drugs are administered, patients should be monitored carefully throughout the perioperative period, with special attention being paid to HR and rhythm.
Xylazine
Although its mechanism of action was unknown at the time of its introduction into clinical practice, xylazine was the first alpha2 agonist to be used by veterinarians. The drug was synthesized in West Germany in 1962 for use as an antihypertensive in people but was found to have potent sedative effects in animals. The chemical name for xylazine is 2(2,6-dimethylphenylamino)-4H-5,6-dihydro-1,3-thiazine hydrochloride. Initially, the drug was used as a sedative in cattle and other ruminants in Europe. In the early 1970s, reports of xylazine’s utility as an anesthetic adjunct began appearing in American and European veterinary literature. These reports documented the effectiveness of xylazine in eliminating muscular hypertonicity in dogs and cats given ketamine, and in producing rapid, predictable sedation, analgesia, and muscle relaxation in horses and cattle after IV administration. It was also evident that there was tremendous variation in the dose of xylazine required to produce equivalent levels of sedation and analgesia in different species. In 1981, the sedative and analgesic effects of xylazine were definitively linked to the activation of central alpha2 adrenergic receptors.
Most clinical studies show that the sedative and analgesic effects of xylazine are comparable in duration and do not support the “conventional wisdom” that the analgesic effect is significantly shorter than the sedative effect. Xylazine administration dramatically decreases injectable and inhalational anesthetic requirements in several species (similar to dexmedetomidine).
Alpha2 adrenergic antagonists
Alpha2 agonists are used to reverse the sedative and cardiovascular effects of alpha2 agonists. Currently, three antagonists (tolazoline, yohimbine, and atipamezole) are available for use in animals, with only atipamezole recommended for reversal of medetomidine and dexmedetomidine in small animals.
In addition to reversing the sedative and cardiovascular effects of alpha2 agonists, alpha2 antagonists can produce significant side effects. If a relative overdose of an antagonist is administered, neurological (excitement and muscle tremors), cardiovascular (hypotension and tachycardia), and gastrointestinal (salivation and diarrhea) side effects can occur. Death has also been reported after rapid IV administration. The mechanism is likely due to the rapid reversal of vasoconstriction without sufficient time for the sympathetic nervous system to increase CO, resulting in severe hypotension.
Complete reversal of the sedative, analgesic, and cardiovascular effects of medetomidine is achieved when atipamezole is administered IM to dogs and cats at four to six times and two to four times the dose (based on micrograms given and not on volume) of medetomidine, respectively. Therefore, if the initial dose of medetomidine is 20 μg/kg for a dog, then atipamezole would be given IM at a dose of 100 μg/kg. Similarly, if the initial dose of medetomidine is 40 μg/kg for a cat, then atipamezole would be given IM at a dose of 120 μg/kg. In both of these examples, the dose of atipamezole may be reduced if more than 30 minutes has elapsed since medetomidine administration.
Benzodiazepine sedatives
Benzodiazepines produce most of their pharmacological effects by modulating gamma-aminobutyric acid (GABA)-mediated neurotransmission. GABA is the primary inhibitory neurotransmitter in the mammalian nervous system and cell membranes of most CNS neurons express GABA receptors. These receptors are also found outside the CNS in autonomic ganglia. Two main types of GABA receptors are involved in neuronal transmission: The GABAa receptor complex is a ligandigated chloride channel that consists of a central pore surrounded by five glycoprotein subunits.
The benzodiazepineibinding site, as well as the binding sites for other injectable anesthetics (barbiturates, propofol, and etomidate), is located in the GABAA receptor complex. Benzodiazepines enhance binding between GABA and the GABAA receptor, and increase the frequency of channel opening. In contrast, barbiturates enhance intrinsic activity and increase the duration of channel opening. Both mechanisms increase chloride conductance and hyperpolarize the cell membrane, which reduces neuronal excitability. Benzodiazepines have no intrinsic agonist activity and cannot alter chloride conductance in the absence of GABA. This lack of intrinsic activity limits CNS depression and provides benzodiazepines with a much wider margin of safety than barbiturates.
Ligands that bind to benzodiazepine receptors are classified as agonists, inverse agonists, and antagonists. Agonists bind to benzodiazepine receptors and produce sedative, anxiolytic, muscle relaxant, and anticonvulsant effects in most animals. Inverse agonists bind to the same receptor and produce the opposite effects. Antagonists have high affinity for the benzodiazepine receptor and have little or no intrinsic activity. These ligands block or reverse the effects of both agonists and inverse agonists. Diazepam, midazolam, and zolazepam are the benzodiazepine agonists used most commonly in veterinary medicine. Diazepam and midazolam are used primarily as sedatives, muscle relaxants, and anticonvulsants. Zolazepam is available in combination with a dissociative anesthetic (tiletamine), which is approved for use as an anesthetic in dogs and cats in the United States.
Diazepam
The chemical name for the diazepam is 7-chloro-1,3-dihydro-1-methyl-5-phenyl-2H-1,4-benzodiazepin-2-one. Diazepam is not soluble in water, and parenteral formulations contain 40% propylene glycol and 10% ethanol. The drug is also sensitive to light and adheres to plastic, so it should not be stored in plastic syringes for extended periods. Diazepam is used primarily as a muscle relaxant and as an anticonvulsant for dogs and cats.
Diazepam is highly lipid soluble and is rapidly distributed throughout the body. Approximately 90% of the drug is protein bound, and diazepam is metabolized by demethylation and hydroxylation to N-desmethyldiazepam (nordiazepam), 3-hydroxydiazepam, and oxazepam. Nordiazepam and oxazepam produce significant pharmacological effects at clinically relevant concentrations. In dogs, the elimination half-life of diazepam after administration of a relatively high dose (2 mg/kg IV) is 3.2 hours. Nordiazepam appears rapidly in plasma and quickly exceeds concentrations of diazepam, whereas oxazepam concentrations peak within 2 hours. The elimination half-Iives of nordiazepam and oxazepam are 3.6 and 5.7 hours, respectively. In cats, the mean elimination half-Iife of diazepam after administration of relatively high doses (5, 10, and 20mg/kg IV) is 5.5 hours. Approximately 50% of the diazepam dose is converted to nordiazepam, and the mean elimination half-life of the metabolite is 21 hours, which is approximately four times longer than the half-life of diazepam.
Diazepam does not sedate dogs reliably, and can cause excitement, dysphoria, and ataxia. In dogs, IV administration of diazepam (0.5 mg/kg) produces arousal and excitement. Diazepam administration can produce dysphoria and aggressive behavior in cats, and the drug should be used with this potential response in mind in this species. Because of these behavioral effects, diazepam alone has limited value as a sedative for dogs and cats.
Diazepam is not a reliable sedative, but is a good muscle relaxant and anticonvulsant in most species. In dogs, diazepam is commonly administered IV at a dose of 0.2–0.5 mg/kg immediately before induction of anesthesia with ketamine. Diazepam also can be administered prior to induction of anesthesia with thiopental, propofol, etomidate, or an opioid. Diazepam appears to be a more reliable sedative in older dogs and can be administered alone or in combination with an opioid to produce sedation in this subpopulation. Higher doses are often administered when diazepam is used as an anticonvulsant. In small animals, diazepam is administered IV at a dose of 0.5–1.0mg/kg to control seizures. The parenteral formulation of diazepam is very irritating and potentially cardiotoxic, and should be administered by slow IV injection.
Midazolam
Midazolam is a benzodiazepine with a fused imidazole ring that accounts for the water solubility of the drug at pH values below 4.0. The chemical name of midazolam is 8-chloro-6-(2-fluorophenyl)-1-methyl-4H-imidazo(1,5-a)(1,4)-benzodiazepine. The pH of the parenteral formulation is 3.5, and the drug is light sensitive like diazepam. At blood pH, midazolam changes its chemical configuration and becomes more lipid soluble, facilitating diffusion into tissues. Midazolam is almost completely (>90%) absorbed after IM injection, and peak plasma concentrations are reached within 15 minutes. The drug is also highly protein bound (>95%) and rapidly crosses the blood-brain barrier. Midazolam is hydroxylated in the liver, and glucuronide conjugates are excreted in the urine.
Midazolam is commonly given to enhance muscle relaxation and facilitate intubation in dogs and cats, and is coadministered with ketamine, etomidate, or propofol. Preanesthetic administration (0.1–0.2mg/kg IV) reduces the induction dose of barbiturates and propofol and the concentration of isoflurane required to maintain anesthesia during surgery. Midazolam administration produces minimal effects on cardiopulmonary function in mammals and birds. Because midazolam has limited effects on cardiopulmonary function, the drug is an ideal sedative for many older or compromised animals. In dogs, midazolam is typically administered alone at doses of 0.2–0.4 mg/kg IM or in combination with opioids (butorphanol, hydromorphone, or oxymorphone) to induce sedative effects. It can be administered IV at doses of 0.1–0.2 mg/kg before induction of anesthesia with ketamine, thiopental, propofol, or etomidate.
Benzodiazepine antagonists
Antagonists have a strong affinity for the benzodiazepine receptor but have no intrinsic activity and are relatively free of side effects. Additionally, benzodiazepine antagonists cannot reverse the effects of anesthetic drugs (barbiturates) that bind to other sites on the GABAA receptor complex. Flumazenil is the only benzodiazepine antagonist currently available for clinical use. In animals, it is used primarily to reverse the sedative and muscle relaxant effects of diazepam and other benzodiazepines.
Flumazenil
Flumazenil is a highly selective, competitive benzodiazepine receptor antagonist. The chemical name of the drug is ethyl-8-fluro-5,6-dihydro-5-methyl-6-oxo-4H-imidazolo-(1,5-a)benzodiazepine-3-carboxylate. Limited pharmacokinetic data are available for animals. An elimination half-life of 0.4–1.3 hours has been reported for dogs. In people, midazolam and flumazenil have similar pharmacokinetic profiles, which makes flumazenil a suitable antagonist for midazolam. Flumazenil rapidly reverses the sedative and muscle relaxant effects of benzodiazepine agonists in animals. In dogs, flumazenil administration completely reverses the behavioral and muscle relaxant effects of an overdose of diazepam (2 mg/kg IV) or midazolam (1 mg/kg IV) within 5 minutes. In addition, flumazenil may reverse the anticonvulsant effects of benzodiazepine agonists. Although flumazenil has minimal intrinsic activity, administration of the antagonist could facilitate the development of seizures in predisposed animals. Flumazenil also appears to have minimal effects on cardiopulmonary function in animals. Currently, flumazenil is the only benzodiazepine antagonist used in veterinary medicine. In dogs, an overdose of diazepam (2.0 mg/kg IV) or midazolam (1.0 mg/kg IV) can be effectively antagonized with flumazenil at a dose of 0.08 mg/kg. These doses correspond to agonist/antagonist ratios of 26:1 and 13:1 for diazepam/flumazenil and midazolam/flumazenil, respectively.
Injectable anesthetic drugs are used to induce an unconscious or hypnotic state or are administered by repeated injection and continuous infusion to maintain the mental depression necessary for anesthesia. The search for new drugs and combinations with appropriate pharmacokinetic–pharmacodynamic profiles for use in domestic and wild animals is ongoing. In animals, unlike in people, a state approaching general anesthesia is not achievable with the use of opioids alone. Consequently, in veterinary anesthesia, opioids have been primarily used as analgesics perioperatively and as anesthetic adjuncts to induce a state of neuroleptanesthesia and are not employed alone as IV anesthetics.
Barbiturate drugs
The barbiturates have been classified into four groups according to duration of action: long, intermediate, short, and ultrashort. All of those used for clinical anesthesia fall in the short or ultrashort classification, whereas those used for sedation or control of convulsions are of long or intermediate action.
The principal effect of a barbiturate is depression of the CNS by interference with passage of impulses to the cerebral cortex. Barbiturates act directly on CNS neurons in a manner similar to that of the inhibitory transmitter GABA. At clinical drug concentrations, barbiturates have two mechanisms of action at GABAA receptors. At lower concentrations, barbiturates exert a GABA-mimetic effect by decreasing the rate of dissociation of GABA from the GABAA receptor. At increasing drug concentrations, barbiturates directly activate the chloride ion channel associated with the GABAA receptor. The GABA-mimetic effects of barbiturates are thought to produce their sedative hypnotic effects, whereas the direct chloride ion channel activation produces their anesthetic effects.
Barbiturates diffuse throughout the body, penetrating cell walls and crossing the placenta. The extent of ionization, lipid solubility (partition coefficient [PC]), and protein binding are the three most important factors in distribution and elimination of barbiturates.
Phenobarbital sodium
Phenobarbital is a long-acting barbiturate, and advantage has been taken of its prolonged action in treating various convulsive disorders. Phenobarbital is not used as an anesthetic agent. However, coadministration of some anesthetics metabolized by the same microsomal enzyme may result in shorter half-life and duration of action due to microsomal enzyme induction.
Pentobarbital sodium
The duration of surgical anesthesia with anesthetizing doses (ca. 30 mg/kg) of pentobarbital varies widely with individual animals, averaging about 30 minutes. Complete recovery usually occurs in 6–18 hours. Occasionally, animals, particularly cats, may not rouse for as long as 24–72 hours. Because of the longer recoveries and availability of more predictable shorter acting agents, pentobarbital is no longer used in North America to produce anesthesia in most small companion animals. It is, however, the main component of most injectable euthanasia solutions.
Methohexital sodium
This is an ultrashort-acting barbiturate that is unique in that it contains no sulfur atom. Its short duration owes more to redistribution than to rapid metabolism. The dose for dogs or cats is 6–10 mg/kg of body weight. Half of the estimated dose is injected IV at a rapid rate, followed by administration to effect. Surgical anesthesia for 5–15 minutes is obtained by an initial injection. More prolonged anesthesia can be maintained by intermittent administration or continuous drip. Recovery is quick and may be accompanied by muscular tremors and violent excitement, which detract from the usefulness of the drug. Even with preanesthetic sedation, the recovery period is characterized by muscle tremors and struggling. Dogs are usually ambulatory 30 minutes after administration ceases.
Thiopental sodium
Thiopental is the thio-analog of pentobarbital sodium, and differs only in that the number 2 carbon has a sulfur atom instead of an oxygen atom attached to it. Thiopental has an ultrashort action because it is rapidly redistributed (e.g., into muscle tissue) and becomes localized in body fat. As concentrations in the plasma, muscle, and viscera fall, the thiopental concentration in fat continues to rise. On the other hand, an appreciable amount is metabolized by the liver, and this contributes to the early rapid reduction of arterial thiopental concentration.
For rapid induction of anesthesia of short duration, the dose is 10–12mg/kg. Should 10–20 minutes of surgical anesthesia be required, the dose range is 20–30mg/kg. One-third of the estimated dose is injected rapidly within 15 seconds, and the remainder is administered slowly to effect. Additional doses may be administered to prolong anesthesia when required. Following large-dose administration, recovery (to standing) usually requires 1–1.5 hours. Large doses will saturate the tissues and cause a prolonged emergence. When induction is preceded by preanesthetic sedation, a dose range of 8–15 mg/kg is used (Figure 2.10).
Occasionally, animals may struggle during induction of barbiturate anesthesia, and some of the drug may be administered perivascularly. This should be avoided if at all possible because a tissue slough may develop. If it is suspected that barbiturate solution has been injected perivascularly, the area should be infiltrated with 1 or 2mL of 2% lidocaine solution. Local anesthetics are effective for two reasons. First, they are vasodilators and prevent vasospasm in the area, and thus aid in dilution and absorption of the barbiturate. Second, they are broken down in an alkaline medium, and this reaction neutralizes the alkali (barbiturate). The use of hot packs or hydrotherapy may be beneficial, as is infiltration of the area with saline to dilute the barbiturate further. Additionally, systemic anti-inflammatory drugs may be of benefit.
Neurosteroids
This class of drugs was first evaluated as a combination of two steroids: alphaxalone and alphadolone acetate. The combination of the two steroids has an exceptionally high therapeutic index (30.6). It has little cumulative effect and the duration of anesthesia varies with species. A new neurosteroid product has been developed that is a 10 mg/mL solution of alphaxalone in 2-hydroxypropyl-ß-cyclodextrin (Alfaxan-CD; Jurox, Rutherford, Australia). This preparation does not appear to cause histamine release, which has been associated with the vehicle used in earlier neurosteroid preparations.
Figure 2.10. The average duration of anesthesia after successive hourly IV injections of equal doses of thiopental to dogs.
Sources: Adapted from data published in Wyngaarden J.N., Woods L.A., Ridley R., Seevers M.H. 1949. Anesthetic properties of sodium-5-allyl-5-(1-methylbutyl)-2-thiobarbiturate (Surital) and certain other thiobarbiturates in dogs. J Pharmacol Exp Ther 95:322; and Branson K.R. 2007. Injectable and alternative anesthetic techniques. In: Lumb and Jones’ Veterinary Anesthesia and Analgesia, 4th ed. W.J. Tranquilli, J.C. Thurmon, and K.A. Grimm, eds. Ames, IA: Blackwell Publishing, p. 284.

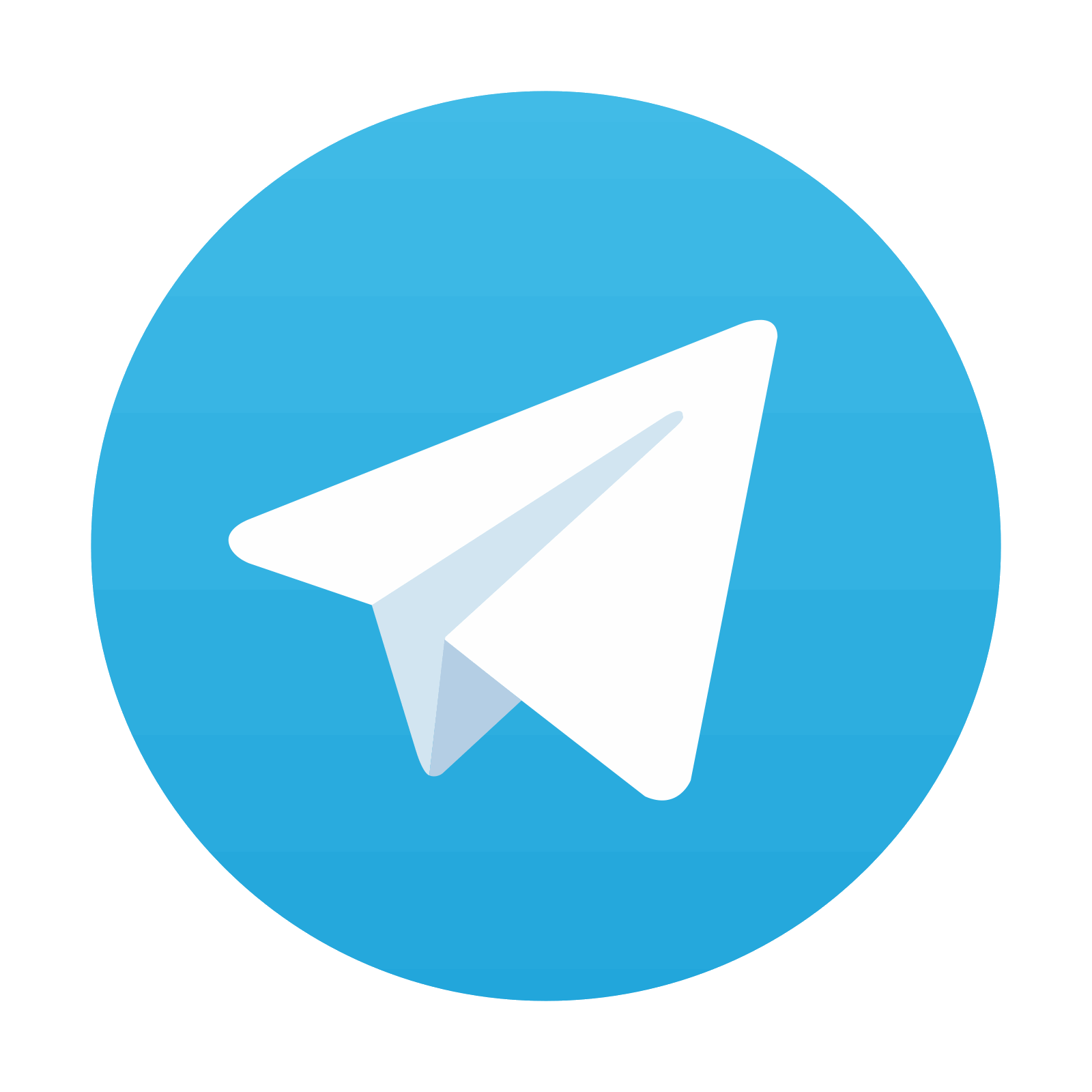
Stay updated, free articles. Join our Telegram channel
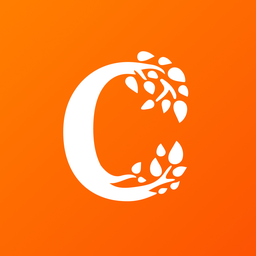
Full access? Get Clinical Tree
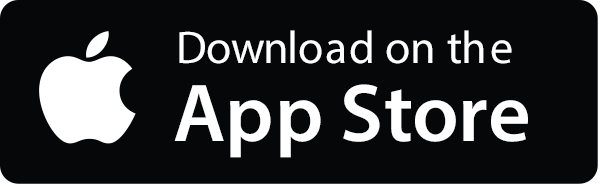
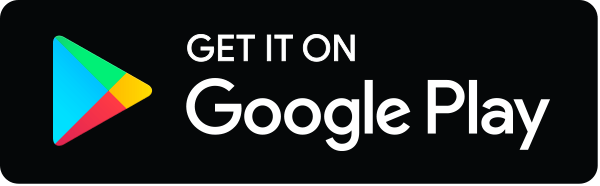