Christopher L. Norkus Allegheny Veterinary Emergency Trauma and Specialty (AVETS) Center, Monroeville, Pennsylvania, USA Consciousness can be defined as explicit awareness. Awareness implies that the brain is in an aroused state and that the patient has perceptual qualities of an experience. Within the central nervous system, the brain stem, pons, thalamic nuclei, and cortex with their connecting neural pathways are the anatomic sites that contribute to the state of consciousness (Fig. 10.1). The American Society of Anesthesiologists (ASA) currently defines general anesthesia as “a drug‐induced loss of consciousness during which patients are not arousable, even by painful stimulation” [1]. In effect, general anesthesia is thought to be a medically induced, reversible loss of consciousness. The traditional goals of successful general anesthesia include reversible unconsciousness, amnesia, analgesia, skeletal muscle relaxation resulting in immobility, and autonomic stability to maintain physiologic homeostasis. These goals are correlated with distinct sites within the central nervous system (CNS). For example, amnesia is associated with the limbic system, unconsciousness is associated with the interaction between the cerebral cortex and the thalamus, and immobility and analgesia are associated with the spinal cord [2]. Anesthetic depth is defined as the drug‐induced probability of nonresponse to stimulation, calibrated against the strength of the stimulus and the difficulty of suppressing the response. Clinically, anesthetic depth is seen as the degree to which the CNS, predominantly the cortex, is depressed by a general anesthetic agent. Broadly speaking, this depends on the concentration of anesthetic agents within the brain, the magnitude of environmental stimulation (i.e., degree of surgical stimulus), and concurrent CNS depressant effects such as hypothermia, hypercarbia, or hypotension. One of the current challenges in modern medicine is monitoring the depth of general anesthesia. This, along with measuring unconsciousness and recognizing awareness, is especially challenging in nonverbal veterinary species. Accurate assessment of the depth of anesthesia contributes to tailoring drug administration to the individual patient. Patient underdosing resulting in inadequate general anesthesia depth may lead to arousal or movement of the patient, which could be dangerous or even deadly to veterinary staff (e.g., horses kicking and large carnivores biting), exaggerated autonomic responses (e.g., arrhythmias, tachycardia, and hypertension), and intraoperative awareness with recall. Presently in humans, the incidence of perianesthetic awareness is approximately 0.1%, with this number increasing in cardiac surgery, cesarean section, and high‐risk patients [3–7]. The incidence of perianesthetic awareness in veterinary species remains unknown. Patient overdosing resulting in excessive general anesthetic depth may result in prolonged patient recovery, increased postanesthetic complications, and patient death. Effect‐site concentration is the concentration of a drug at the site of its biological activity; for example, the brain with general anesthetics. Effect‐site concentration is proportional to the pharmacological effect, whereas measurement of solely plasma concentration may not be. Unfortunately, rapidly measuring effect‐site concentration within the clinical anesthesia setting is not feasible at this time. In 1965, Eger introduced the concept of minimum alveolar concentration (MAC), and it has since been used as the standard measure of potency for volatile anesthetic agents. It is defined as the concentration of inhaled anesthetic within the alveoli at which 50% of subjects do not move in response to a noxious stimulus. In humans, this noxious stimulus is considered a surgical incision; however, in animals, this is often a noxious but non‐surgical stimulus such as tail clamp. MAC creates a unifying principle for inhaled anesthetics as inhaled concentration can be associated with a given response and is reproducible across various anesthetic agents. For example, administration of 0.25 MAC (MAC‐amnesia) will produce amnesia in humans, whereas 0.3–0.5 MAC (MAC‐awake) of a volatile anesthetic agent is needed to produce unconsciousness or return to awakening [8–10]. The end‐tidal concentration of inhaled anesthetic agents at which 50% of patients can be intubated smoothly is known as “MAC for endotracheal intubation” (MAC‐EI). Figure 10.1 Key regions in the central nervous system that contribute to a patient’s state of consciousness. Source: Dr. Christopher Norkus with illustration by Kyna Kozak, with permission. Administration of 1 MAC (MAC) of a volatile anesthetic prevents movement in response to a surgical or otherwise noxious stimulus in 50% of subjects. Considering normal distribution, MAC represents only a single point on the dose‐response curve and can be seen as equivalent to a median effective dose (ED50). Therefore, an individual patient may require more or less inhalant anesthetic than MAC. Assuming a standard deviation of MAC to be 10% and following the properties of a normal distribution, immobility would be expected to be achieved in 68% of patients at 1.1 MAC, 95% of patients at 1.2 MAC, and 99.7% at 1.3 MAC [10]. Administration of 1.5 MAC (MAC‐BAR) of a volatile anesthetic blunts autonomic responses to surgical incision in 50% of human patients. The autonomic responses commonly used to define MAC‐BAR include changes in pupil dilation, heart rate, and blood pressure [9]. Clinically, these multiples of MAC may be useful to roughly gauge anesthetic depth and titrate anesthetics. For example, the use of MAC may result in an inadequate or light plane of general anesthesia, while 1.5 MAC would represent a deep plane of general anesthesia for many patients. There are, however, numerous limitations to relying solely on this approach. Numerous factors can alter MAC. MAC can be increased in the face of hyperthermia, hyperthyroidism, young age, and concurrent medications that raise circulating central catecholamines (e.g., ephedrine, monoamine oxidase inhibitors, and amphetamine intoxication). MAC can be reduced by factors such as hypothermia, severe hypotension, advanced age, concurrent use of other CNS depressants (e.g., opioids, dexmedetomidine, and ketamine), pregnancy, and severe hypoxemia. Additional pharmacogenetic factors such as red hair in humans have been shown to alter MAC as well as anesthetic recovery time [11,12]. MAC values vary widely between species, and it is quite possible that similar genotypic or phenotypic differences exist across different animal breeds and species. The degree of noxious stimulus in question may also be variable and, depending on the procedure, may extend beyond a single skin incision, which defines MAC. Predicting end‐tidal inhalant concentration may be impossible without the use of anesthetic gas monitoring equipment, which is often not available outside of veterinary specialty or academic practice settings. Lastly and most notably, volatile anesthetic concentrations are measured as expired end‐tidal concentrations, which may not accurately reflect what anesthetic concentration reaches the brain. Similarly, with the use of total intravenous anesthesia (TIVA), target‐controlled infusions (TCI) can be utilized to predict plasma concentrations. These approaches predict drug concentration and not necessarily drug effect. As a result, MAC, its multiples, and plasma concentrations should not be relied upon to be the sole determinant of anesthetic depth. Physician Dr. Arthur Guedel served in the United States Army during the First World War and was stationed in France where he was responsible for overseeing up to six hospitals at one time. As part of this oversight, he was tasked to train orderlies and nurses to administer diethyl ether to wounded soldiers returning from the battlefield. Guedel developed guidelines using a wall chart that could be used by anesthetists to gauge the depth of anesthesia (Fig. 10.2) [13]. While Guedel’s original observations were made in human patients anesthetized with diethyl ether, they were subsequently adapted for use with other hypnotic agents and ultimately applied for use in animals. Guedel described four progressive stages of anesthesia beginning at its initial administration and ending at near death. Within stage 3 (surgical anesthesia), sub‐classifications of light, medium, and deep anesthesia planes were described. These planes of anesthesia represented the progressive CNS depression that could be observed while a patient was within a surgical depth of anesthesia. Modern balanced anesthetic and multimodal analgesia techniques utilize numerous agents, which may confound Guedel’s classification system, originally developed for use with the single agent diethyl ether. For example, the use of anticholinergics might result in alterations to patient heart rate, while the use of a dissociative agent such as ketamine might impact eyeball position, ocular reflexes, and general muscle tone. This has necessitated a greater reliance on additional patient monitoring parameters, including blood pressure, respiration, and neuromuscular tone, and created a continued interest in depth‐of‐anesthesia devices based upon electroencephalographic monitoring of CNS activity. Nevertheless, despite the incorporation of many new monitoring modalities in practice, the veterinary anesthetist should continue to have a strong understanding of the correlation of changing physical signs and their relationship to anesthetic depth progression. Stage 1 is considered the stage of voluntary movement and is defined as lasting from initial drug administration to loss of patient consciousness. Some analgesia may be present in the deeper phases of this stage. Excited, apprehensive animals may struggle. Respirations may become irregular, or increase, and the patient may voluntarily hold their breath for brief periods. A catecholamine release results in a strong, rapid heartbeat, increased patient blood pressure, and pupillary dilation. Cardiac arrhythmias may be observed during this stage. Salivation is frequent in some species, and urination or defecation may occasionally occur. Muscle tone remains and patients retain a normal eye position and palpebral and corneal reflex. Patients during this phase retain a pedal reflex and react strongly to surgical manipulation. With the approach of stage 2, animals become progressively ataxic, lose their ability to stand or right themselves, and assume lateral recumbency. Stage 2 is considered the stage of delirium or involuntary movement. It is commonly clinically referred to as the “excitement phase.” By definition, this stage lasts from loss of consciousness to the onset of a regular pattern of breathing. Patients may exhibit breath‐holding, tachypnea, and hyperventilation. They may retain muscle tone. Continued catecholamine release causes an increased heart rate and increased blood pressure. Arrhythmias may occur and the patient’s pupils remain dilated. Palpebral and corneal reflexes remain brisk. Nystagmus commonly occurs in horses. During this stage, animals may briefly vocalize. In some species, especially ruminants and cats, salivation may occur. In some species, including dogs, cats, and goats, vomiting may be seen. The larynx of cats and pigs is very sensitive at this stage, and premature stimulation with an endotracheal tube may easily result in laryngospasm. Patients during this phase retain a pedal reflex and react strongly to surgical manipulation. Figure 10.2 Characteristics of the stages of general anesthesia. Source: Dr. Christopher Norkus with illustration by Kyna Kozak, with permission. Stage 3 is considered the stage of surgical anesthesia. It is characterized by unconsciousness with progressive depression of reflexes. This stage is the targeted anesthetic level for procedures requiring general anesthesia. Muscle relaxation develops and ventilation becomes slow and regular. Vomiting and swallowing reflexes are lost. In humans, this stage has been further divided into planes I–IV for finer differentiation. Others have suggested the simpler classification of light (plane I), medium (plane II), and deep (plane III) planes are more appropriate. Light anesthesia persists until eyeball movement ceases. Medium anesthesia is characterized by progressive intercostal paralysis, and deep anesthesia by diaphragmatic respiration. A medium depth of anesthesia has traditionally been considered a light to medium plane of surgical anesthesia (stage 3, plane II), characterized by stable respiration and pulse rate, abolished laryngeal reflexes, an absent palpebral reflex, a strong corneal reflex, and adequate muscle relaxation with absent response to surgical stimulation. Deep surgical anesthesia (stage 3, plane III) is characterized by decreased intercostal muscle function and tidal volume, increased respiration rate, profound muscle relaxation, diaphragmatic breathing, a weak corneal reflex, and a centered and dilated pupil. Patients in stage 3 have their heart rates return to normal ranges and may experience progressive bradycardia. Blood pressure normalizes and begins to decrease as anesthetic depth is deepened. The frequency of arrhythmias decreases. Patients in light planes of anesthesia may react to surgical manipulation and have a pedal reflex, while these traits are lost in medium‐to‐deeper planes. In general, patients will progressively lose jaw tone and limb muscle tone, although this can vary depending on species and patient age. For example, young dogs may have minimal jaw tone even at light anesthesia planes, while other species such as ruminants and swine may maintain a high degree of jaw tone even at deep planes. Eyeball position will also vary depending on the species. In dogs and cats, for example, the globe will rotate to a ventromedial orientation. In other species such as horses, however, the globe will remain centrally oriented. In ruminants, the globe may rotate ventrally in light and medium anesthesia planes showing only the sclera and then returning centrally during deep anesthesia planes. Stage 4 is that of extreme CNS depression and overdose. In this phase, cardiopulmonary function fails. The patient may exhibit marked hypotension, weak pulses, bradycardia or transient tachycardia, prolonged capillary refill time, and other cardiac arrhythmias. Patients will hypoventilate and respiration itself may cease or become irregular. Pupils will acutely dilate. All reflexes are blunted, and muscle tone is absent. The corneal reflex ceases. In dogs and cats, the eyeball position will rotate to a central position. In this stage, death quickly occurs unless immediate resuscitative steps are taken, including lessening the patient’s anesthesia depth. Electroencephalography is the recording of spontaneous electrical brain activity from scalp electrodes [14–16]. Traditionally, these electrodes are placed at standard positions according to the International 10–20 system [14,16,17]. The electrical signals obtained from the surface of the scalp represent the summation of neuronal activity within the cerebral cortex, and result from excitatory and inhibitory postsynaptic potentials (PSPs) in large pyramidal neurons located in the lower layers, such as layer V, of the cerebral cortex [14,18–20]. The EEG amplitude and frequency are influenced by afferent inputs from sensory‐specific thalamic nuclei serving as gates between peripheral receptors and the cortex, and by epicenters within the cerebral cortex [21]. In 1934, Hans Berger proposed that the appearance of α waves in the EEG may represent cerebrocortical events associated with consciousness in the awake man [22]. It is believed that EEG activity associated with consciousness is generated from pacemaker neurons within the ascending reticular activating system (ARAS), and then mediated and modulated through thalamic connections [19,21,23–25]. These pacemaker neurons oscillate in the frequency range of 8–12 Hz and synchronize the excitability of cells in the thalamocortical pathways. Small cerebrocortical areas appear to also act as epicenters from which α activity spreads through cortical neuronal networks, generating the α rhythm that dominates the resting EEG. In the awake state, consciousness is maintained by a circulating activity among the ARAS, intralaminar nuclei, and the cerebral cortex [21]. Additional sensory stimulation will cause cortical arousal that is characterized by the desynchronization of α oscillators with the appearance of a faster rhythm in the β frequency range (12–25 Hz) [21,25–28]. The term “desynchronization” is characterized by a shift in the EEG pattern from high‐voltage, slow‐wave activity to low‐voltage, fast‐wave activity [19,23,29]. As early as 1937, Gibbs and colleagues reported that anesthetic agents altered electroencephalogram (EEG) activity from low‐voltage fast waves to high‐voltage slow waves and proposed that the EEG could be used clinically to measure the effects of anesthesia [30]. In 1959, Martin and colleagues first described hypnotic general anesthetics as producing a biphasic EEG effect [31]. They observed that during light anesthesia, there was EEG desynchronization, which changed to progressive synchronization, slowing, and increased amplitude as anesthesia deepened (Fig. 10.3A–C). With further increases in anesthetic depth, the EEG displayed burst suppression and lengthening periods of EEG silence (Fig. 10.3D). Furthermore, a topographic distributional change from occipital lobe dominance to frontal lobe dominance was observed. More specifically, the EEG frequency bands utilized for assessment of anesthetic depth are the δ (0–4 Hz), θ (4–8 Hz), α (8–13 Hz), and β (13–30 Hz) bands [32,33]. During induction and maintenance of anesthesia, there is a progressive decrease in EEG frequency content characterized by a shift from a low‐voltage, fast‐wave pattern to a high‐voltage, slow‐wave pattern [34–37]. As anesthetic depth increases, the predominant EEG pattern is characterized by a decrease in β activity and a concomitant increase in both α and δ activity [38]. As anesthesia deepens further, EEG activity in the θ and δ frequency bands appears initially in centroposterior regions with subsequent anterior spread [39]. Lastly, very deep levels of general anesthesia are first indicated by flat periods interspersed with periods of α and β activity, known as a “burst suppression pattern,” followed by a complete loss of electrical discharge known as “isoelectricity” or “electrical silence” [35,36,40]. Upon reducing the dose of the anesthetic agent, these patterns reverse, and lighter levels of anesthesia are indicated again by a decrease in amplitude and an increase in frequency content. Figure 10.3 During induction and maintenance of anesthesia, there is a progressive decrease in EEG frequency content slowing, which will be characterized by a shift from a low‐voltage, fast‐wave pattern to a high‐voltage, slow‐wave pattern (A–C). Deep levels of general anesthesia are indicated by flat periods interspersed with a burst suppression pattern, followed by a complete loss of electrical discharge known as isoelectricity or electrical silence (D). Source: Dr. Christopher Norkus with illustration by Kyna Kozak, with permission.
10
Anesthetic Depth Monitoring and Electroencephalography
Introduction
Minimum alveolar concentration
Physical signs of anesthetic depth
Stage 1
Stage 2
Stage 3
Stage 4
Electrophysiologic monitoring
Depth‐of‐anesthesia devices
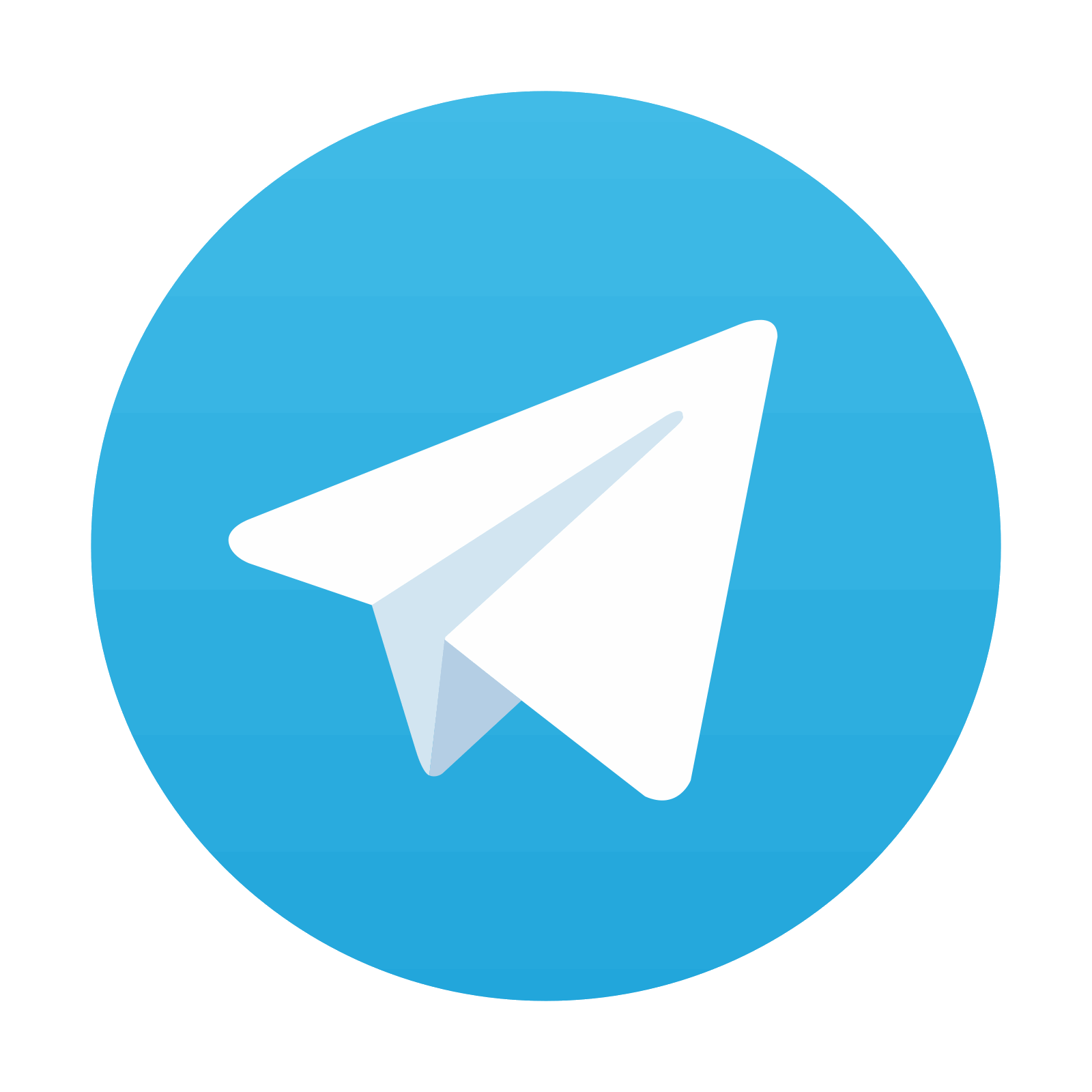
Stay updated, free articles. Join our Telegram channel
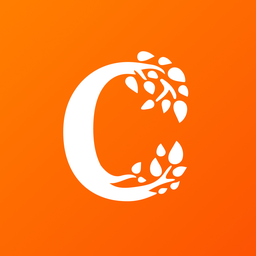
Full access? Get Clinical Tree
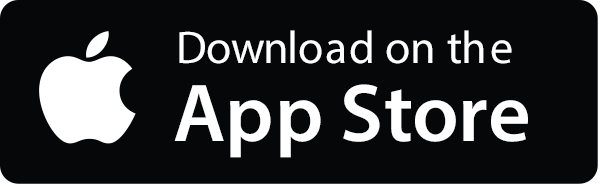
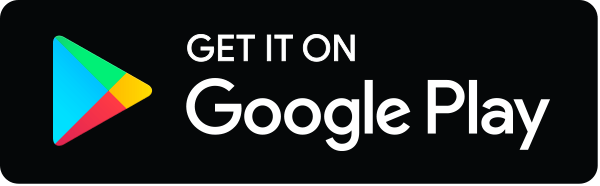