Daniel S.J. Pang Faculty of Veterinary Medicine, University of Calgary, Calgary, Alberta, Canada and Faculty of Veterinary Medicine, Université de Montréal, Saint‐Hyacinthe, Québec, Canada Adjunctive drugs can be broadly described as drugs that fall outside common clinical use but may provide a benefit beyond that of more commonly used drugs or are less readily characterized because they play a supportive role in the provision of anesthesia and analgesia. Some of the drugs described in this chapter may enter the mainstream as evidence supporting their use grows, while others may remain unconventional, restricted to second‐ or third‐line therapy, or fall out of use as alternatives are identified or emerging evidence fails to support their use. This chapter is divided into non‐analgesic and analgesic adjuncts. Dantrolene is a peripherally acting muscle relaxant, producing skeletal muscle relaxation through ryanodine receptor antagonism and inhibition of the dihydropyridine receptor calcium channel [1–3]. There are three isoforms of ryanodine receptors with RyR1 believed to be predominant in skeletal muscle. Receptors are located in organelle membranes (i.e., mitochondrial, endoplasmic and sarcoplasmic reticular, and nuclear). The primary effect of dantrolene is to reduce calcium release from the sarcoplasmic reticulum into the cytoplasm via the RyR1 receptor (a calcium channel), thereby dissociating excitation–contraction coupling. Its muscle‐relaxing properties are limited to skeletal muscle though cardiac depression has been reported in dogs when administered at high doses (greater than 5 mg/kg) or in combination with verapamil [4]. At therapeutic intravenous (IV) doses (1–5 mg/kg) in dogs and cats, minute ventilation during general anesthesia is reduced through depression of both respiratory rate and tidal volume [5,6]. In humans, adverse effects are described as minor and include phlebitis, transient muscle weakness, and gastrointestinal upset [7]. Dantrolene is primarily indicated for the treatment and prevention of the pharmacogenetic disorder malignant hyperthermia (MH) [8–14], and rhabdomyolysis [15–19], with a potential role in cytoprotection and as an antiarrhythmic [2,3,20]. Shortly after MH was first reported in humans [21,22] and pigs [23,24], the successful use of dantrolene for the prevention and treatment of MH was published with the experiments performed in pigs [9]. It has since been used to treat MH in dogs [10] and horses [11]. Dantrolene is used in the prevention and treatment of exertional rhabdomyolysis in horses [16,18,25], though use in rhabdomyolysis in a dog [15] and tetanus in cats [19] has also been reported. In horses given dantrolene before general anesthesia, there is a risk of hyperkalemia [26]. A study of six adult horses given oral dantrolene (6 mg/kg) or water 1 h before induction of general anesthesia (crossover study design) found hyperkalemia (serum potassium > 5.5 mEq/L) occurred in four horses, with two of these horses having serum potassium concentrations > 7 mEq/L [26]. Three horses developed bradyarrhythmias associated with hyperkalemia. Dantrolene administration was also associated with reduced cardiac output (approximately 25–30%), heart rate, and arterial blood pressure (approximately 20%). No difference in recovery time was observed. Dantrolene undergoes hepatic metabolism, primarily hydroxylation, in the dog and horse. Metabolites and up to 1% of unchanged dantrolene are excreted in urine and bile [27–29]. Dosages in horses are as follows: 1.9 mg/kg IV loading dose followed by oral dosing at 2.5 mg/kg every 60 min [28], or 2–4 mg/kg PO [11,16,18,26,29]. Notably, there is considerable interindividual variation in plasma concentrations following oral administration [16,26]. Dosages in dogs and cats are as follows: 1–3 mg/kg IV (low end of dose range for muscle relaxation and high end for the treatment of MH); 5–6 mg/kg PO once daily as prophylaxis. As seen with horses, oral dosing in dogs is associated with wide variability in plasma concentrations of dantrolene [30]. Dosages in pigs are as follows: 1–3 mg/kg IV for the treatment of MH; 5 mg/kg PO for prophylaxis. Dantrolene is available as a powder for reconstitution (with sterile water). The two commercial formulations available are reconstituted to 0.33 mg/mL (Dantrium®) and 50 mg/mL (Ryanodex®). Solutions of reconstituted dantrolene are light sensitive and stable for 6 h [3]. Dantrolene is also available as 100 mg capsules. Diphenhydramine has a long history of perioperative use, particularly in dogs and cats undergoing mast cell tumor resection or exhibiting suspected allergic and anaphylactic reactions. It is an antihistamine, acting as a reversible competitive antagonist at the H1 receptor [31,32]. Histamine receptors, of which there are at least four (H1, H2, H3, and H4), are widely distributed in the body, with H1 present in the brain, airway smooth muscle, skin, gastrointestinal and genitourinary tracts, adrenal medulla, immune system, heart, and vascular endothelium (reviewed by Peters and Kovacic) [32]. Agonist activity at the H1 receptor leads to phospholipase C activation and increased intracellular calcium, which, in turn, promotes airway smooth muscle contraction and catecholamine release from the adrenal medulla. In the vascular endothelium, H1 receptor activation leads to nitric oxide formation and vasodilation. Additionally, increased intracellular calcium triggers a host of secondary signaling pathways, leading to vasodilation and increased cellular permeability. Centrally, diphenhydramine’s effect on histamine receptors in the brain is to cause drowsiness in people [31]. While this effect is often proposed in animals, there is little supporting evidence [33–35]. In a randomized, blinded, placebo‐controlled clinical trial in dogs, diphenhydramine doses of 2–8 mg/kg intramuscular (IM) were not associated with sedation (observed for 30 min after administration), with sedation scores no different than the saline control group [33]. At the highest dose (8 mg/kg), there was a small increase in sedation score compared to baseline, but this was not statistically significant when compared with other groups. As an older drug, it is less selective than newer agents. It may be that higher doses, in the region of 10 mg/kg PO, are required to cause sedation in dogs [33]. In addition to its effects on the H1 receptor, it also has antimuscarinic and antidopaminergic properties, which are likely to contribute to the adverse effects associated with high doses in dogs [31,36]. At doses of 30–45 mg/kg PO in dogs, diphenhydramine resulted in mydriasis, muscle tremors, increased muscle tone, head swaying, and hyperreactivity [36]. It is primarily used in humans for the management of motion sickness, as well as postoperative and opioid‐induced nausea and vomiting [37]. In cats, a dose of 3 mg/kg PO resulted in behavioral changes within 30 min, reaching a peak effect at 1–2 h and lasting for over 4 h [38]. These effects included altered locomotion, increased muscle tone, tremors, hypersalivation, and vomiting. Effects on sleep were varied but included increased wakefulness and disrupted sleep patterns. The benefits of diphenhydramine administration before mast cell tumor excision and in suspected anaphylaxis are unclear. Based on an earlier pharmacokinetic study showing plasma levels of diphenhydramine exceeding the therapeutic range in humans, Sanchez et al., in a randomized, blinded, placebo‐controlled trial, found no impact of diphenhydramine (1 mg/kg IV) on plasma histamine concentrations in dogs (n = 8) undergoing mast cell tumor resection (three dogs had grade 1 tumors, two had grade 2, two had grade 3, and one had grade 4) [39]. Intraoperatively, mean and diastolic arterial blood pressures were approximately 10–15 mmHg lower in the diphenhydramine group compared to control, with a small increase in cardiac index [39]. An in vitro study of a canine mast cell line found that diphenhydramine did not prevent histamine release [40]. Current treatment guidelines for anaphylaxis emphasize epinephrine for first‐line therapy, with antihistamines described as having a “limited role” as a second‐ or third‐line therapy, useful in managing cutaneous symptoms, if present [41,42]. In cutaneous allergic reactions in dogs, there is little evidence supporting the use of diphenhydramine [35]. The pharmacokinetics of diphenhydramine have been described in rabbits, camels, horses, sheep, and dogs [34,43–46]. In conscious dogs administered 1 mg/kg IV or 2 mg/kg IM, terminal half‐life was reported to be approximately 4 and 7 h, respectively, with 88% bioavailability from IM injection [34]. No adverse effects were reported at these doses and with these routes of injection (the IV dose was given as a bolus over < 5 s) and resultant plasma levels were in the therapeutic range reported in humans. Oral diphenhydramine bioavailability in dogs is low (3–7%) [47]. Doses in dogs and cats are 1–4 mg/kg (every 8 h, PO, IM, or IV) and 0.5–1 mg/kg (every 12 h, PO or IM), respectively. In dogs, IM injection is preferred over IV use, with a described risk of hypotension/cardiovascular instability associated with the latter route [34]. The drug is supplied as a 50 mg/mL injectable formulation. The analeptic (central nervous system [CNS] stimulant) agent doxapram has a long history of use in veterinary medicine and has been applied in a wide variety of situations including arousal from sedation/anesthesia [48–50], cardiopulmonary resuscitation (CPR) [51], stimulation of respiration in neonates and following drug‐induced respiratory depression [52–60], and assessment of laryngeal function [61–63]. The mechanism of action leading to respiratory stimulation is likely a combination of central and peripheral effects [64–66]. Electrophysiological studies in cats and dogs showed increased activity of the respiratory nuclei of the medulla when given therapeutic doses of doxapram [64]. As the dose was increased, cortical stimulation occurred followed by convulsions. A safety margin of 20–60:1 was cited before onset of central stimulation and convulsions. Experimental evidence suggests that stimulation of respiration is dose dependent, with activation of peripheral aortic and carotid receptors occurring at doses (0.05–0.25 mg/kg IV) below those typically used clinically [65]. As the dose is increased (0.5–5.0 mg/kg IV), stimulation of respiratory and non‐respiratory brainstem neurons occurs [65]. There is limited evidence for interspecies variability in the doxapram dose effective for respiratory stimulation versus CNS arousal. Low doses of doxapram are successful in eliciting respiratory stimulation in cats before cardiovascular or CNS stimulation occurs [64–66], but higher doses may be necessary in other species. It is unclear to what extent this dose dependency occurs in dogs and horses, with evidence of CNS arousal occurring at doses effective for respiratory stimulation [52,67–69]. The molecular mechanisms underlying peripheral and central control of respiration remain an active area of research. Recent evidence suggests involvement of two‐pore domain potassium channels, and these channels may also be involved in the mechanism of inhalational anesthesia [70–72]. See [73] for a review. Doxapram has the ability to significantly increase minute ventilation through an increase in both respiratory rate and tidal volume, leading to the term “pharmacologic ventilator” [74]. However, its effects are relatively short‐lived, reflecting a rapid decline in plasma levels following an IV bolus [75]. Doxapram undergoes hepatic metabolism in dogs with renal excretion of metabolites. A similar pharmacokinetic profile is observed in horses with a rapid decline in plasma levels following IV bolus administration, extensive hepatic metabolism, and renal excretion of metabolites [76]. Doxapram also results in modest increases in arterial blood pressure and heart rate in humans [74]. While cardiovascular changes are short‐lived in healthy animals (i.e., several minutes), one report of its use in dogs (1.5 mg/kg IV) rendered hypovolemic from controlled hemorrhage showed sustained increases in cardiac output and arterial blood pressure for up to 1 h [69]. Doxapram does not induce cardiac arrhythmias in dogs anesthetized with halothane or cycloproprane in the presence of epinephrine and hypercapnia and does not cause meaningful changes in hematology or serum biochemistry [77–78]. In healthy adult horses and those with experimentally induced respiratory disease, a dose of 0.3 mg/kg IV resulted in an approximately 50% increase in respiratory rate and tidal volume [79]. The duration of action was not described. During halothane anesthesia, preceded with acepromazine and thiopental in ponies, the addition of doxapram (0.05 mg/kg/min IV) resulted in a return of arterial carbon dioxide tension (PaCO2) and pH to near normal levels, with an increase in respiratory rate (tidal volume was not measured) [67]. An increase in mean arterial blood pressure of approximately 20% without an accompanying increase in heart rate was observed during doxapram infusion. Interestingly, the infusion of doxapram was associated with a reduction in plane of anesthesia, necessitating an increase in halothane vaporizer setting. In cats anesthetized with a preparation of alfaxalone–alfadolone (Saffan®), administration of doxapram (7.5 mg/kg IV) resulted in a 181% increase in minute ventilation, which lasted for several minutes [53]. A similar pattern was seen in cats anesthetized with thiopental [53]. At this dose, heart rates increased for several minutes during both thiopental and Saffan® anesthesia to approximately 15–20% above the pre‐doxapram period. Recovery was faster in the Saffan® group with the addition of doxapram, while no change was observed in the thiopental group. The use of doxapram in patients with a history of seizures is controversial [73]. While it is clear that cortical activity increases in a dose‐dependent fashion, it is unclear if this constitutes proconvulsant activity. Care should be exercised when administering doxapram in patients with a history of seizures. As always, the use of appropriate dosing (i.e., to effect) is critical. Two areas of active doxapram usage are in foal and calf neonatal medicine and assessment of laryngeal function in dogs. Retrospective data from a cohort of foals with hypoxic‐ischemic encephalopathy (neonatal maladjustment syndrome) revealed doxapram (0.02–0.05 mg/kg/min IV) to be more effective than caffeine (loading dose of 7.5–12 mg/kg followed by 2.5–5 mg/kg/day PO via nasogastric tube) at reducing PaCO2 for at least 12 h [55]. However, foals remained acidemic with no significant increase in pH. The reduction in PaCO2 was achieved with an increase in tidal volume, without an increase in respiratory rate. No effects on heart rate were observed, and there was no difference in mortality rates. These data agreed with an earlier retrospective study in healthy foals showing no advantage of caffeine over saline in correcting respiratory acidosis created with isoflurane anesthesia [54]. Furthermore, a doxapram dosing regimen of 0.5 mg/kg followed by 0.03 mg/kg/min IV was as effective as 0.5 mg/kg followed by 0.08 mg/kg/min. Giguère et al. also reported an improvement in arterial oxygen tension (PaO2) following doxapram administration, compared with saline control or caffeine [54]. The effects of doxapram in healthy foals differed from those observed in healthy adult horses, with a small but significant increase in heart rate observed in healthy foals regardless of doxapram dose. Doxapram also resulted in a modest increase in mean arterial blood pressure of approximately 10 mmHg. The mechanism of increased ventilation also differed; minute ventilation was increased in healthy foals as a result of increased respiratory rate and not tidal volume. In calves, a comparison of doxapram (2 mg/kg IV) and theophylline (7 mg/kg IV) showed a marked increase in respiratory rate with doxapram but not theophylline [56]. Respiratory rate doubled 10 min after doxapram administration followed by a steady decline over the next 2 h (end of the recording period). The effect on PaCO2 (maximal decrease of approximate 20 mmHg) and PaO2 (maximal increase of approximate 15 mmHg) peaked 30 s following administration, followed by a steady return toward baseline over the recording period. Small increases in heart rate (120–155 beats/min) and systemic systolic arterial blood pressure (105–112 mmHg) were also observed without a significant change in cardiac output. A greater change (20 mmHg increase) was observed in systolic pulmonary arterial blood pressures. The authors concluded that the transient respiratory stimulation following doxapram administration could be beneficial in newborn calves when positive pressure ventilation was not possible. Similar results were observed in neonatal calves (2–15 h old) given doxapram at approximately 1 mg/kg IV, which resulted in a doubling of minute ventilation, an approximately 20% increase in PaO2, and a 25% decrease in PaCO2 [57]. Interestingly, a systematic review of doxapram use in human preterm infants did not identify a clear advantage associated with doxapram use over saline [80], and there is evidence that doxapram may cause cortical damage due to a reduction in cerebral blood flow [81–83]. Laryngeal paralysis in dogs manifests as a paradoxical movement of the arytenoid cartilages in affected dogs, with inward collapse of the arytenoid cartilages as subatmospheric thoracic pressure is generated during inspiration. While there are numerous studies evaluating the effect of doxapram on arytenoid cartilage motion in dogs, there appears only one in which both healthy and diseased (laryngeal paralysis) dogs were included [61]. In that study, following premedication with acepromazine (0.022 mg/kg IM in diseased dogs and 0.2 mg/kg IM in healthy dogs) and butorphanol (0.44 mg/kg IM), animals were preoxygenated for 5 min and general anesthesia induced with isoflurane delivered by mask. Doxapram was administered at 1.1 mg/kg IV following induction of general anesthesia. At baseline, the normalized glottal gap area was significantly larger in dogs with laryngeal paralysis during both inspiration and expiration. Following doxapram administration, the normalized glottal gap area at inspiration was significantly reduced in dogs with laryngeal paralysis compared to normal dogs, and the area during expiration was significantly greater in dogs with laryngeal paralysis. The dramatic changes observed (i.e., marked arytenoid adduction during inspiration) allowed for diagnosis but also led the authors to warn of the need to potentially intubate the airway to provide oxygen. Studies in healthy dogs consistently report increased laryngeal motion following doxapram administration (0.5–2.5 mg/kg IV) [63]. In horses, laryngeal function has been evaluated by comparing the following techniques: animals standing at rest with a twitch, at rest without twitch, manual occlusion of nostrils, post‐exercise (lunging at canter), swallowing, doxapram (40 mg/kg IV), and xylazine (0.5 mg/kg IV) [62]. Subjectively, doxapram resulted in an increased respiratory rate in approximately half of the horses examined (total n = 7), but the most successful technique for stimulating deep breaths was manual nostril occlusion. Using doxapram during CPR is no longer recommended, though this practice may persist. An internet survey of 600 veterinarians in small animal practice, with or without board certification in critical care and emergency medicine or anesthesia, showed that while doxapram was as frequently available to both specialists and practitioners (74% and 81%, respectively), a significantly higher percentage of general practitioners had used it during CPR (29% and 70%, respectively) [51]. This contrasts starkly with the current evidence‐based CPR guidelines in which doxapram is not mentioned [84]. The use of doxapram at 0.1–0.2 mg/kg IV has also been suggested to facilitate recovery from general anesthesia in horses, when appropriate, by increasing the level of arousal and stimulating respiration, thereby speeding reduction in alveolar concentrations of inhalational agents [85]. Other species in which doxapram use has been recorded are cattle (1 mg/kg IV was effective in speeding recovery from xylazine), camels (0.05–0.13 mg/kg IV was effective at speeding recovery from xylazine in Bactrian camels), llamas (2.2 mg/kg IV was not effective in reversing xylazine), and southern elephant seals (appeared to cause CNS stimulation at 5 mg/kg when used to aid recovery from a range of drug combinations; therefore, authors recommended 2 mg/kg) [86–89]. Acid secretion in the stomach is initiated at the level of parietal cells by gastrin, histamine type‐2 (H2), and muscarinic (M3) receptors. Receptor activation results in increased proton pump activity on the luminal side of the cell via calcium‐ or cAMP‐dependent pathways. Several H2 receptor antagonists (famotidine, ranitidine, and cimetidine) have been used clinically. Famotidine and ranitidine are 20–50 and 4–10 times more potent than cimetidine, respectively. Perioperative use is usually to offset risk of gastrointestinal ulceration, with common indications including stress‐ or non‐steroidal anti‐inflammatory drug (NSAID)‐related abomasal and gastric ulcers in calves, foals, and horses, and following regurgitation during anesthesia in dogs. In a crossover study of five healthy horses, a comparison of famotidine and ranitidine found a high degree of individual variation in measured gastric fluid pH following administration of either drug at a range of dosages [90]. Each horse received famotidine (0.5, 1, and 2 mg/kg) and raniditine (4.4 and 6.6 mg/kg) via nasogastric tube. For famotidine, gastric fluid pH increased above 6 in three of five horses receiving 0.5 mg/kg, five of five horses receiving 1 mg/kg, and three of five horses receiving 2 mg/kg. For ranitidine, gastric fluid pH increased above 6 in four of five horses receiving 4.4 mg/kg and five of five horses receiving 6.6 mg/kg. Ranitidine demonstrated a tendency to result in a longer elevation of gastric fluid pH (166 ± 106 min versus 98 ± 110 min; mean ± SD). The drug response varied considerably between horses, leading the authors to suggest a dose of 6.6 mg/kg ranitidine. Similar studies in 2–6‐day‐old foals and milk‐fed calves resulted in recommended dosages of ranitidine of 6.6 mg/kg PO three times daily and 50 mg/kg PO three times daily, respectively [91–92]. However, more recent evidence highlights the risk of extrapolating the need for H2 antagonists in critically ill foals without measurement of gastric pH [93]. This study found that almost 50% of hospitalized foals had a baseline gastric pH, which was alkaline. Data supporting the use of famotidine in calves and foals are not available. Omeprazole and pantoprazole are proton pump inhibitors, which act on the proton pump located at the luminal surface of gastric parietal cells. Inhibition of acid secretion by proton pump inhibitors is cumulative in humans, requiring 2–4 days to achieve maximal inhibition [94]. This delay results from a combination of (1) increasing inhibition of parietal cells as dormant cells are activated following initial inhibition of active cells, (2) increased intestinal absorption of the drug as acid secretion is decreased in response to the drug’s effects, and potentially (3) the impact of cytochrome P450 inhibition to decrease the drug’s own metabolism. Proton pump inhibitors are metabolized by the cytochrome P450 system (CYP1A1/2 and CYP2D15 in dogs), and the pharmacokinetics of esomeprazole (the S‐enantiomer of omeprazole) have been reported in dogs [95]. The delay to achieve maximal effect can be minimized with initial IV administration or use of enteric‐coated formulations (though breaking or crushing coated tablets may reduce this benefit). Interestingly, the efficacy of proton pump inhibitors is increased with acid secretion (as occurs with feeding) and reduced when given in conjunction with H2 receptor antagonists [96]. Human clinical evidence suggests that omeprazole is more effective at raising gastric pH above 3 (a level correlated with healing gastric and duodenal ulcers in humans) compared to H2 receptor antagonists [97]. The dose in dogs and cats is 1–2.5 mg/kg PO once or twice daily [94,98,99], while the dose in horses and foals is 1–4 mg/kg PO once daily, with the low end of dose range for ulcer prevention and the higher end for treatment [100–102]. In 2‐day‐old calves, the pharmacokinetics of IV pantoprazole (1 mg/kg) have been studied and the terminal half‐life reported to be 2.8 h, which is longer than that reported in foals (1.5 h), adult alpacas (0.5 h), and adult goats (0.7 h) [103–106]. In foals, pantoprazole (1.5 mg/kg IV) was effective at increasing gastric pH for up to 24 h following a single dose [104]. In alpacas, pantoprazole (2 mg/kg SC or 1 mg/kg IV, daily for 3 days) was effective at increasing third compartment pH above baseline within 4 or 12 h of initial administration, respectively [105]. The perioperative use of famotidine in dogs and cats is commonly performed in cases with suspected gastrointestinal ulceration or in patients at risk of ulceration. Recommended doses of famotidine in dogs and cats vary widely due to limited clinical data. In dogs, 0.1–0.5 mg/kg twice daily or 1 mg/kg once daily (PO, IV, SC, IM) has been advocated. In cats, 0.2–0.25 mg/kg once or twice daily (IM, SC, PO, IV) has been suggested. Slow IV administration in cats is recommended based on anecdotal evidence that fast injection (i.e., less than 5 min) is associated with hemolysis from the benzyl alcohol component of the injectable formulation. However, a retrospective study where hospital policy was to administer famotidine over 5 min IV did not identify an effect on packed cell volume (PCV) [107]. The same study showed no change in PCV when cats received famotidine SC. There are limited clinical data demonstrating efficacy of famotidine in dogs, and omeprazole may be superior at raising gastric pH for a useful period of time [98,108]. A recent study in dogs with exercise‐induced gastritis and clinically relevant gastric ulcers showed famotidine (approximately 1 mg/kg PO once daily) to be superior to no treatment in preventing gastric ulcers but inferior to omeprazole (approximately 0.85 mg/kg PO once daily) [108]. In healthy adult dogs, famotidine (0.5 mg/kg IV twice daily) resulted in a significant elevation of gastric pH above saline control and ranitidine, but did not raise pH as high, or for as long, as omeprazole (1 mg/kg PO once daily). Increasing the dosing frequency of famotidine to three times daily did not result in any improvement [98]. The preoperative use of omeprazole or esomeprazole shows variable results depending on dose and route of administration [95,109–111]. In one study, the administration of esomeprazole (two 1 mg/kg IV doses 12 h apart before induction of general anesthesia) resulted in a less acidic reflux but did not reduce the incidence of reflux [109]. In contrast, another study reporting the use of preoperative omeprazole (single 1 mg/kg PO dose at least 4 h before induction of general anesthesia) showed a reduction in the number of gastroesophageal reflux and regurgitation episodes [110]. A direct comparison between findings is difficult due to differences in study design. Zacuto et al. also had another treatment group, esomeprazole (1 mg/kg IV) with cisapride (1 mg/kg IV), which resulted in less acidic reflux but also a significant reduction in the number of reflux events compared to the control and omeprazole groups [109]. Esomeprazole (1 mg/kg, IV, SC, and enteric‐coated formulation PO) was effective at increasing gastric pH in conscious dogs [95]. The duration that pH was ≥ 3 was longest with SC and PO administration, with an SC dose maintaining pH ≥ 3 for 80% of the 24‐h observation period (in contrast to approximately 60% and 40% for the first and second 12‐h periods after IV administration, respectively). Slightly greater efficacy (not statistically significant) and less variability were observed with once daily administration PO for 5 days. The pharmacokinetic profile of SC and PO administration is favorable in dogs, with bioavailability of approximately 100% and 70%, respectively [95]. Peak plasma concentrations were achieved between 1.8 and 0.8 h after PO administration on the first and fifth day, respectively. However, the terminal half‐life is approximately 1 h regardless of route of administration (PO, SC, IV), indicating that once daily dosing is insufficient, with twice daily dosing recommended in dogs and cats [94,95]. Similar pharmacodynamic data were reported in dogs given omeprazole (approximately 0.5–1 mg/kg of an enteric‐coated formulation PO, twice daily, for 5 days) in a randomized, blinded, placebo‐controlled crossover study, with gastric pH maintained ≥ 3 for 90% of the 4‐day observation period (days 2–5 of PO administration) [111]. Recently, a long‐acting omeprazole formulation was evaluated in dogs [112]. Following a single injection (4 mg/kg IM), gastric pH ≥ 3 was maintained for over 60% of a 7‐day observation period (peak effect on days 1–4). A study of the same formulation in horses (3.4–4.4 mg/kg IM) found it to be effective for up to 7 days after injection in four of seven horses studied [113]. There is currently no clear evidence supporting the use of one proton pump inhibitor over another in dogs or cats, but available evidence indicates that proton pump inhibitors are better than H2 receptor antagonists at increasing gastric pH [94,98,99,108]. It is important to recognize that, while the incidence of gastroesophageal reflux is relatively high in anesthetized patients (up to approximately 50%) [114,115], the incidence of complications such as esophageal stricture formation is extremely low [116]. There is a theoretical concern with chronic proton pump inhibitor therapy resulting in a change in gastric fauna and bacterial overgrowth, potentially increasing the risk of pneumonia in patients predisposed to regurgitation, such as those with megaesophagus. Another potential concern is the effect of omeprazole to inhibit the cytochrome P450 system, specifically CYP2C19, responsible for diazepam metabolism [117,118]. There is currently no evidence that this is of clinical significance in veterinary medicine, and the large therapeutic margin of diazepam may reduce the risk of adverse events. Famotidine is available as 10 mg tablets (other strengths may be available), an 8 mg/mL suspension, and a 10 mg/mL solution for injection. Omeprazole is available as 20 and 40 mg capsules and is licensed as an oral paste in horses (370 mg/g of paste). Esomeprazole is available as 20 mg capsules and as injectable formulations (21.3 and 42.5 mg/mL). Pantoprazole is available as tablets (20 and 40 mg) and an injectable formulation (4 mg/mL). Guaifenesin, originally derived from the Guaiacum genus of trees, is a centrally acting skeletal muscle relaxant with sedative properties primarily co‐administered IV with injectable anesthetic agents for the induction and maintenance of general anesthesia in horses and ruminants. Historically, it was used for this indication in humans along with a role in the management of tetanus. It has been in use in veterinary anesthesia since the 1960s [119,120]. In humans, it is currently used exclusively as an expectorant [121]. The mechanism(s) of action of guaifenesin are largely unknown, but evidence from the 1950s suggests sites of action in both the brain (brainstem and subcortical regions) and spinal cord (polysynaptic reflex inhibition) via interneuron depression, resulting in sedation and muscle relaxation [120,122]. There is extremely limited evidence to suggest it has any analgesic properties and none are typically attributed [120]. It has a wide therapeutic margin, with doses resulting in cardiopulmonary adverse effects such as hypotension, respiratory depression, and apneustic breathing pattern, being approximately 70–80% greater than that required to induce recumbency in horses (75–150 mg/kg) [119–123]. When given to induce recumbency in ponies and horses (dose ranges of 109–134 mg/kg), plasma levels of guaifenesin ranged from 238,000 to 313,000 ng/mL [124,125]. Cardiovascular effects are mild when guaifenesin is administered on its own at recommended doses, with no change in heart rate, cardiac output, central venous pressure, or respiratory rate, and an approximately 20% decrease in mean arterial blood pressure [124,126]. Similarly, respiratory effects are minimal, and the physiologic changes associated with recumbency appear to have a greater effect than any respiratory depression from guaifenesin [127]. Minute ventilation is maintained as a result of increased respiratory rate offsetting a decrease in tidal volume, indicating a sparing effect on the respiratory muscles [124]. Schatzmann et al. found minimal changes in PaO2 when horses were administered guaifenesin (100 mg/kg IV) but remained standing [127]. Overdose in horses results in a period of transient muscle spasm and mydriasis as the dose approaches 180 mg/kg IV, followed by loss of palpebral reflex, with death following respiratory or cardiac arrest at approximately 460 mg/kg [120]. Guaifenesin undergoes hepatic metabolism with glucuronidation followed by renal excretion. A sex difference in elimination has been identified in ponies with more rapid elimination in females [125]. The dose of guaifenesin required to achieve recumbency also shows a sex difference in ponies, with stallions requiring a higher dose than mares (130 versus 108 mg/kg IV) and a longer half‐life (84 versus 60 min) [124]. Plasma half‐life was approximately 75–80 min at doses of 88 and 134 mg/kg IV in adult horses, with no sex difference between geldings and mares, though geldings required a higher dose to achieve recumbency (163 versus 111 mg/kg IV) [124]. Guaifenesin is commonly prepared as a 5–15% solution with 0.9% saline or 5% dextrose solution, though it can also be solubilized in sterile water. There is a concentration‐dependent risk of hemolysis which appears unrelated to the speed of administration [119,127,128]. Hemolysis has been documented in horses when the concentration exceeds a 15% solution, and in cattle when it exceeds 5% [119,127,129]. Intravascular thrombus formation is also dose related, and concentrations of greater than 7% are associated with increased risk of thrombosis [127,130,131]. This effect is related to solution concentration rather than speed of administration, with reported histologic changes including endothelial loss with formation of a fibrin clot [130]. With a 10% solution the thrombus is not occlusive [130]. Guaifenesin is prone to precipitate out of solution when stored below room temperature (22 °C), but this is reversible with gentle heating such as immersion in a warm water bath [120] or gradual warming in a microwave. Perivascular injection has been associated with tissue necrosis [120], and administration via an IV catheter is recommended. Urticaria attributed to guaifenesin has also been reported [132]. In the reported case, the horse also received xylazine, ketamine, butorphanol, bupivacaine, and isoflurane, but the timing of the appearance of urticaria and failure of recurrence during two subsequent anesthetic episodes without guaifenesin implicates a role for guaifenesin. Plasma histamine levels were slightly elevated (5.2 ± 7.1 ng/mL) compared with baseline (3.2 ± 2.4 ng/mL) when guaifenesin was given IV in combination with xylazine and ketamine to adult horses (n = 14) [133]. The inclusion of guaifenesin (with xylazine and ketamine) as part of a partial intravenous anesthesia (PIVA) protocol administered to mares with dystocia was associated with 25 times greater odds of death compared with α2‐adrenergic receptor agonist and ketamine PIVA (9 times greater odds), and α2‐adrenergic receptor agonist PIVA [134]. The authors suggested that the inclusion of guaifenesin contributed to greater ataxia and sedation in recovery. In a study of the effect of numerous perianesthetic drugs (α2‐adrenergic receptor agonists, S‐ketamine, lidocaine, rocuronium, atipamezole, acepromazine, butorphanol, benzodiazepines, and guaifenesin) on the lithium sensor used in lithium dilution cardiac output measurement, guaifenesin had minimal effect on sensor performance (< 10% bias) [135]. Maropitant is a neurokinin‐1 (NK1) receptor antagonist licensed as an antiemetic in dogs and cats. It is highly protein‐bound and undergoes extensive hepatic metabolism by CYP3A, CYP2D15 (dogs), and CYP1A (cats) enzymes [136] [137]. Oral bioavailability is approximately 20% in dogs and 50% in cats [138]. Neurokinin receptor antagonism by maropitant reduces binding of the neuropeptide neurotransmitter substance P. Substance P is intimately involved in the brainstem nuclei (area postrema, nucleus tractus solitarius, and vagal dorsal motor nucleus) facilitating emesis [139]. The most frequently encountered and reported adverse effect of maropitant is pain on injection [140]. The commercial preparation is formulated with β‐cyclodextrin to increase solubility and the binding relationship is temperature dependent. Metacresol is included as a preservative. Until recently, free, unbound maropitant was suspected to cause pain on injection and refrigerating the solution was investigated as a means of reducing this reaction. An injectate temperature of 4 °C was associated with a mild response (“twitching of the skin, licking of the fur, or digging at the injection site”) in one out of 17 dogs; the remaining 16 dogs did not react to injection. In contrast, injections at 25 °C were associated with a painful response in nine out of 17 dogs, four of which were classified as moderate–severe (“short‐term vocalization, jumping, wincing, prolonged yelping or aggression”) [140]. This pattern of responses was reflected in concurrent visual analog scale (VAS) scores, with a significant increase in VAS score associated with the 25 °C injectate temperature compared to the 4 °C injectate temperature (or saline injectate, at both 4 °C and 25 °C) [140]. It appears that there is a role for the preservative, metacresol, in contributing to pain on injection [141]. A generic formulation of maropitant, using benzyl alcohol as a preservative, was found to cause less reaction on SC injection in dogs (total n = 32 dogs, crossover study) when compared with the formulation using metacresol [141]. Pain scores (as assessed with a VAS and a simple descriptive scale) were significantly lower with the benzyl alcohol formulation with no difference between injectate temperatures (4 °C and 25 °C), and VAS scores were approximately one‐quarter to one‐third those recorded for the metacresol formulation. In cats, the manufacturer reports a moderate response (retreating and vocalizing) to SC injection in 22.6% of cats (n = 133 total) receiving 1 mg/kg maropitant compared to 1.6% of cats (n = 62 total) receiving a placebo injection [137]. A significant response (retreating, hissing, scratching, and vocalizing) to SC injection was reported in 11.3% of cats (n = 133 total) given 1 mg/kg SC maropitant and in 1.6% of cats (n = 62 total) receiving placebo. The injectate temperature was not reported. Assessing the response to maropitant injection (1 mg/kg SC) in cats by blinded observers using a VAS found a VAS score ≥ 7/10 cm was awarded in 27.3% of cats (35/128 observations), in contrast with 4% (6/136 observations) in the saline injection group [142]. As an antiemetic, maropitant has been shown to be effective in cats and dogs in a variety of situations, including motion sickness, chemotherapy, and non‐specific gastritis [138,143–149]. Its efficacy has compared favorably to metoclopramide, chlorpromazine, and ondansetron with a range of emetogens and clinical conditions [143,145,150]. Within anesthesia and analgesia, maropitant is primarily used for its antiemetic properties, with much of the work focused on reducing vomiting following opioid premedication (hydromorphone, morphine) [142,151–156]. The potential for maropitant to provide analgesia has also been studied [157–159]. The mechanism of maropitant as a potential analgesic is mediated via NK1 receptors. These receptors have been identified at the following sites: sensory afferent nerves [160], spinal cord [161], brain, and viscera [162]. There is evidence of higher receptor concentrations of substance P in visceral versus somatic afferent fibers [163]. A minimum alveolar concentration (MAC)‐sparing effect ranging from 16–30% has been shown in two nociceptive models: tail clamp (dogs) and ovary and ovarian ligament stimulation (dogs and cats) [157–159]. The range in MAC‐sparing effect may reflect the different study models or doses used in each study, or both. A loading dose of 5 mg/kg IV followed by 150 μg/kg/h resulted in a MAC reduction of 16% after a tail clamp stimulus during sevoflurane anesthesia compared to control [157]. Administration of maropitant via the epidural route did not reduce MAC in the same model, and the authors suggested that inappropriate dose, failure to cross dural membranes, or a restricted site of action may have contributed [157]. In contrast, in a canine ovary and ovarian ligament stimulation model, a lower IV dose of maropitant (1 mg/kg IV followed by 30 μg/kg/h) resulted in a 24% decrease in the MAC of sevoflurane (maropitant plasma concentration of 112 ng/mL) [158]. Increasing the maropitant dose to 5 mg/kg IV followed by infusion at 150 μg/kg/h resulted in a small (non‐significant) further reduction in MAC of 30% [158]. A similar study in cats (same nociceptive model and anesthetic agent) but with a single IV bolus of maropitant (1 mg/kg) resulted in a MAC reduction of 15% [159]. Increasing the dose of maropitant (5 mg/kg) did not lead to a further decrease in MAC [159]. When maropitant (1 mg/kg SC) is given in conjunction with a multimodal analgesic protocol (including methadone and meloxicam), in a randomized, blinded study, to cats undergoing ovariohysterectomy, the impact on reducing isoflurane requirement is less marked (approximately 0.25% isoflurane difference) [164]. In a randomized, blinded, controlled study, providing maropitant by continuous rate infusion (CRI) (30 or 100 µg/kg/h, after a 1 mg/kg IV loading dose) to cats undergoing ovariohysterectomy also receiving morphine (0.3 mg/kg IM) and acepromazine (0.05 mg/kg IM), maropitant was associated with lower postoperative pain scores compared with the control group [165]. However, there was no advantage to maropitant over other treatments (lidocaine CRI, ketamine CRI, lidocaine–ketamine CRI ± maropitant). A canine MACBAR study (n = 6 dogs) reported reductions in sevoflurane requirements of approximately 15% when maropitant (1 mg/kg SC) or maropitant combined with carprofen (4 mg/kg SC) was administered 60 min before an electrical stimulus [166]. Maropitant’s antiemetic effect has been documented in dogs and cats receiving hydromorphone [151,152], morphine [142,153–156], or xylazine [138]. In cats, 1 mg/kg of maropitant administered 2 h before xylazine (0.44 mg/ kg IM) significantly reduced emesis by 76%, 90%, and 100% for the SC, PO, and IV routes of administration, respectively [138]. Additionally, administration of 1 mg/kg maropitant orally 24 h before xylazine administration resulted in a 66% reduction in emetic events [138]. Over a similar timeframe, female cats given maropitant (1 mg/kg SC) 20 h before premedication with morphine (0.1 mg/kg IM) and dexmedetomidine (0.02 mg/kg IM) vomited less (1/32 cats) than those given saline (20/34) [142]. When 1 mg/kg of maropitant was administered SC 1 h before hydromorphone (0.1 mg/kg IM) in healthy dogs (n = 9), emesis, retching, and nausea did not occur [151]. This compared to incidences of emesis, retching, and nausea of 66%, 11%, and 22%, respectively, in the saline control group (n = 9). Compared to the use of acepromazine as a strategy for reducing emesis following hydromorphone administration, maropitant is more effective [167,168]. When given IV to dogs 45–60 min before premedication with IM hydromorphone (0.1 mg/kg) and acepromazine (0.03 mg/kg), maropitant (1 mg/kg) prevented retching and vomiting (0/13 dogs). This contrasted with the saline control group, in which 6/13 dogs retched or vomited [152]. The same study did not find any difference in the incidence of gastroesophageal reflux between treatment groups (induction of anesthesia with propofol, maintenance with isoflurane, anesthesia time approximately 2.5 h). The time between administration of maropitant and the agent likely to cause emesis is important [154,168,169]. When maropitant (1 mg/kg SC) was given simultaneously with or 15, 30, 45, or 60 min before hydromorphone (0.1 mg/kg IM), retching or vomiting occurred in the simultaneous and 15‐min treatment groups (60% and 20%, respectively) but not in the 30‐, 45‐ or 60‐min groups (n = 10 dogs per treatment group) [169]. When maropitant (1 mg/kg SC) was given concurrently with morphine (0.5 mg/kg IM) and acepromazine (0.05 mg/kg IM), the incidence of retching and vomiting (70% and 50%, respectively) was similar to the saline control group (n = 20 dogs per treatment group) [154]. By contrast, giving maropitant 30 min before morphine–acepromazine reduced the incidence of retching and vomiting (25% and 15%, respectively). Oral administration of maropitant (2–4 mg/kg) given 2 h before hydromorphone (0.1 mg/kg IM) in dogs appears similarly effective to SC administration in preventing vomiting [169]. Giving maropitant orally (1–3 mg/kg) up to 19 h before an emetogen (cisplatin) was effective in significantly reducing incidence of vomiting [144]. In cats, oral maropitant (2.5–3 mg/kg) given approximately 2 or 18 h before premedication with morphine (0.1 mg/kg IM) and dexmedetomidine (0.02 mg/kg IM) was effective at reducing the incidence of retching and vomiting (30–40% in the control group versus 4–13% in the maropitant group) [170,171]. The antiemetic efficacy of maropitant in the face of a wide range of emetogens likely reflects its central site of action at the common pathway governing emesis. The impact of maropitant on nausea has been less clear than with retching/vomiting, reflecting differences in classifying nausea, blinding to treatment groups, choice of emetogen, and timing of administration [142,143,153–156,164,169–174]. Overall, maropitant is less effective in preventing nausea than retching/vomiting, with most studies showing no difference compared with saline control [142,143,153–155,164,170–174]. Compared to metoclopramide and ondansetron, in a low‐dose cisplatin canine model for nausea, ondansetron outperformed both maropitant and metoclopramide [143]. Metoclopramide (0.5 mg/kg IV) did not reduce signs of nausea compared to saline control and maropitant (1 mg/kg IV) showed a minimal improvement, whereas ondansetron (0.5 mg/kg IV) attenuated signs associated with nausea. The authors suggested that 5‐HT3 serotonergic receptors (for which ondansetron is an antagonist) play a more important role in mediating nausea than NK1 receptors. In that study, the antiemetics were infused over 15 min beginning 45 min after cisplatin administration. The same study found arginine vasopressin and cortisol served as biomarkers for nausea. Similarly, no advantage of maropitant (1 mg/kg SC) over metoclopramide (0.5 mg/kg SC) for preventing nausea was found in dogs premedicated with morphine (0.5 mg/kg SC) 45 min after maropitant or metoclopramide [155]. Limited evidence suggests a potential improvement in return to feeding and food consumption associated with maropitant [156,175]. A study from the manufacturer found that dogs undergoing ovariohysterectomy or castration surgery returned to feeding faster and consumed significantly more food when treated with maropitant (1 mg/kg SC, 45 min before morphine 0.5 mg/kg SC) versus saline [156]. These data should be interpreted with caution as perioperative analgesia was limited to a single dose of morphine and the presence of postoperative pain not assessed. In a comparison of preanesthetic treatment with maropitant (1 mg/kg SC) or morphine (0.5 mg/kg SC) in dogs undergoing ovariohysterectomy surgery, a higher proportion of dogs in the maropitant group (64.7% [11/17] versus 15.3% [2/13] in the morphine group) ate within 3 h of extubation [175]. Relatively high numbers of dogs in each treatment group received postoperative rescue analgesia with IV morphine (13/17 in the preanesthetic maropitant group and 10/13 in the preanesthetic morphine group). Further work is needed to corroborate these findings but improvements in return to normal function fit with the concept of enhanced recovery after surgery [176–178]. As the popularity of maropitant has grown, there is evidence that it is inappropriately prescribed in dogs for indications for which it has no efficacy [179]. The current label dose of the injectable maropitant formulation (10 mg/mL) in dogs is 1 mg/kg SC or 1 mg/kg IV (in dogs over 4 months old, over 1–2 min). In cats (over 4 months old), maropitant is labeled for IV use at 1 mg/kg over 1–2 min. Maropitant administered IV during general anesthesia (1 mg/kg over 2 min) in dogs premedicated with acepromazine (0.005 mg/kg IM)–butorphanol (0.2 mg/kg IM) or dexmedetomidine (0.005 mg/kg IM)–butorphanol (0.2 mg/kg IM), followed by induction of anesthesia with propofol and maintenance with isoflurane, has the potential to decrease arterial blood pressure [180]. The reduction in arterial blood pressure was greater in the acepromazine–butorphanol group, with a short period of mean arterial blood pressure below 60 mmHg during the period of injection. Minimal changes were observed in the dexmedetomidine–butorphanol group. Statistically significant decreases in arterial blood pressure (of approximately 10–15 mmHg) were observed in awake dogs, with a compensatory increase in heart rate. The mechanism of action underlying these blood pressure changes is unknown. Pharmacokinetic data for maropitant are available for cats, dogs, chickens, horses, and rabbits [137,181–185]. Methocarbamol is a centrally acting muscle relaxant that selectively inhibits spinal and supraspinal polysynaptic reflexes through its action on interneurons, without direct effects on skeletal muscle [186,187]. It is commonly used in human medicine for back pain with a muscular component [188]. Use in veterinary medicine has historically been associated with tetanus [189–191], metaldehyde, 4‐aminopyridine and pyrethrin/permethrin toxicity [192–198], and exertional rhabdomyolysis [199–200]. More recently, it has been successfully used for the management of acute muscle strain in combination with rest, physical therapy, and NSAIDs [201]. Higher doses may result in sedation, and this should be accounted for when used in combination with sedative and anesthetic agents. Pharmacokinetics have been studied in dogs [202] and horses [199,200,203–205]. Therapeutic serum concentrations are achieved rapidly by an oral route of administration in dogs and horses [202,203]. Extensive hepatic metabolism (dealkylation and hydroxylation followed by conjugation) is followed by primarily urinary excretion of metabolites [202]. Guaifenesin is a metabolite of methocarbamol produced in low concentrations in horses [199,200]. The recommended dose of methocarbamol in cats and dogs is 40–60 mg/kg (PO, IV) three times daily for one day followed by 20–40 mg/kg (PO, IV) three times daily until symptoms resolve [201]. Higher doses may result in toxicity and potential effects of co‐administration of drugs with CNS depressant effects should be considered [189,191,206]. One case series reported doses of 55–100 mg/kg IV every 30–60 min in dogs with tetanus, though its efficacy was questioned when compared to other muscle relaxants/sedatives employed [191]. Per rectum administration (55–200 mg/kg, up to 330 mg/kg/day per rectum every 6–8 h) has been reported when an IV formulation was not available [206]. Its use is associated with few adverse effects, with a human study finding the incidence of adverse events similar to that of placebo [188]. Administration of methocarbamol at therapeutic doses does not result in cardiopulmonary changes. Overdose may result in sedation and excessive muscle weakness with any effects being short‐lived. Methocarbamol is available as 500 mg and 750 mg tablets, and as a 100 mg/mL solution for injection. Metoclopramide is primarily indicated as an antiemetic in small animals. It is less effective than maropitant as an antiemetic and its prevention of nausea varies between studies [143,150,155,207]. It has also been investigated for use in promoting gastric emptying and reduction of gastroesophageal reflux [208–210]. Metoclopramide is believed to increase gastroesophageal (lower esophageal) sphincter tone [211,212] and promote peristalsis of the duodenum along with an improvement in antropyloroduodenal coordination. Together, these effects promote gastric emptying in healthy dogs and cats [210,213]. Supporting evidence for its use in promoting gastric emptying in clinical cases is limited [208]. Following a dose of 0.3 mg/kg in dogs 1 week after gastropexy for correction of gastric dilatation‐volvulus, metoclopramide did not promote gastric emptying [208]. Using manometry in conscious Beagles, a comparison of oral cisapride (0.5 mg/kg), metoclopramide (0.5 mg/kg), and placebo (gelatin capsule) found that only cisapride induced a significant increase in gastroesophageal sphincter pressure, with increased pressures at 4 and 7 h after administration [214]. Pressures recorded following metoclopramide did not differ from placebo. In a comparison of two doses of metoclopramide (loading dose of 1 mg/kg then 1 mg/kg/h IV, or 0.4 mg/kg then 0.3 mg/kg/h IV) in dogs undergoing orthopedic surgery, the low and high doses of metoclopramide resulted in a reduction of the risk of gastroesophageal reflux by 34% and 54%, respectively [209]. Given the low risk of clinical sequelae following gastroesophageal reflux, the case for preemptive therapy is unclear. In dogs with laryngeal paralysis, a population susceptible to aspiration pneumonia, administration of metoclopramide (1 mg/kg IV loading dose followed by 1 mg/kg/h CRI) had no impact on the incidence of aspiration pneumonia (2/28 dogs in the control group, 4/33 dogs in the metoclopramide group, relative risk 0.59, 95% CI 0.12–3.0) [215]. The anesthetic protocol was standardized (no premedication, anesthetic induction with propofol, maintenance with isoflurane or sevoflurane, and buprenorphine 0.02 mg/kg IV given after orotracheal intubation). Metoclopramide has multiple sites of action, including serotonin receptor antagonism in the CNS and dopamine (D2) receptor antagonism in the CNS and gastrointestinal tract [139]. Its dopamine antagonist activity can result in extrapyramidal signs (muscle spasms, restlessness), even at recommended doses, as has been reported in humans, cattle, and a dog [216–218]. Metabolism is primarily hepatic with a high first‐pass effect [219]. The recommended doses in dogs and cats are 0.25–0.5 mg/kg IV, IM, or PO every 8–12 h (higher doses may be required in refractory cases). For CRI, a loading dose of 0.4 mg/kg IV followed by 0.3–1 mg/kg/h is recommended. The administration of metoclopramide in horses and ponies has shown limited efficacy [220]. Doses of 0.06–0.25 mg/kg caused restlessness, sedation, and occasionally colic. Lack of supporting evidence for beneficial large intestinal effects does not indicate its use in colic [221]. Use in cattle is limited as the dose effective at increasing reticular motility (0.3 mg/kg IM) in healthy animals is associated with extrapyramidal signs (restlessness then depression) [216]. The effects of the reported effective dose were short‐lived, lasting approximately 20 min. Similarly, in calves, metoclopramide (0.1 mg/kg IM) was no better than saline at increasing abomasal motility or emptying rate [222]. Available formulations include 5 and 10 mg tablets, a 1 mg/mL oral solution, and a 5 mg/mL injectable solution. Ondansetron and dolasetron are serotonin (5‐HT3) receptor antagonists often administered as antiemetics before chemotherapy [223]. Ondansetron has been shown to be highly effective in cisplatin‐induced nausea and emesis in dogs and doxorubicin‐induced emesis in ferrets [143,224]. Cisplatin induces emesis through peripheral activity on the gastric mucosa to activate 5‐HT3 receptors in vagal and sympathetic afferents to the emetic center of the brainstem [225,226], and ondansetron and dolasetron block this pathway at the level of the emetic center. This mechanism of action differs from the centrally mediated antiemetic effects of maropitant (see above). Evidence suggests that these drugs can be successful in treating nausea and vomiting associated with renal and hepatic disease as well as gastroenteritis in dogs and cats [227–229]. Ondansetron has also been used successfully in treating a Chihuahua with obstructive sleep apnea, a condition in which serotonin plays a role in respiration and airway patency [230]. In comparisons between maropitant and ondansetron, the results are mixed, probably reflecting differences in cause of vomiting (peripherally versus centrally acting emetogens). Ondansetron (0.5 mg/kg PO or IV) has been shown to be less effective than maropitant in preventing emesis resulting from apomorphine administration, premedication with hydromorphone (0.1 mg/kg IM)–acepromazine (0.02 mg/kg IM)–glycopyrrolate (0.01 mg/kg IM), and following tranexamic acid administration in dogs, but more effective in a cisplatin model of nausea in dogs, and similarly effective in the management of emesis associated with parvoviral enteritis [143,150,174,228,231]. Evidence from clinical human literature does not suggest a difference in efficacy between dolasetron and ondansetron [232]. A similar comparison has not been studied in a clinical veterinary setting. While adverse effects have not been reported in dogs and cats, in 2010, the United States Food and Drug Administration released a drug safety communication contraindicating the use of injectable dolasetron for the prevention of nausea and vomiting associated with chemotherapy in humans [233]. This resulted from evidence of electrocardiographic QT prolongation and torsade de pointes associated with IV administration. The warning did not extend to oral therapy. Pharmacokinetics of ondansetron and dolasetron have been reported in dogs and cats [234–237]. In the dog, dolasetron undergoes hepatic metabolism, producing the active metabolite reduced dolasetron. This metabolite and the unchanged drug are both excreted in urine and feces [238]. Ondansetron metabolism is mediated by CYP3A4 in the liver with excretion of metabolites in urine and feces [239]. The pharmacokinetics of ondansetron are altered in cats with hepatic disease, with reduced clearance and increased plasma concentration [237]. Ondansetron dose in cats and dogs is 0.5–1 mg/kg twice daily (IV or PO) or 30 min before chemotherapy. A lower dose may be effective in reducing emesis associated with other emetogens. For example, 0.22 mg/kg IV reduced the incidence of emesis when co‐administered with dexmedetomidine in cats [240]. The dose of dolasetron in dogs and cats is 0.6–1 mg/kg IV or PO, once daily. Available formulations of ondansetron include 4 and 8 mg tablets, a 4 mg/5 mL oral solution, and a 2 mg/mL injectable solution. Dolasetron is available as 50 and 100 mg tablets and a 20 mg/mL solution. Procainamide is a Class 1a (Singh‐Vaughan Williams and Keefe classifications) antiarrhythmic agent with efficacy for the treatment of supraventricular and ventricular tachyarrhythmias. It is a derivative of the local anesthetic procaine (ester linkage replaced with amide). Through sodium channel blockade, it reduces the rate of rise of phase 0 of the cardiac action potential, raises the threshold potential, and prolongs the refractory period. It also has some anticholinergic effects. It is often used as a second‐line therapy for ventricular tachycardia (VT) resistant to lidocaine. A comparison between procainamide and lidocaine for the management of postoperative ventricular arrhythmias (i.e., ventricular premature contractions (VPCs), VT, R‐on‐T phenomenon, and multiform VPCs) in a mixed population of medium‐to‐large breed dogs showed these drugs to be equally effective in restoring sinus rhythm [241]. Procainamide was administered as a loading dose of 10 mg/kg IV over 5 min then an infusion of 20 μg/kg/min IV (lidocaine loading dose of 2 mg/kg IV, followed by 60 μg/kg/min IV). No adverse effects were associated with procainamide administration. Efficacy in the treatment of atrial fibrillation is mainly from experimental models of induced arrhythmia studies in dogs, with one recent case report [242,243]. The clinical case report describes the successful use of procainamide (14.3 mg/kg IV over 15 min) to treat atrial fibrillation associated with pericardiocentesis and unresponsive to a single dose of lidocaine (1.2 mg/kg IV). Procainamide administration resulted in cardioversion to a sinus rhythm [242]. In Boxers with familial ventricular arrhythmias (including arrhythmogenic right ventricular cardiomyopathy), two studies have reported the efficacy of antiarrhythmic agents in terms of median survival time [244] and response of ventricular arrhythmias [245]. Caro‐Vadillo et al. did not find a significant effect on median survival time (age and the presence of syncope were better predictors) between the following treatments: sotalol, mexiletine–atenolol, or procainamide (20–26 mg/kg PO every 8 h) [244]. Meurs et al., in a comparison between procainamide (20–26 mg/kg PO every 8 h), atenolol, sotalol, or mexiletine–atenolol, found that only sotalol or mexiletine–atenolol were effective at reducing the number and severity of VPCs and heart rate [245]. However, none of the treatments resulted in an improvement in the incidence of syncope, though it is possible that the study was underpowered for this outcome. There is limited evidence of its use in horses, with one report of failed therapy for ventricular extrasystoles and VT at a total dose of 20 mg/kg over two administrations given at a 2‐h interval [246]. In a case report of a horse with atrial fibrillation, procainamide (20 mg/kg IV) was included as part of a successful treatment regimen (with sotalol and rest) following semisuccessful electrical cardioversion that converted the cardiac rhythm from atrial fibrillation to atrial tachycardia with variable AV conduction [247]. The pharmacokinetics of procainamide have been reported in horses where, unlike dogs [248], the active metabolite NAPA (N‐acetylprocainamide) is produced which has class III antiarrhythmic activity (i.e., it prolongs the cardiac action potential) [249]. Recently, the pharmacokinetics of a sustained‐release formulation of procainamide have been reported in dogs [250]. An effective dose in dogs is 10 mg/kg IV over 10 min followed by an infusion of 20 μg/kg/min [241]. Single or repeated bolus injection of 1–2 mg/kg IV may be effective in dogs for the management of short‐duration ventricular ectopic activity associated with surgical manipulation or catecholamine release. The recommended dose in cats is a loading dose of 1–2 mg/kg slowly IV followed by an infusion of 10–20 μg/kg/min. Toxicity manifests as a widening of the QRS complex, additional arrhythmias, and hypotension [245]. It is available as 250, 375, and 500 mg tablets and a 100 mg/mL solution for injection. Sodium nitroprusside (SNP) elicits arterial and venous dilation by liberating the potent endogenous vasodilator nitric oxide (NO). Cyanide ions are also produced as a by‐product. For an in‐depth review of NO physiology, the reader is referred to [251]. Following administration of SNP, its vascular effects are mediated locally in the vascular endothelium and underlying smooth muscle. NO production (from reaction with oxyhemoglobin, also generating methemoglobin) activates soluble guanylyl cyclase, increasing levels of cyclic guanosine monophosphate (cGMP) in the vascular musculature. cGMP inhibits entry of calcium into smooth muscle cells and increases uptake of intracellular calcium by smooth endoplasmic reticulum, resulting in vasodilation. When delivered by IV infusion, SNP has a rapid onset and offset, allowing titration to effect. A dose range of 1–5 μg/kg/min has been reported to successfully reduce arterial blood pressure in a dog with phenylpropanolamine overdose and SNP has been used to induce hypotension in experimental studies in horses (0.1–1.5 μg/kg/min) and pigs (0.5–5 μg/kg/min) [252–255]. Close monitoring of systemic arterial blood pressure and heart rate and rhythm is essential due to the risk of inadvertent hypotension or reflex tachycardia. Use of SNP during CPR (“sodium nitroprusside enhanced CPR”) has been shown to provide neuroprotection in experimental models of cardiac arrest, though it is not currently known if this will translate to clinical use [256]. The rapid offset of SNP results from the short half‐life of NO, which is rapidly oxidized to nitrite [257]. Cyanide ions, produced with NO from SNP, are metabolized by the liver to thiocyanate, which is then excreted by the kidneys [258]. In cases of overdose or hepatic or renal insufficiency, there is a risk of cyanide and thiocyanate toxicity. Toxicity manifests as tachycardia, hyperventilation, metabolic acidosis (as cyanide binds cytochrome oxidase, thereby inhibiting aerobic metabolism), and seizures. Toxicity can be treated with thiosulfate (6 mg/kg/h IV in dogs) [259–261]. Solutions of SNP are light sensitive, turning from a light orange/straw‐colored solution when fresh (reconstituted with 5% dextrose) to dark brown/blue upon exposure to light. The solution should be discarded if this occurs. Nitroprusside is available in 10 and 25 mg/mL solutions for injection following dilution. Trazodone is a serotonin antagonist and reuptake inhibitor, with dose‐dependent antagonist activity at 5‐HT2A and 5‐HT2C receptors and an inhibitory effect on serotonin reuptake [262]. As such, it may contribute to serotonin syndrome, when given concurrently with selective serotonin reuptake inhibitors, tricyclic antidepressants, or monoamine oxidase inhibitors [263,264]. It also has antagonist effects at histamine (H1A) receptors and α1‐ and α2‐adrenergic receptors [262]. Trazodone has become increasingly popular over recent years, prescribed for fear management and anxiolysis on a regular basis and perioperatively [263,265]. It is prescribed in humans for sleep disorders arising from a variety of causes. Despite widespread use, there is limited peer‐reviewed veterinary literature available. In an experimental cat study (n = 6), trazodone reduced activity (assessed via accelerometry) to 43–86% of pre‐dosing values, with doses of 50, 75, and 100 mg/cat (corresponding to 10.6–16.7 mg/kg, 16–25 mg/kg, and 21–33 mg/kg PO, respectively) [266]. The highest dose (100 mg/cat) was most effective in reducing activity, with a peak effect at 2.5 h after dosing, but considerable interindividual variability in baseline and post‐trazodone activity was reported. However, behavioral responses to a comprehensive physical examination (including abdominal palpation, otoscopic and ophthalmic examination, and mock venipuncture and cystocentesis with needle penetration of skin) performed 90 min after dosing were unchanged compared to placebo. Similarly, a stress score did not identify differences between placebo and the 100 mg/kg treatment. Heart and respiratory rates were unaffected by trazodone compared with placebo. In a double‐blinded, placebo‐controlled, randomized crossover study (n = 10 cats) evaluating the effect of trazodone on transport and handling, trazodone (50 mg/cat, approximately 11 mg/kg PO) reduced signs of stress during transport and improved ease of handling [267]. Reduced stress (from “very tense” to “weakly relaxed”) and improved tractability (from “restraint needed to examine safely” to “easy/relatively easy to examine”) were identified. Sleepiness was identified as an adverse effect. Trazodone was given approximately 1–1.5 h before transport to the clinic and 1.5–2 h before physical examination. As with cats, data from dogs is from a small number of studies, with variability in reported responses to trazodone. A randomized, placebo‐controlled, double‐blinded study did not identify differences in behaviors between treatments [268]. Dogs (n = 29) received either placebo or trazodone (2.8–10.8 mg/kg PO, every 12 h) with trazodone dose adjusted according to owner’s wishes during a 4‐week period following elective orthopedic surgery, during which activity restriction and confinement were prescribed. Behavioral assessments included greeting behavior, calmness, behavior on leash, and tolerance of confinement. The authors suggested several reasons for trial failure, including selection bias toward calmer dogs, caregiver placebo effect (owners performed behavioral assessments), placebo‐by‐proxy effect (owner beliefs altered interaction with their dog leading to a change in dog behavior), and lack of sensitivity in the behavioral assessment instrument. By contrast, a recent double‐blinded, placebo‐controlled crossover study in dogs (n = 20) found that trazodone (9–12 mg/kg PO) given 90 min before transport to a veterinary clinic was associated with reduced stress scores in the clinic, with most owners (18/20) correctly identifying when trazodone had been given [269]. Two earlier studies in dogs showed efficacy with trazodone, though both had limitations in study design, limiting data interpretation. A study of 36 dogs admitted for elective orthopedic surgery all received trazodone postoperatively for 4 weeks, with a subset of owners requesting a dose increase after week 2 (to 7 mg/kg PO every 8 h) [270]. Owners assessed behaviors (greeting behavior, calmness, behavior on leash, and tolerance of confinement). Compared to preoperative behavioral assessments, trazodone was associated with reduced intensity of greeting and overall calmness, but not response to owner commands or tendency to pull on leash walks. Trazodone was assessed as improving tolerance of postoperative confinement. Most owners reported an onset of drug effects within 45 min of dosing (16–90 min for 90% of owners) and a median duration of action of > 4 h (upper limit of observation period). A study of hospitalized dogs (most dogs were admitted to the surgery service) evaluated a convenience sample of 59 dogs prescribed trazodone (4.7 ± 1 mg/kg PO) by the supervising veterinarian, with a comparison group of dogs not prescribed trazodone (n = 58) [271]. Stress‐related behavior scores were higher in the trazodone treatment group at baseline compared with the untreated group, but there was a significant reduction in these scores when assessed approximately 110 min following trazodone administration. One dog showed signs of aggression 60 min after trazodone administration. In horses, 6 mg/kg PO induced mild sedation (based on a simple descriptive scale) in 7/8 horses, which lasted between 0.5 and 8 h after trazodone administration, with 4/8 horses remaining sedated at 12 h and no signs of sedation apparent at 24 h [272]. Higher doses (7.5 and 10 mg/kg PO) caused sedation within 20–30 min of administration that was generally mild (3/6 horses were heavily sedated at the higher dose) and lasted a few hours [273]. In the latter study, arrhythmias diagnosed by thoracic auscultation, without an ECG, were noted in 2/6 horses that received 10 mg/kg PO. In goats, intended as a model for wild ruminants, a dose of 10 mg/kg PO resulted in a five‐ to sixfold increase in time spent sleeping and lying down, with reduced activity lasting approximately 4 h [274]. Eating and ruminating were unaffected. Two studies have evaluated the effect of trazodone on the propofol requirement for induction of general anesthesia in dogs [275,276]. A retrospective study of dogs (n = 15) undergoing radiotherapy treatment showed a significant reduction in propofol dose (approximately 25%) when trazodone was given approximately 2 h before anesthesia (4.8 [2.9–7.3] mg/kg PO) [275]. Dogs were premedicated with alfentanil and atropine and served as their own controls. A prospective, randomized, unblinded study did not find an effect of trazodone (5–7 mg/kg PO 2 h before anesthesia) on the propofol dose requirement (dose administered over 20–30 seconds) compared with acepromazine (0.01–0.03 mg/kg IM 30 min before anesthesia) [276]. All dogs (n = 15 per treatment) were also given morphine (1 mg/kg IM 30 min before anesthesia). A MAC‐sparing effect has been identified in dogs, with a 17% reduction in isoflurane MAC following trazodone (8 mg/kg PO) [277]. Trazodone is metabolized to an active metabolite, meta‐chlorophenylpiperazine, which has agonist activity at the 5‐HT2C receptor, though its significance in animal species is unknown [273,278]. The primary route of elimination is renal (approximately 75%) with the remainder undergoing fecal elimination. Pharmacokinetics have been described in dogs (PO, IV, per rectum), horses, pigeons, and goats [272,274,278–281]. Time to peak effect appears to vary markedly between individual dogs, with peak plasma concentrations achieved 445 ± 271 min after 8 mg/kg PO (n = 6 dogs), though onset of signs may be apparent within 45 min [270,280]. Pharmacokinetics have not been described in cats, but variability in behavioral responses may also reflect interindividual pharmacokinetic differences [266]. Adverse effects appear uncommon in dogs following PO dosing, with one report of hepatotoxicity in a dog attributed to trazodone (4 mg/kg PO once or twice daily) and reports of aggression following IV (3/6 dogs) and PO (1/59 dogs) administration [271,280,282]. Trazodone at doses between 1 and 30 mg/kg IV results in transient, dose‐dependent reductions in heart rate and arterial blood pressure [283]. In cats (n = 12) given trazodone (approximately 11 mg/kg PO) 90–120 min before echocardiography, sedation was observed but there were minimal changes in cardiac measurements [284]. In horses, 4/8 horses exhibited sweating following oral trazodone (6 mg/kg), while IV dosing was associated with aggression, ataxia, tremors, and circling (2/2 horses, 2 mg/kg) and ataxia, tremors, circling, and sweating (1.5 mg/kg) [272,278]. Reported doses in dogs range from 3 to 12 mg/kg PO every 8–12 h [268,269,271,280] and those in cats range from 8 to 30 mg/kg PO [266,267,284]. Available formulations include 50, 100, 150, and 300 mg tablets. The primary perioperative indication for vasopressin is in CPR and refractory hypotension [84,285–288]. It is a potent vasoconstrictor, acting on V1a receptors located in vascular smooth muscle, though it also displays activity at V1b (anterior pituitary) and V2 (renal collecting duct) receptors, and inhibits nitric oxide production [289,290]. Its analog, desmopressin (DDAVP), is used in the treatment of central diabetes insipidus [291–293] and management of coagulopathy [294–296]. Vasopressin is an alternative vasopressor to epinephrine for use during CPR, though there is no clear evidence supporting its role as a replacement for epinephrine. Recent veterinary CPR guidelines suggest its use (0.8 U/kg IV) on alternating rounds of CPR instead of epinephrine, though there is no evidence that it confers a benefit over epinephrine in humans [84,297]. Due to the lack of β1‐adrenergic receptor activity, it may reduce the risk of myocardial ischemia compared with epinephrine, though it will still cause coronary vasoconstriction. An additional potential advantage over epinephrine is that V1 receptors, unlike α1‐adrenergic receptors, remain responsive in an acidic environment [298]. Reports of clinical use in the veterinary literature are mixed [285,287], with vasopressin showing no benefit over epinephrine in a randomized comparison using a standardized CPR technique [287] but associated with an improved rate of return to spontaneous circulation in a hospital‐wide study [285]. A meta‐analysis demonstrated a potential improvement in return of spontaneous circulation associated with its use, though contributing studies were predominantly in porcine models of ventricular fibrillation [288]. Vasopressin can be administered via the endotracheal route, if necessary, with one animal study suggesting that the same dose as that recommended for IV use is effective [299]. What has become clear from the Reassessment Campaign on Veterinary Resuscitation (RECOVER) initiative [300] is that there is wide variability in study design and reporting of animal models of CPR, making it difficult to draw firm conclusions from currently available experimental evidence. Anecdotal reports suggest the use of vasopressin in cases of refractory hypotension, though the available veterinary literature is sparse and human studies are contradictory [286,290,301–304]. This is likely a reflection of the heterogeneity of clinical and experimental studies [303]. Infusion rates of 0.5–5 mU/kg/min and 0.1–2.5 mU/kg/min have been suggested in dogs and foals, respectively [286,290,301]. Users should be aware of the potential for detrimental hypoperfusion of vascular beds resulting from the resultant vasoconstriction [305]. Desmopressn acetate (DDAVP), a synthetic analog of vasopressin with reduced vascular effects, is used for perioperative management of patients with von Willebrand disease [295,306,307] and management of central diabetes insipidus [291–293,308]. von Willebrand disease, the most common canine hereditary defect of hemostasis, is classified according to one of three subtypes, with type 1 being the least severe and most common, especially in Doberman Pinschers [309]. Management consists of both non‐transfusion and transfusion therapy, based on disease severity and clinical presentation. Non‐transfusion therapy includes appropriate patient management (i.e., good surgical technique, minimizing use of drugs with antiplatelet activity, careful handling) and DDAVP. Administration of 1 μg/kg SC or IV 30 min before surgery may support hemostasis, but the response is more variable in dogs than humans [295,306]. Administering DDAVP intranasally or into the conjunctival sac is commonly performed, but there is limited supporting evidence of its efficacy via this route in von Willebrand disease. Other reported uses for DDAVP include support of hemostasis following administration of aspirin before surgery [310,311], as a prophylactic measure to manage postoperative diabetes insipidus following hypophysectomy [296], and administration to canine blood donors to increase von Willebrand factor production before blood collection for cryoprecipitate [312]. Injectable vasopressin is available as a 20 U/mL solution. DDAVP is available as a 4 μg/mL injectable solution, 0.1 and 0.2 mg tablets, 100 μg/mL spray, and a 0.01% solution for intranasal administration. The dopamine agonist and N‐methyl‐D‐aspartate (NMDA) receptor antagonist amantadine was originally used as an antiviral agent [313,314] but has entered clinical use in human medicine for diseases and presentations as varied as Parkinson’s disease [315], traumatic brain injury (TBI), and pain. Its continued use in the management of Parkinson’s disease arose serendipitously from a patient with Parkinson’s disease noticing an improvement in motor function wtaking amantadine for influenza [316]. Recent meta‐analysis and systematic reviews have indicated a potential role for amantadine in TBI though further studies are required to confirm promising earlier findings [317,318]. Human studies have demonstrated improved arousal when amantadine was administered between 3 days and 4 weeks following TBI, and purported mechanisms are through CNS stimulation mediated by amantadine’s dopaminergic activity and reduced neurotoxicity through its NMDA receptor antagonism [319,320
25
Anesthetic and Analgesic Adjunctive Drugs
Introduction
Non‐analgesic adjuncts
Dantrolene
Diphenhydramine
Doxapram
Famotidine, omeprazole, and pantoprazole
Guaifenesin (GG, glyceryl guaiacolate ether)
Maropitant
Methocarbamol
Metoclopramide
Ondansetron and dolasetron
Procainamide
Sodium nitroprusside
Trazodone
Vasopressin (arginine vasopressin, antidiuretic hormone) and desmopressin
Analgesic adjuncts
Amantadine and memantine
Stay updated, free articles. Join our Telegram channel
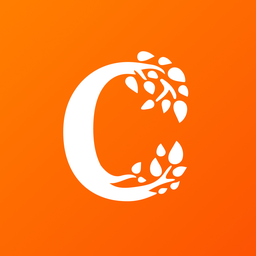
Full access? Get Clinical Tree
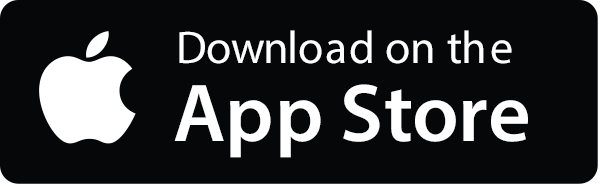
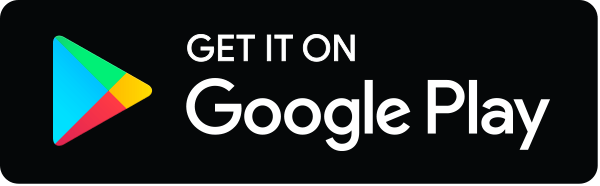