Erik H. Hofmeister Department of Clinical Sciences, College of Veterinary Medicine, Auburn University, Auburn, Alabama, USA An adverse event during anesthesia is defined as an event that may result in patient harm and may be due to the effects of anesthetic drugs, the patient’s condition, the diagnostic or therapeutic procedure being conducted, or human error. These events are often emergencies due to the narrow therapeutic margins of drugs used to induce anesthesia, the co‐opting of normal homeostatic mechanisms, and the manipulation of highly sensitive key organ systems such as the circulatory, respiratory, and neurologic systems. Rapid intervention is often needed before such an event leads to an adverse patient outcome. Rates of adverse events differ among species, country, and across published studies. For example, the risk of death across species ranges from 1 in 2000 for dogs to 1 in 29 for birds [1,2]. The timeframe of what constitutes an anesthetic‐related adverse event varies, ranging from an occurrence during anesthesia to any event occurring within seven days of anesthesia. Further information is available in Chapter 2. The consequences of adverse events range from minimal to substantial. For example, a short episode of myoclonus during anesthesia is unlikely to affect a patient’s long‐term welfare, whereas gastroesophageal reflux leading to esophageal stricture will have significant negative effects on patient well‐being. Death is often considered to be the worst possible outcome, although significant morbidity and suffering may arguably be worse in some cases. Adverse events can be categorized according to a variety of criteria. These include the frequency or rate of occurrence, the severity of consequences to the patient, the organ system involved, and/or the causative circumstances. It is impractical to use frequency and severity to categorize adverse events as they are not always known in veterinary medicine. Organ system designations may not capture all adverse events, such as drug overdose or machine error. Therefore, a causative circumstances framework will be used in this chapter, broadly considering that cardiovascular or respiratory events may be caused by a wide variety of circumstances. The anesthetist should be prepared to rapidly identify and manage adverse events. A lack of awareness of any of these complications will almost certainly impair identification of the problem. Typically, when faced with a problem, people use cognitive shortcuts to determine if the scenario matches a pattern they have seen previously [3]. If it is not a pattern with which they are familiar, higher order cognitive processing, which is complex and time‐consuming, must be employed. Because of the rapid intervention required for many anesthetic emergencies, familiarity with them so that pattern recognition can be employed in problem‐solving is essential. Awareness of these events and understanding how to identify and manage them is a requirement for developing expertise in veterinary anesthesia. Cardiopulmonary arrest (CPA) describes the acute cessation of cardiac mechanical function with concurrent apnea [4] resulting in termination of oxygen delivery to tissues and death if not addressed rapidly. A variety of pathologic arrhythmias are associated with CPA, with treatment varying among them. The goal of cardiopulmonary resuscitation (CPR) is to provide effective blood flow to the heart and brain to facilitate return of spontaneous circulation (ROSC). CPR involves basic life support (BLS), which encompasses the initial steps taken in the face of CPA, and advanced life support (ALS), which involves drug therapy and more elaborate and specific treatments and monitoring during CPR. Postresuscitation care is an essential component of CPR, as merely succeeding to achieve ROSC does not guarantee successful patient discharge from the hospital [5]. CPR has overwhelmingly poor outcomes in both human and veterinary medicine. Reported rates of initial ROSC in dogs and cats are 17–58% and 21–57%, respectively [5–7]. Unfortunately, after CPR, the overall survival‐to‐discharge rates drop to 4–7% in dogs, 3–19% in cats, and 1.2% in exotic species [5–8] for all cases. The rates of ROSC and discharge are unknown in horses, although anecdotally the prognosis for foals is better than adult horses [9]. Successful ROSC and discharge after CPA in adult horses are sufficiently rare to warrant publication as case reports [10]. The prognosis for anesthetized small animals is notably higher, with 25–50% of patients having CPA under anesthesia surviving to discharge [5,6]. This difference may be due to the fact that CPA under anesthesia may be precipitated by human errors, rather than the severity of patient disease [11]. In addition, anesthetized patients usually have venous access established, are intubated and breathing enriched oxygen rather than room air, and are being monitored continuously, facilitating more rapid recognition of CPA [6]. In spite of the grave prognosis for CPR after CPA, one study reported that veterinary clients believe the rate of discharge after CPA to be 39% and do not believe that there is a difference in discharge rate between patients who suffer CPA while under anesthesia versus those not under anesthesia [12]. Most clients indicated that they want to discuss CPR with their veterinarian, and most want the veterinarian to make the decision to resuscitate [12]. This may explain why the euthanasia rate following ROSC is so high [7], as clients may not appreciate the low chance of discharge, the costs associated, or had an opportunity to discuss CPA/CPR with their veterinarian in advance. Given the risk of arrest during anesthesia, the likelihood of successful discharge, and client misinformation, it behooves the anesthesia team to communicate with the client about CPA before anesthetizing a patient. Increased time from CPA to initiating CPR and duration of CPR decreases the likelihood of ROSC [5,6]. To ensure the highest chance of success, hospital staff should be prepared and trained to respond to the crisis quickly and efficiently. Training has been shown to improve adoption of activities that benefit CPR efforts [13]. During treatment of an arrest, the veterinarian in charge will usually take a central role coordinating the resuscitation procedures (i.e., running the code). It is important that the anesthesia team is well trained in current CPR procedures [14]. Regardless of initial training, there is always time‐related decay of skills. Refresher training and mock CPR simulation every 6 months are recommended [4]. The necessary emergency drugs and equipment should be organized in one place (e.g., crash cart) and should be adequately stocked, regularly audited, and placed in a readily accessible area. This should include supplies for venous access, airway management, and initial pharmacological therapy. It is also wise to have a surgical kit for emergency thoracotomy, tracheostomy, and venous dissection available. Equipment failures during CPR were commonly cited as a deficiency in one veterinary study [15]. Therefore, regular testing and resupply of the crash cart should be conducted. In 2012, the Reassessment Campaign on Veterinary Resuscitation (RECOVER) was organized to develop comprehensive, evidence‐based CPR guidelines for veterinary medicine. It was a collaborative initiative involving Diplomates of the American College of Veterinary Anesthesia and Analgesia (ACVAA) and the American College of Veterinary Emergency and Critical Care (ACVECC) who systematically evaluated the published evidence relevant to small animal CPR and generated CPR guidelines (Fig. 5.1) [16,17]. Implementation of the RECOVER guidelines has been associated with an improved outcome in one study [18] but not in another [7]. Regardless, all hospital staff involved in CPR, particularly anesthesia and emergency staff, should be aware of these guidelines. One study indicated that, while specialists are aware of the guidelines, many general practitioners are not [19]. This suggests an education gap, which should be closed through the veterinary education curriculum and continuing education programs. Basic life support (BLS) is the foundation for all modern CPR. It consists of recognition of CPA, initiation of chest compressions, management of the airway, and assisted ventilation [20]. Studies have shown that the quality of BLS performed is directly related to ROSC and survival rate; thus, early and sustained chest compressions are recommended [20]. When multiple trained rescuers are available, initiation of chest compressions and efforts to secure the airway can occur simultaneously, and subsequent ventilation can be delivered concurrent with chest compressions. Chest compressions should begin as soon as possible to facilitate effective blood flow to the heart and brain. Quality compressions with effective recoil are essential to optimize blood flow [20]. Chest compressions should be performed continuously without interruption by each resuscitator until a change in personnel is indicated to maintain compression quality. A compression rate of at least 100 per minute should be sustained. Use of a mental metronome (such as a song with a beat per minute rhythm of 100) improves the accuracy of chest compression rates in veterinary students [21] and could be a valuable aid in CPR team training. Myocardial blood flow is determined by the coronary perfusion pressure (CoPP), defined as the difference between aortic diastolic and right atrial diastolic pressures: Figure 5.1 RECOVER CPR Algorithm. This algorithm summarizes clinical guidelines most relevant to the patient presenting acutely in CPA. The box surrounded by the gray dashed line contains, in order, the initial BLS and ALS actions to be taken when a patient is diagnosed with CPA: (1) administration of chest compressions, (2) ventilation support, (3) initiation of ECG and PETCO2 monitoring, (4) obtaining vascular access for drug administration, and (5) administration of reversal agents if any anesthetic/sedative agents have been administered. The algorithm then enters a loop of 2‐min cycles of CPR with brief pauses between to rotate compressors, to evaluate the patient for signs of ROSC, and to evaluate the ECG for a rhythm diagnosis. Patients in PEA or asystole should be treated with vasopressors and, potentially, anticholinergic drugs. These drugs should be administered no more often than every other cycle of CPR. Patients in VF or pulseless VT should be electrically defibrillated if a defibrillator is available or mechanically defibrillated with a precordial thump if an electrical defibrillator is not available. Immediately after defibrillation, another 2‐min cycle of BLS should be started immediately. BLS, basic life support; CPA, cardiopulmonary arrest; CPR, cardiopulmonary resuscitation; C:V, compression‐to‐ventilation ratio; ETCO2, end‐tidal carbon dioxide; PEA, pulseless electrical activity; ROSC, return of spontaneous circulation; VF, ventricular fibrillation; VT, ventricular tachycardia. Source: Fletcher et al. [17], with permission. where ADP is aortic diastolic pressure and RADP is right atrial diastolic pressure. Cerebral blood flow correlates with cerebral perfusion pressure (CEPP): where MAP is the mean arterial pressure and ICP is intracranial pressure. Therefore, interventions that increase diastolic or mean arterial pressures or decrease RADP and/or ICP are beneficial to patients undergoing CPR. Cerebral blood flow in healthy animals is maintained at a fairly constant level when cerebral perfusion pressure is between 50 and 150 mmHg. During cardiac arrest, autoregulation of cerebral perfusion is disrupted and cerebral blood flow becomes linearly related to perfusion pressure. Optimizing the patient’s hemodynamic status during CPR is essential to minimize the risk of adverse cardiac and neurologic outcomes [22]. Chest compressions in animals should be performed by compressing the chest by one‐third to one‐half of its width, with a greater depth of compression being associated with improved aortic pressures [20]. An appropriate chest compression technique also improves the pressure gradient between the aorta in diastole and the right atrium, which augments coronary perfusion and increases the probability of ROSC [20]. There are two theories that explain the mechanism of forward blood flow with chest compressions: the thoracic pump theory and the cardiac pump theory. The thoracic pump describes movement of blood out of the thoracic cavity due to changes in intrathoracic pressure (IttP). This mechanism is thought to be important in animals with a body weight over 15 kg [20]. The cardiac pump describes movement of blood out of the heart due to direct, mechanical compression of the heart by the thoracic wall [20]. After release of the compression, both the thoracic cavity and the heart itself recoil (i.e., chest recoil) during the decompression phase. The decompression phase is critical to successful ROSC as this is when the heart fills with blood, which facilitates effective stroke volume [20]. If the chest is not allowed to recoil fully, IttP remains positive, which decreases venous return to the heart and, hence, stroke volume [20]. As noted above, continuous administration of chest compressions with minimal interruption is essential. Even short pauses to evaluate the electrocardiogram (ECG), for example, decrease the aortic pressure and reduce the likelihood of successful ROSC. CPR should be performed in a 2‐min cycle, with a resuscitator doing chest compressions for the full 2 min before exchanging with a different resuscitator. Performing high‐quality chest compressions is fatiguing, requiring regular exchange of resuscitators to maintain compression effectiveness [20]. If the same resuscitator performs chest compressions for an extended period of time (e.g., 5 min), the number of correct chest compressions delivered has been shown to decrease [20]. In cats and small dogs, two‐handed compression over the heart or one‐handed compression around the base of the heart may be used. In larger dogs, two‐handed compression over the heart (for keel‐chested dogs) or over the widest part of the thorax (for round‐chested dogs) is recommended [4]. Two‐handed compression can also be used in foals. In adult horses, using the knees or sitting intermittently on the thorax may be used [10]. Chest compressions, as well as other manipulations associated with CPR, can cause pulmonary hemorrhage and liver fractures [23]. After ROSC, the patient should be evaluated for trauma, including ultrasound of the thoracic and abdominal cavity, and any damage appropriately addressed. If multiple rescuers are present, interposed abdominal compression (IAC)‐CPR can be performed where conventional chest compressions are combined with abdominal compressions delivered during the relaxation phase. While interposed abdominal compression has been shown to improve venous return and hemodynamic values in animal CPR models [20], in an actual clinical setting, it has been associated with a lower likelihood of success [24]. Attempting to synchronize abdominal and chest compressions while maintaining a fast compression rate may actually impair chest compressions, and this may explain why the beneficial effects in the laboratory do not translate to clinical CPA cases. Open‐chest CPR is recommended in cases where closed‐chest CPR is ineffective or in cases of elevated IttP such as pleural space disease, pericardial disease, severe abdominal distension, or flail chest. With closed‐chest compressions, the cardiac output generated is only 20–30% of normal. Performing a thoracotomy for open‐chest CPR will not only increase cardiac output but also provides an opportunity to occlude the descending aorta, which redirects blood flow to essential organs (i.e., brain and heart). Concern about infection risk is often cited as a cause for delay in initiating open‐chest CPR, but one human study found this risk to be very low [25]. The exact surgical approach through the thoracic wall does not need to be perfect, as either the fourth or fifth intercostal space has been found to be acceptable in dogs [26]. Securing the airway and providing adequate ventilation is essential during CPR [20]. The most expedient technique for accomplishing this is usually via orotracheal intubation. If prompt orotracheal intubation is not possible (e.g., for out‐of‐hospital arrest), ventilation via a face mask or mouth‐to‐nose can be performed; however, these techniques are associated with significantly lower PaO2 values and higher PaCO2 values than those achieved with orotracheal intubation [27]. In people, even experienced personnel have only an 82% first‐time intubation success rate during CPR [28]. Intubation should be confirmed by direct laryngoscopy or, if impractical, by auscultation of air movement in the lungs, observation of chest wall movement, or condensation within the endotracheal tube [29]. After the airway has been secured, the patient should be ventilated manually with the maximal available inspired oxygen concentration (i.e., FIO2~1.0). Although current guidelines suggest a ventilation rate of 10 breaths/min without interruption of chest compressions [20], a ventilation rate as low as one breath every 30 compressions (i.e., 3 breaths/min) is effective at maintaining oxygen and carbon dioxide at appropriate levels while minimizing detrimental effects on compression mechanics [30]. A full breath should be delivered in approximately 1 s with a 10 mL/kg tidal volume. A prolonged inspiratory time subjects the patient to a high IttP for an extended period, which decreases venous return, increases right ventricular afterload, and decreases left ventricular distensibility [31]. Advanced life support (ALS) involves drug therapy, more elaborate and specific treatments (e.g., correction of electrolyte disturbances), and defibrillation when indicated [32]. Pharmacologic therapy has been shown to improve outcomes in CPA (Table 5.1). Some drugs are indicated in most instances of CPA (e.g., epinephrine), whereas the decision to administer others depends on patient‐specific factors (e.g., administering calcium to a patient with profound hypocalcemia). Drugs should be given by the route that facilitates the most rapid delivery to the heart. The order of preference for drug administration routes in CPR is: (1) central venous line, (2) forelimb intravenous catheter, (3) any other peripheral intravenous catheter, (4) intraosseous catheter, and (5) intratracheal delivery (for epinephrine, vasopressin, or atropine only). If epinephrine, atropine, or vasopressin is given by the intratracheal route, the dose should be increased (by 10‐fold in the case of epinephrine), and it should be delivered via a long catheter (e.g., red rubber) advanced to the level of the carina [32]. Vasopressors are widely employed during veterinary CPA events to increase aortic pressures by increasing systemic vascular resistance and directing more of the intravascular volume from the peripheral circulation to the central compartment [32]. Both epinephrine and vasopressin are reasonable choices for all types of cardiac arrest. At the correct dosage, epinephrine exerts its critical vasoconstrictor effects by acting on vascular α‐adrenergic receptors. Epinephrine’s β‐adrenergic effects (i.e., inotropy and chronotropy) are less important and may actually lead to detrimental increases in myocardial oxygen consumption once ROSC is achieved [32]. Vasopressin is a non‐catecholamine vasopressor that acts on V1 receptors in peripheral vessels while decreasing cellular hyperpolarization and increasing intracellular calcium concentration. In a study in dogs, similar outcomes were obtained with vasopressin and epinephrine [33]. A high dose (0.1 mg/kg) of epinephrine is no longer recommended for CPR. Table 5.1 Pharmacotherapy during cardiopulmonary resuscitation (CPR). IV, intravenous; VT, ventricular tachycardia; VF, ventricular fibrillation; CPA, cardiopulmonary arrest. For animals with high vagal tone (e.g., vomiting, ileus) and subsequent bradycardia/asystole, the use of atropine is reasonable [32]. Evidence supporting atropine’s routine use in CPR is sparse, but, as it has not been associated with worse outcomes, such use is considered acceptable. Although now‐outdated recommendations did not support the routine use of corticosteroids, a systematic review in people provides evidence for the value of steroids in CPR [34]. This is consistent with a study in veterinary medicine, which documented a beneficial effect of steroids [5]. Potential beneficial effects of corticosteroids in CPA may be a result of counteracting relative adrenal insufficiency in patients with longstanding critical disease [35], counteracting impairment of adrenal function as a result of CPA, exerting anti‐inflammatory effects, improving cardiovascular function, or blunting a catecholamine surge [34]. Lidocaine administration may be associated with vasodilation and a reduction in cardiac output. In pulseless ventricular tachycardia (VT) and ventricular fibrillation (VF), early and rapid defibrillation is advised. In dogs with shock‐resistant VT or VF, amiodarone is preferred over lidocaine, although hypotension and anaphylactic reactions have been reported in this species following amiodarone administration [32]. The routine use of sodium bicarbonate during CPR is not recommended [32]. Bicarbonate administration may cause paradoxical cerebral acidosis, hyperosmolarity, and decreased catecholamine effectiveness. Bicarbonate use may be considered in prolonged CPA (i.e., greater than 10–15 min) or for CPA due to severe hyperkalemia or severe metabolic acidosis. The routine use of calcium during CPR is not recommended [32]. The use of calcium may be considered for CPA due to calcium channel blocker overdose, severe hypocalcemia, or severe hyperkalemia. Calcium administration may cause cellular apoptosis with impaired neurological outcome and myocardial damage. Fluid therapy is essential if CPA was caused by hypovolemia or hemorrhage. However, in euvolemic patients, aggressive fluid therapy may be detrimental due to decreased tissue blood flow caused by compromised tissue perfusion pressures [36]. In one study in euvolemic dogs with induced CPA, 11 mL/kg of either Lactated Ringer’s solution or whole blood increased cardiac output but decreased both coronary and cerebral perfusion because of the disproportionate increase in RADP compared to ADP [37]. In the face of ventricular fibrillation (VF), chest compressions should be initiated to circulate oxygenated blood to the myocardium before attempting electrical conversion with defibrillation. A hypoxic myocardium is unlikely to be successfully defibrillated. It is particularly important to initiate chest compressions before defibrillation if there is a delay between identification of CPA and administration of defibrillation. Immediately after a defibrillation attempt, chest compressions should be resumed for another full CPR cycle. Electrical defibrillation should start at 2–5 J/kg. If unsuccessful, a 2‐min cycle of CPR should proceed; then, defibrillation attempted with a 50% increase in energy. This cycle, with concomitant increases in energy, continues until the rhythm changes (e.g., asystole develops) or ROSC is achieved. If anesthesia‐related CPA is due to drug overdose, reversal agents should be administered if available. Agents such as naloxone, flumazenil, and atipamezole should be administered in the case of opioid, benzodiazepine, or α2‐adrenergic receptor agonist overdose, respectively. All inhalant anesthetics cause myocardial depression, and their use should be terminated during CPR. Lipid rescue may be considered in animals with CPA due to local anesthetic overdose (see Chapter 29). If CPA occurs during an abdominal surgery, consideration should be given to performing open‐chest CPR by accessing the heart through the diaphragm. Several devices have been invented and tested to improve cardiac output during CPR. There are adjunctive CPR devices that actively decompress the chest during the expansion phase after delivery of a compression, devices that automate chest compressions, and devices that increase negative IttP during chest recoil to facilitate venous return (i.e., the impedance threshold device or ITD). Although the ITD has been shown to be beneficial in improving carotid blood flow and coronary perfusion in dogs undergoing CPR [22], there is currently no compelling evidence that any of these devices improve outcomes in people or animals [38]. Accurate and rapid identification of patients experiencing CPA is essential to ensure prompt initiation of CPR efforts, which directly impacts outcome. Monitoring the effectiveness of CPR efforts during CPR is critical to ensure that hemodynamic and respiratory goals are being met. Monitoring also provides important patient prognostic information and should guide postresuscitation planning and care. Animals often experience agonal gasps or apnea as a prelude to CPA [5]. Other indications include collapse, a fixed gaze, lack of a palpable pulse, loss of an audible signal from a Doppler device (usually in an anesthetized patient), a rapid decrease in end‐tidal carbon dioxide tension (PETCO2) (in an anesthetized, intubated patient), observation of a characteristic arrhythmia on ECG, hypoventilation, and/or seizures. If CPA is suspected, the patient should be rapidly evaluated by auscultation of heart sounds. Seeking a peripheral pulse via palpation or placement of a Doppler probe delays the start of treatment and is not recommended to identify CPA [29]. The loss of sound from a preplaced Doppler device may precede CPA and should be investigated immediately [29]. Valuable time to initiate CPR efforts should not, however, be lost by checking the Doppler device or readjusting the probe. Other causes of a lost Doppler signal (e.g., a closed adjustable pressure‐limiting [APL] valve) should also be investigated. In anesthetized, intubated patients, a rapid decrease in PETCO2 can indicate a precipitous drop in cardiac output preceding cardiac arrest [29]. Observation of an abrupt decline in PETCO2 should be taken as a strong indicator of actual or impending CPA, and the patient should be assessed immediately. Once BLS steps have been initiated, ECG leads should be attached to identify if a treatable arrhythmia (e.g., bradycardia, ventricular fibrillation) is present. Continued ECG monitoring is warranted as patients may transition to a treatable arrhythmia during CPR efforts. The ECG can be checked during the change‐over in personnel performing compressions every 2 min. Ceasing chest compressions more regularly to check the ECG rhythm is not recommended [29]. PETCO2 monitoring should be instituted as it has a proven association with CPR outcomes [39]. PETCO2 is a non‐invasive surrogate for cerebral perfusion pressure and values ≥ 18 mmHg between 3 and 8 min after starting CPR is a sensitive predictor for ROSC [39]. If CPR efforts are not generating adequate PETCO2 values, changes may need to be made (e.g., change personnel performing chest compressions and/or convert to thoracotomy for open‐chest CPR). Continued monitoring of ventilation parameters should include ensuring thoracic wall movements are occurring, respiratory rate is not exceeding 10 breaths/min, inspiratory time is 1 s, and inspiratory pressure is not excessive [29]. The respiratory rate, in particular, is often higher than is recommended even with experienced personnel. Venous blood gas monitoring can be used to inform the likelihood of ROSC and provide information about tissue perfusion and electrolytes (e.g., hypocalcemia), which may require targeted treatment. During and after CPR, oxygenation goals are to maintain a PaO2 of 80–100 mmHg or a saturation of 94–98%. Long‐term hyperoxemia (i.e., PaO2 > 100 mmHg) is undesirable as it can cause oxygen free‐radical formation. After ROSC, hypoventilation is a common complication due to respiratory muscle weakness or poor responsiveness of the brainstem to CO2 levels. A target PaCO2 of 32–40 mmHg is desired and can be achieved with mechanical ventilation if the patient is not able to adequately ventilate spontaneously. Following ROSC, numerous body systems suffer injury secondary to interrupted blood flow and subsequent ischemia‐reperfusion injury [40]. This constellation of problems is referred to as “postcardiac arrest syndrome” and includes damage to the brain, kidneys, and heart, as well as derangements of coagulation. Following the development of postcardiac arrest syndrome, other large‐system inflammatory conditions, such as systemic inflammatory response syndrome (SIRS) and acute respiratory distress syndrome (ARDS) can develop. Ischemia‐reperfusion in the brain leads to mitochondrial dysfunction, excitotoxicity, altered phospholipids, oxidative stress, increased lactate, endoplasmic reticulum stress, and inflammation [41]. Mortality (i.e., second arrest) is very common in the first 24 h after resuscitation [6]. Postcardiac arrest care should be intensive with diligent patient monitoring. Continuous or serial monitoring of the ECG, arterial oxygenation (SpO2), body temperature, blood glucose, and systemic blood pressure should be instituted, as well as serial physical examinations and assessment of neurologic status [29]. Therapeutic protocols are based on hemodynamic optimization, cerebral protection, and therapeutic hypothermia [40]. Hemodynamic optimization aims to maintain blood pressure and, therefore, cerebral perfusion pressure. Numerous studies have found an association between arterial blood pressure and outcome in people [42–44]; however, the “optimal” blood pressure following ROSC has not been clearly defined. Targeting a mean arterial blood pressure (MAP) in the range of 70–80 mmHg with the use of inotropes and vasopressors seems to be associated with improved outcomes. Cerebral protection can be facilitated by the use of a variety of pharmacologic interventions. Corticosteroids seem to offer some benefit, potentially due to relative adrenal insufficiency following CPA [41]. Numerous other interventions that target specific pathways of cerebral ischemia‐reperfusion‐induced dysfunction are being investigated, but evidence supporting their clinical use is lacking. Mannitol is often used to minimize cerebral edema following ROSC, but the evidence for its use is not particularly compelling. Therapeutic hypothermia has been used in people for decades, and there is strong evidence for its routine inclusion in postresuscitation care [45]. Beneficial effects of therapeutic hypothermia include reductions in cerebral oxygen requirement, brain metabolic demand, excitatory neurotransmitters, inflammatory cytokines, and free radicals, along with inhibition of neuronal cell apoptosis [41]. Potential complications include patient discomfort, longer tissue healing time, and coagulopathy. It appears that early application of even mild hypothermia (e.g., 36 °C/97 °F) is beneficial in the postresuscitation period and avoidance of hyperthermia is associated with improved survival in people. The anesthetic complications involving the cardiovascular system discussed below are obviously not mutually exclusive and may occur alongside, or as a result of, many of the other complications presented in this chapter. Hypotension has been reported to occur in 7–59% of anesthetized small animal patients [46–48]. The incidence in other species is also high, at 79% for rabbits [49] and 55% for horses [50]. While this complication occurs commonly in anesthetized animals, especially those anesthetized with inhalant agents, effective anticipation, recognition, and management means that it infrequently develops into a true anesthetic emergency. Pre‐existing conditions associated with hemodynamic instability, surgical manipulations (e.g., compression of the vena cava), and various drugs can all precipitate or exacerbate significant hypotension and may present challenges for the anesthetist. The adverse effects of hypotension are related to both its severity and duration. Relatively mild hypotension may be tolerated for some time, whereas profound hypotension for even 15 min may result in clinically significant morbidity. Classically, it is believed that maintaining a MAP over 60 mmHg maintains visceral organ perfusion [51,52], a MAP over 70 mmHg maintains muscle perfusion in large animals (e.g., adult horses) [53], and a diastolic arterial pressure (DAP) over 40 mmHg maintains myocardial perfusion [54]. Consciousness typically requires a systolic arterial pressure (SAP) over 50 mmHg [55]. Blood pressure is directly dependent on cardiac output and systemic vascular resistance (SVR). Cardiac output, in turn, depends on heart rate and stroke volume with the major determinants of stroke volume being preload, contractility, and afterload. Therefore, hypotension results from alterations to, or abnormalities of, any of these five variables (i.e., preload, contractility, afterload, heart rate, and SVR), and management of hypotension is directed at manipulating one or more of these. Continuous monitoring of blood pressure is indicated in any patient undergoing general anesthesia. Direct arterial blood pressure monitoring is indicated in all large animal cases anesthetized with inhalant anesthetics as well as any patients with the potential for hemodynamic instability during anesthesia. Monitoring blood pressure has been associated with a lower risk of cardiac‐related mortality in horses [56]. Figure 5.2 Clinical approach to hypotension. Note that other considerations, such as pre‐existing cardiac disease, concurrent drug administration, blood volume status, and concurrent non‐cardiac disease, may affect this generic approach. A simple flowchart outlining the clinical approach to hypotension is presented in Fig. 5.2. Note that other considerations, such as pre‐existing cardiac disease, concurrent drug administration, blood volume status, and concurrent non‐cardiac disease may affect this simple approach. Further information is provided in Chapters 12, 32, 33, and 36. Acute blood loss has two significant impacts on oxygen delivery to tissues: decreased preload and decreased hemoglobin concentration. Hemorrhage prior to or during anesthesia increases the risk of hemodynamic instability. Patients who have suffered hemorrhage prior to anesthesia should be stabilized if at all possible before the start of anesthesia. Those with severe ongoing hemorrhage requiring immediate surgical intervention should be aggressively treated during the preanesthetic period and throughout the maintenance phase of anesthesia. In dogs undergoing anesthesia and surgery, the volume of hemorrhage has been associated with increased cost of care, with each mL of blood loss contributing $2 to the cost [57]. In dogs undergoing thoracic surgery, intraoperative blood product use (presumably due to hemorrhage) was associated with a 4.4‐fold increase in the likelihood of postanesthetic death [58]. Similarly, in dogs undergoing emergency abdominal surgery, blood product use increased the risk of death by sevenfold [59]. These studies support the practice of hemodynamic stabilization prior to anesthesia whenever possible and highlight the negative impacts of acute intraoperative hemorrhage on patient outcomes. The indications for treating hemorrhage include absolute blood volume loss, increased base excess or lactate, and changes in cardiovascular parameters associated with hemorrhage including relative increases in heart rate and decreases in systolic blood pressure (i.e., the shock index; HR/SAP). For absolute blood volume loss, treatment is recommended once estimated blood loss approaches 20% of total blood volume, or approximately 17 mL/kg in the dog [60]. Direct observation of intraoperative blood loss during the surgical procedure facilitates estimation of the volume lost and should be closely monitored. A base excess > 6.6 or a lactate > 5.0 mmol/L may indicate the need for treatment. In the awake dog, a shock index (HR/SAP) > 1.0 may be a more sensitive and specific indicator of 18% blood volume loss than heart rate or blood pressure alone [61]. General anesthesia may complicate interpretation of variables typically associated with the need to treat hemorrhage. For example, inhalant‐induced hypotension and concurrent tachycardia due to intense surgical stimulation may make clinical decision‐making more challenging. Volume replacement may consist of crystalloids, colloids, and/or blood products, and should be initiated as early as possible after recognition of blood loss. Further information is available in Chapter 32. The incidence of clinically significant cardiac dysrhythmias in healthy dogs under anesthesia is as high as 18% [62]. Cardiac dysrhythmias have the potential to decrease cardiac output by affecting diastolic filling (and hence venous return), contractility, and/or heart rate. They also have the potential to increase myocardial work by increasing heart rate above physiologic levels. Many arrhythmias occur in the absence of cardiac disease and may be precipitated by anesthesia, but most are clinically insignificant. However, the appearance of some arrhythmias under anesthesia may be more serious and warrant prompt recognition and intervention (e.g., atrial fibrillation and third‐degree atrioventricular block). Specific treatment of an arrhythmia is generally undertaken if cardiac output is adversely affected or if increased myocardial work is putting excessive stress on the heart. Impacts on cardiac output are typically inferred based on arterial blood pressure measurement, although clinical signs such as syncope in a conscious patient may also indicate insufficient cardiac output. Increases in myocardial work caused by a tachyarrhythmia may be particularly problematic in patients with diastolic cardiac dysfunction (e.g., hypertrophic cardiomyopathy). Treatment is aimed at correcting the underlying cause of the arrhythmia (e.g., placement of a pacemaker in a patient with third‐degree atrioventricular block) and/or pharmacologic manipulation of cardiac conduction. Further information is available in Chapters 11, 35, and 36. While uncommon, anaphylactic or anaphylactoid reactions may occur in the perianesthetic period and constitute adverse events. True anaphylaxis is a type I hypersensitivity reaction caused by rapid immunoglobulin E (IgE)‐mediated interactions. Anaphylactoid reactions mimic anaphylaxis but are not caused by IgE. Both types of reactions cause release of substances by mast cells and basophils, primarily histamine. This results in a variety of effects, including pruritus, urticaria, hypotension, tachycardia, bronchospasm, and vomiting and diarrhea. Anaphylactic reactions can be caused by the patient encountering an antigen, which triggers anaphylaxis or by pharmacologic substances. Topical cleansing agents, antibiotics, radiographic contrast agents, and atracurium are common culprits in an anesthetic setting. In addition to symptomatic treatment to support cardiorespiratory function, epinephrine can be given IM at 0.01 mg/kg. Many of the respiratory complications discussed below overlap and have cause‐and‐effect relationships with one another. For example, hypoxemia is a common sequela to many of the complications listed but general considerations for hypoxemia are also presented in its own section. Respiratory depression (i.e., hypoventilation) is defined as inadequate CO2 elimination that is detected as an increase in the partial pressure of arterial carbon dioxide (PaCO2) or end‐tidal carbon dioxide (PETCO2). It is important to recognize that significant hypoventilation may occur in the face of a “normal” respiratory rate with few other associated clinical signs; thus, the only way to reliably identify hypoventilation is to measure CO2. Although hypoventilation (i.e., PETCO2 > 45 mmHg) is relatively common, severe hypoventilation (i.e., PETCO2 > 60 mmHg) is relatively uncommon with one large‐scale retrospective canine study reporting it in ~ 9% of patients [47]. Apnea refers to the complete absence of breathing and is the extreme endpoint on the spectrum of respiratory depression. While it can occur at any point during the perianesthetic period, apnea immediately following induction of anesthesia is common, depending on the anesthetic protocol and administration technique, with reported incidence rates in dogs as high as 75% [63]. Respiratory depression/apnea as an anesthetic complication spans a continuum ranging from mild hypercapnia, which may require no intervention, to apnea which requires immediate endotracheal intubation (if not already done) and ventilatory support. In addition to the direct effects of hypercapnia (e.g., respiratory acidosis, tachycardia, etc.), hypoventilation induces indirect effects including the potential for hypoxemia and impaired uptake of inhalant anesthetics. Acidosis can disrupt enzyme systems, decreasing cardiac contractility, and reducing the effectiveness of certain drugs (e.g., inotropes) [64,65]. Hypercapnia can also precipitate arrhythmias, hypertension, hypoxemia, narcosis, and vasodilation with subsequent hypotension and increased intracranial pressure (ICP) [66]. The risk of hypoventilation producing hypoxemia depends on the inspired oxygen concentration (FIO2). With an FIO2 of 1.0, PETCO2 would need to be > 500 mmHg to lower PaO2 below 60 mmHg. This scenario is not clinically likely. However, with an FIO2 of 0.21 (i.e., a patient breathing room air), hypoventilation resulting in a PETCO2 of ~70 mmHg will cause hypoxemia. Profound hypercapnia exerts a MAC‐sparing effect starting at ~ 94 mmHg and linearly decreases MAC until it reaches 240 mmHg, at which point CO2 achieves anesthesia alone [66]. By extension, the time to onset of hypoxemia due to apnea is also dependent on FIO2. In dogs preoxygenated with 88% oxygen for 3 min and then induced with propofol (causing subsequent apnea), SpO2 values remained above 90% for an average of almost 5 min, compared with dogs without preoxygenation where SpO2 values fell below 90% by 70 s [67]. Thus, provision of enriched oxygen immediately before induction of anesthesia will delay the onset of hypoxemia due to apnea or profound hypoventilation compared to inspiration of room air. Inhalant anesthetics move down a series of partial pressure gradients from the anesthetic circuit to the alveoli and back for uptake and elimination. During apnea or profoundly decreased ventilation, uptake and elimination of the inhalant anesthetic may be impaired [68]
5
Anesthetic Emergencies, Resuscitation, and Adverse Events
Introduction
Cardiopulmonary arrest
Basic life support
Chest compressions
Breathing
Advanced life support
Pharmacologic therapy
Drug
Dose regimen
Comments
Epinephrine
0.01 mg/kg IV every 3–5 min
Use acceptable in most cases of CPA; causes peripheral vasoconstriction and increased aortic pressure
Vasopressin
0.8 IU/kg IV every 5 min
Use acceptable in most cases of CPA; causes peripheral vasoconstriction and increased aortic pressure
Corticosteroid
1 mg/kg IV prednisone equivalent
Use acceptable in most cases of CPA
Atropine
0.05 mg/kg IV every 5 min
Routine use is not recommended but is acceptable
Lidocaine
2 mg/kg IV
Routine use is not recommended but may be used in shock‐resistant VT or VF
Amiodarone
5 mg/kg IV
Routine use is not recommended but may be used in shock‐resistant VT or VF
Sodium bicarbonate
1 mEq/kg IV
Routine use is not recommended but may be used in prolonged CPA or CPA due to severe hyperkalemia or severe metabolic acidosis
Calcium gluconate
50 mg/kg IV
Routine use is not recommended but may be used in CPA due to calcium channel blocker overdose, severe hypocalcemia, or severe hyperkalemia
Defibrillation
Anesthesia‐related CPA and CPR
Adjunctive CPR techniques and devices
Monitoring
Identifying CPA
Monitoring during CPR
Postresuscitation care
Cardiovascular complications
Hypotension
Hemorrhage
Cardiac dysrhythmias
Anaphylaxis
Respiratory complications
Respiratory depression and apnea
Stay updated, free articles. Join our Telegram channel
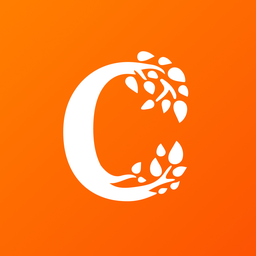
Full access? Get Clinical Tree
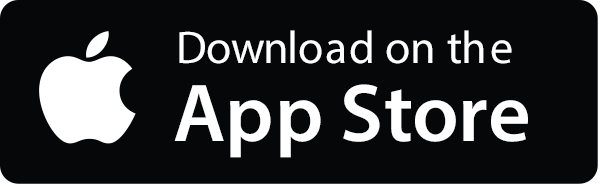
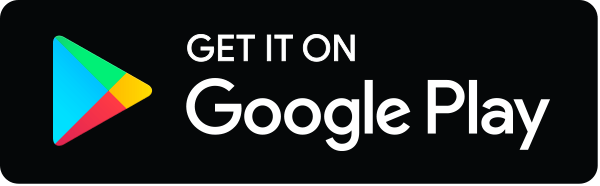