Introduction
Dogs and cats frequently require anesthesia for diagnostic evaluation or surgical correction of neurological disorders. Diagnostic procedures that require either general anesthesia or heavy sedation include electroencephalography (EEG), imaging techniques, and other electrodiagnostic tests. Veterinary neurosurgical anesthesia is more often required in patients with spinal cord rather than intracranial disorders. A frequently performed neurosurgical procedure in veterinary medicine is involved in treatment of intervertebral disk disease. However, the increased use of advanced imaging techniques, such as computed tomography and magnetic resonance imaging (MRI), has led to a greater frequency of intracranial operative procedures in dogs and cats. In animals with neurological disease, consideration of the dynamics of intracranial pressure (ICP), cerebral blood flow (CBF), and cerebrospinal fluid (CSF) production and flow is important in preventing patient morbidity or death. Matching appropriate CBF with cerebral metabolism is critical for optimal homeostasis of the animal with neurological disease. Recognition of the effects of drugs, physiological responses during anesthesia, and therapeutic interventions on CBF is essential in meeting the demands of the patient with intracranial disease.
In normal awake animals, blood supply to the central nervous system (CNS) is controlled by autoregulatory mechanisms. Alteration in CBF can result from a variety of changes in arterial oxygenation, carbon dioxide partial pressure, mean arterial pressure (MAP), and venous outflow. The brain and spinal cord are protected by encasement within the bony skull and vertebral column. Increases in blood flow within the noncompliant cranial vault cause an increase in the intracranial volume.1–3 Once increases in CBF cause the intracranial volume to exceed the limits of effective compliance, ICP increases sharply. When clinical findings suggest ICP is already increased by intracranial masses, trauma, or derangement of autoregulation, extreme care is required, because slight changes in intracranial volume greatly increase ICP.2 Significant ICP increases may lead to cerebral ischemia and brain herniation.1
Figure 13.1. Effects on cerebral blood flow (CBF) caused by independent changes in PaO2, PaCO2, and mean arterial pressure (MAP).

Autoregulation of CBF
Autoregulation of brain blood flow is usually very effective in a systemic mean arterial blood pressure range of approximately 50–150 mm Hg. Within this range of blood pressure, many factors—including intracranial tumors, hypercapnia, severe hypoxia, and many anesthetics—interfere with autoregulation and cause changes in ICP (Figure 13.1).1,4,5 Blood vessels in the brain supplying diseased or neoplastic tissues may be fully dilated and unaffected by normal autoregulation mechanisms.
The CNS depression of general anesthesia is usually accompanied by a decrease in cerebral metabolic rate (CMR) and cerebral metabolic requirement for oxygen (CMRO2). This decrease in oxygen requirement is thought to be protective in the possible event of relative ischemia during anesthesia and neurosurgery. There are conflicting reports on the efficacy of various anesthetics in reducing CMRO2, just as there are with regard to the relative effects of the anesthetics on CBF and ICP. Isoflurane, sevoflurane, etomidate, and the barbiturates are generally recognized as contributing substantially to the reduction of CMRO2, affording some cerebral protection.5
In patients with preexisting elevated ICP, further increases can be caused by gravitational or positional interference with drainage of venous blood from the head. Obstruction by occlusion of jugular veins through surgical positioning of the head, use of a neck leash, or venous occlusion to obtain blood samples or placement of jugular vein catheters can rapidly cause dangerous increases in ICP.6 For intracranial neurosurgery, a slight elevation of the head above the level of the heart (with the neck in a neutral position) will facilitate venous drainage, lowering ICP. Extreme elevation is avoided to minimize the risk of venous air embolization.3
Only at very low arterial oxygen tensions does the CBF change in response to oxygen partial pressure. When arterial oxygen partial pressure (PaO2) decreases below a threshold of 50 mm Hg, CBF increases (Figure 13.1). The relationship between arterial carbon dioxide partial pressure (PaCO2) and CBF, on the other hand, is linear. CBF increases by about 2 mL/min/100 g of brain tissue for every 1-mm Hg increase in arterial carbon dioxide over the range of PaCO2 from 20 to 80mmHg.7 Hyperventilation has been used extensively in neuroanesthesia to reduce CBF (via cerebral vasoconstriction). This maneuver decreases tissue bulk, facilitating intracranial surgery. Although quite effective, this technique is somewhat controversial in some situations, because a potential exists for the diversion of remaining blood flow preferentially to diseased tissues lacking autoregulation at the expense of normal brain tissue.8 Deliberate hyperventilation to decrease ICP may be risky when mean arterial blood pressure is reduced to less than 50 mm Hg. The ensuing ischemia could be deleterious to normal brain tissues if a “steal” of CBF diverts remaining blood flow.8,9 The rapid and substantial reduction in CBF and ICP achieved by hyperventilation makes it a valuable tool for the immediate reduction in brain bulk to facilitate intracranial surgery and to reduce acute brain swelling.
Although controversial, restriction of intravenous fluids to only that volume necessary to maintain adequate circulating volume and cardiac output is usually recommended in neurosurgical patients with increased ICP.10,11 Excessive fluid volume has been associated with increased central venous pressure, decreased venous outflow, and increased risk of compounding cerebral edema. Diuretic therapy is frequently indicated in the medical management of patients with intracranial masses and elevated ICP or cerebral edema.6 Dextrose administration is also somewhat controversial and must be individualized to the situation. Hyperglycemia is associated with adverse outcome in animals with cerebral ischemia, and cerebral edema can be exacerbated by administration of isotonic dextrose. However, intravenous dextrose administration decreases the incidence of seizures in patients after metrizamide myelography and is indicated in hypoglycemic seizures or hypoglycemic coma.1,12,13
Glucocorticoids are effective in the treatment of some forms of cerebral edema and have been shown to be effective in reducing the increased ICP that is caused by brain tumors and hydrocephalus. Glucocorticoid therapy may be considered in the management of patients with cerebral edema associated with primary or metastatic brain neoplasia. Since dexamethasone administration has been shown to reduce the rate of CSF formation in dogs, steroid administration may be of some value in the preanesthetic management of hydrocephalic patients considered at risk of further increases in ICP.14,15 Corticosteroids are now known to be contraindicated in cases of CNS trauma.
Sedatives, tranquilizers, and analgesics
For many years, the suspected increased seizure activity associated with administration of the phenothiazine (e.g., acepromazine) and possibly butyrophenone (e.g., droperidol) tranquilizers contraindicated their use in seizure-prone patients and in patients for diagnostic EEG.16 A more recent retrospective study indicates that acepromazine does not potentiate seizure activity.17 Control of seizures with benzodiazepine tranquilizers (e.g., diazepam or midazolam) is desirable in the management of seizure-prone patients but can obscure characteristic patterns in diagnostic EEGs. In addition, benzodiazepines appear to decrease CBF and ICP.18
Evidence for or against the use of alpha2 adrenergic agonists in dogs and cats with neurological disease is scant. Medetomidine administered to isoflurane-anesthetized dogs had no effect on ICP measured using a fiberoptic transducer, whereas antagonism of medetomidine by using atipamezole was associated with a dramatic transient increase in ICP.19 Dexmedetomidine, the pharmacologically active stereoisomer of medetomidine, decreased CBF in both halothane-and isoflurane-anesthetized dogs.20,21 The alpha2 agonists appear to be rational choices to provide sedation for examination or as a preanesthetic medication for patients with neurological disease.
Opioids or neuroleptanalgesic combinations are sometimes used in anesthetic management of patients with increased ICP. The direct effects of opioids on CBF and ICP are minimal. However, opioids may indirectly increase CSF pressure and should be used cautiously in patients with cerebral trauma or space-occupying tumors. Increases in pressure within the cranium may aggravate the underlying condition.
The elevation in CSF pressure is caused by accumulation of carbon dioxide, which in turn is caused by opioid-induced hypoventilation. If a patient is ventilated to prevent hypercapnia, the increase in CSF pressure does not occur when opioids are administered.22 When opioids are used in these cases, the respiratory status must be assessed through arterial blood gas analysis or end-tidal carbon dioxide levels, and when necessary, the patient should be ventilated to prevent hypercapnia. The judicious use of opioids for pain management in the postoperative period often does not cause as much respiratory depression as does pain itself.23
Injectable anesthetics
Ketamine can dramatically increase both CMR and CBF. Telazol® (tiletamine and zolazepam; Pfizer Animal Health, New York) is typically placed in the same category as ketamine; however, studies on CBF and ICP as specifically affected by Telazol are lacking. Combination of ketamine with a γ-aminobutyric acid (GABA) agonist has been shown to ameliorate the effect of ketamine on ICP in dogs.24,25 Whether this is the case for Telazol remains to be proven. In a clinical setting under controlled ventilation, ketamine administration with a GABA agonist and without nitrous oxide has been safely used in neurologically impaired patients.26 Administration of etomidate is associated with regional reductions in blood flow, but this has been associated with exacerbation of ischemic brain injury in human patients.27 Thiobarbiturates, etomidate, and propofol all decrease CMR and CBF, effectively maintaining the coupling between flow and metabolism. Propofol infusion combined with infusion of a short-acting opioid such as fentanyl or remifentanil is a popular anesthetic protocol for neurological patients and provides an effective alternative to the subsequent maintenance of anesthesia using inhalants.
Neuromuscular blocking a gents
The effect of neuromuscular blocking agent s (NMBA s) on ICP will depend on the agent used: most of the nondepolarizing NMBAs such as atracurium, vecuronium, mivacurium, or pancuronium have no significant effect. Agents that are associated with histamine release (e.g., d-tubocurarine) may increase the ICP. Succinylcholine is associated with a direct increase in ICP and should generally be avoided in patients with raised ICP.
Inhalants
All modern volatile anesthetics suppress CMR and, with the exception of halothane, can produce burst suppression of the electroencephalogram. At that depth of anesthesia, CMR is reduced by about 60%. Volatile anesthetics have dose-dependent effects on CBF. In doses lower than the minimal alveolar concentration (MAC), CBF is not significantly altered compared to awake. (The MAC is the lowest end-tidal concentration of inhalant that prevents purposeful movement in 50% of a population.) Beyond doses of 1 MAC, direct cerebral vasodilation results in an increase in CBF and cerebral blood volume. Nitrous oxide has minimal direct effect on ICP when used at concentrations of 50–70% in oxygen. However, nitrous oxide will equilibrate with confined gas spaces and can dramatically increase volume and pressure in these circumstances (e.g., pneumoencephalopathy).
Cardiovascular supportive drugs
Systemic vasodilators (e.g., nitroglycerin, nitroprusside, hydralazine, calcium channel blockers) vasodilate the cerebral circulation and can, depending on MAP, increase CBF (Table 13.1). Vasopressors such as phenylephrine, norepinephrine, ephedrine, and dopamine do not have significant direct effects on the cerebral circulation. Their effect on CBF is dependent on their effect on systemic blood pressure. When MAP is below the lower limit of autoregulation, vasopressors increase systemic pressure and thereby increase CBF. If systemic pressure is within the limits of autoregulation, vasopressorinduced increases in systemic pressure have little effect on CBF.
Table 13.1. Drug effects on CBF and ICP
Increase | Decrease | Minimal or no effect |
Nitroprusside | Benzodiazepines | Phenylephrine |
Nitroglycerin | Opioids | Norepinephrine |
Hydralazine | Thiobarbiturates | Dopamine |
Calcium channel blockers | Propofol | Ephedrine |
d-Tubocurarine | Etomidate | Acepromazine |
Succinylcholine | Dexmedetomidine | |
Inhalant > 1.0 × MAC | Nitrous oxide | |
Ketamine | Inhalant < 1.0 × MAC | |
Atipamezole | Atracurium | |
Mivacurium | ||
Pancuronium | ||
Vecuronium |
MAC, minimal alveolar concentration.
Anesthetic management of specific neurological problems
MRI
Anesthetic concerns during MRI examination center on the potentially dangerous environment of a strong magnetic field.28 Ferromagnetic projectiles have had serious, even lethal, consequences in MRI suites. Anesthetic management of animals undergoing MRI exam can most simply be accomplished by means of injectable agents.29–31 Inhalant anesthetics have been used in MRI suites by distancing the vital ferromagnetic components of the anesthesia machine from the magnetic field. Use of an extended nonrebreathing circuit, such as the Bain circuit, has been described.32 One drawback of this technique is the higher fresh gas flow rates required for larger animals. Another modification of anesthetic technique for MRI involves the use of extended rebreathing hoses with the anesthetic machine placed near the magnet, yet just beyond the critical point of magnetic attraction. For equine MRI, a large animal machine suitable for delivering inhalant anesthetics to horses can be used in this manner. There is anecdotal evidence that prolonged exposure (more than 2 hours) in a 3–5-gauss magnetic field begins to affect vaporizer (Isotec; Datex-Ohmeda, Helsinki, Finland) output such that higher vaporizer settings are required to maintain adequate anesthesia. In human patients, this effect has been reported for Fortec vaporizers (Cyprane, Keighley, UK).33 Although many vaporizers are mainly constructed from nonferrous materials, many do contain components affected by magnetic fields.34
Anesthesia in MRI suites can be monitored by using MRI-compatible equipment to measure the electrocardiograph, blood pressure, pulse oximetry, and airway gases.35 Less expensive alternatives include use of remotely placed monitors with specialized cabling designed to avoid interference with the magnetic field and induction of electric currents leading to burns. Simple systems can be used to measure direct arterial blood pressure, such as the aneroid manometer placed beyond the magnetic field or several feet of extension tubing placed between the transducer and patient. Some dampening and degradation of the arterial pulse wave may occur, but often direct measurement of arterial blood pressure is invaluable to assure maintenance of adequate cerebral perfusion pressure.
Because the magnetic fields vary considerably among various MRI units in clinical practice, consultation with a knowledgeable biomedical engineer is advised prior to implementing a monitoring system or novel means of anesthetic delivery.
Myelography and intervertebral disk disease
For the relatively common surgical procedures to decompress cervical or thoracolumbar intervertebral disk herniation, anesthetic management should address (1) protection from possible seizures and other potential complications associated with administration of myelographic contrast agents, (2) perioperative pain relief, (3) maintenance of adequate spontaneous ventilation, and (4) management of concurrent disorders such as urinary incontinence or other factors predisposing patients to adverse recovery.
Radiographic contrast myelography is sometimes performed in the immediate preoperative period to localize the lesion(s) and to identify the proper site(s) for surgical decompression. As this procedure is often performed during the same anesthetic period, patient management is designed to optimize conditions for both the diagnostic (radiographic) and the therapeutic (surgical) procedures. Dural puncture for sampling of CSF and/or for administration of myelographic contrast agent requires a depth of anesthesia at less than a surgical plane but adequate to prevent patient movement with subsequent trauma.
The incidence of seizure activity and the other potential adverse side effects of myelography appears to be greatly reduced with use of newer contrast agents such as iopamidol and iohexol rather than metrizamide.36,37 Hyperflexion of the cervical spine for cisternal CSF collection and for cervical administration of myelographic contrast can easily kink most endotracheal tubes, causing airway obstruction. Endotracheal tubes that are armored or contain spiral wire are quite resistant to kinking. Metal or other radiopaque reinforcement in the armored tubes makes them unsuitable for use in cervical and cranial radiographic studies. Close attention to adequacy of the airway and spontaneous ventilation is of paramount importance when flexing the neck.
Intracranial masses and elevated ICP
Patients with intracranial masses, dysfunctional CBF autoregulation, and/or increased ICP are at risk of rapid decompensation under anesthesia. Preoperative assessment should include measurement of arterial blood pressure, because many patients with elevated ICP will have an accompanying increase in systemic arterial blood pressure (Cushing’s response). Systemic hypertension is an attempt to compensate and maintain adequate cerebral perfusion pressure. If MAP is significantly elevated prior to drug administration, arterial blood pressure should not be allowed to drop to normally acceptable levels under anesthesia (e.g., a MAP of 60 mm Hg), because cerebral ischemia can result despite an acceptable mean blood pressure.
Manual or mechanical positive pressure ventilation should be immediately available and instituted at induction. Mechanical ventilation should be continued until extubation. In some cases, allowing PaCO2 to rise in order to stimulate spontaneous ventilation, before the respiratory depressant effects of the anesthetics wane, may cause serious elevations in ICP and possibly herniation of the brain. Anesthetic monitoring should address the physiological variables associated with altered ICP. Venous and arterial blood pressures and airway or arterial sampling for carbon dioxide analysis should be included, if possible. Intravenous mannitol (0.5–1.0 g/kg IV slowly) may be useful to reduce ICP before, during, or after anesthesia. Optimal anesthetic management can substantially improve patient status and the outcome of intracranial surgical procedures.
Management of seizures in the perianesthetic period
CMR may be dramatically increased during seizure activity. Therefore, patients with neurological impairment should be critically evaluated to prevent drug-induced seizures during the perianesthetic period. Traditionally, drugs with side effects that may include seizure activity (e.g., ketamine, methohexital) are avoided. Chlorpromazine, when combined with intermittent light stimulation, has been associated with lowered seizure threshold in Beagle dogs.38 However, a retrospective study found no association between clinical administration of acepromazine in 36 dogs with seizure history and incidence of seizures.17 In addition, in dogs with active seizures, acepromazine was associated with decreased seizure activity or prevention of short-term recurrence. Another retrospective study of 31 dogs with a history of seizures found no correlation between acepromazine administration in a clinical setting and recurrence of seizure activity.39 Thus, there appears to be a relevant basis for judicious use of acepromazine in dogs with a seizure history.
Anesthesia for electrodiagnostic techniques
In veterinary medicine, clinical electrodiagnostic techniques are used to record potentials from muscle, peripheral nerves, spinal cord, brain stem, cortex, and retina. Even during those procedures in which the stimulus is innocuous, artifacts caused by movement may render the technique ineffective. Therefore, many of these procedures must be performed in anesthetized or tranquilized animals producing less stress while insuring a minimum of recording artifacts.
Electroencephalogram and bispectral index
Anesthetic effects on the electroencephalogram (EEG) depend on the anesthetic type and depth of anesthesia. Anesthesia initially causes an increase in the voltage and a decrease in the frequency of cortical potentials when compared with the record of an awake, alert dog. Spikes and spindles may also riddle the EEG of lightly anesthetized dogs and cats.40,41 As anesthesia deepens, the overall voltage begins to diminish. A dose–response decrease in cerebral oxygen consumption (CMRO2) accompanies the use of isoflurane in dogs and causes the EEG to become isoelectric at an end-expired concentration of 3%.42 The same type of cortical alteration in electrical activity, sometimes referred to as burst suppression, has been reported in swine but may occur in other species as well.43 Dose-dependent CNS depression of EEG activity by most anesthetics is characteristic and has led to the development of EEG-based anesthetic monitoring techniques.44
Notable exceptions to the general rule that anesthetics decrease EEG activity include the dissociative anesthetics and the volatile anesthetic enflurane. Further, alterations in normal PaCO2 are associated with significant changes in the quantitative EEG of dogs during halothane anesthesia.45 Monitoring CNS depression by using EEG during anesthesia has been the focus of study for decades. However, direct interpretation of the EEG has not proven adequately reliable or time responsive for use in operative circumstances. Processed EEG monitoring has evolved as a way to provide immediate feedback to anesthetists in regard to patients’ CNS activity. Spectral edge frequency, total power, beta/delta frequency ratios, and other specific parameters derived from computerprocessed EEG have been correlated to varying degrees with anesthetic depth, but interpretation can vary depending on the selection of anesthetic agents.
Intraoperative EEG processing has been developed with significant improvement in reliability. For example, the bispectral index (BIS) monitor is a proprietary device that provides information related to anesthetic depth and is readily interpreted. In addition, the BIS has proven to be reliable as an indicator of anesthetic depth in people given a variety of anesthetic agents and adjuncts.46,47 The BIS is a unitless number between 0 and 100 derived from the processed EEG.48 People undergoing surgery are typically maintained at a depth of anesthesia that yields a BIS value between 40 and 60, which reliably prevents intraoperative awareness.49–53
In animals, several reports have evaluated the use of BIS as an indicator of anesthetic depth. In dogs, isoflurane and sevoflurane anesthetic depth has been correlated to BIS. 54–56 Anesthetized cats had a nonlinear relationship of BIS with increasing multiples of sevoflurane minimum alveolar concentration.57 The change in BIS values following stimulation in isoflurane-anesthetized cats provided a useful measure of anesthetic depth.58 Use of BIS in veterinary medicine has been reviewed.59
Nerve-conduction studies
Nerve-conduction velocity has been successfully recorded in dogs while using a variety of anesthetic protocols. Because these procedures have not been done in unanesthetized animals, the effects of anesthetics on these procedures are largely unknown.
Evoked potentials may be altered by anesthetic agents, depending on the specific location of signal generators. Generally, cortical potentials are more likely to be affected than brain stem potentials. Various anesthetics and adjunctive drugs used in the perianesthetic period may affect latencies of evoked potentials. These effects appear to be specific to the particular drug and species.
Revised from “Neurological Disease” by Ralph C. Harvey, Stephen A. Greene, and William B. Thomas in Lumb and Jones’ Veterinary Anesthesia and Analgesia, Fourth Edition.
1. Shapiro H.H. Neurosurgical anesthesia and intracranial hypertension. In: Anesthesia, 2nd ed. R.D. Miller, ed. New York: Churchill Livingstone, 1986, pp 1563–1620.
2. Stoelting R.K. Pharmacology and Physiology in Anesthetic Practice. Philadelphia, PA: JB Lippincott, 1987.
3. van Poznak A. Special consideration for veterinary neuroanesthesia. In: Principles and Practice of Veterinary Anesthesia. C.E. Short, ed. Baltimore, MD: Williams and Wilkins, 1987, pp 177–183.
4. Hansen T.D., Warner D.S., Vust L.H., et al. Regional distribution of cerebral blood flow with halothane and isoflurane. Anesthesiology 69: 332–337, 1988.
5. Osborn I. Choice of neuroanesthetic technique. Anesthesiol Clin North America 5: 531–540, 1987.
6. Dayrell-Hart B., Klide A.M. Intracranial dysfunctions: stupor and coma. Vet Clin North Am Small Anim Pract 19: 1209–1222, 1989.
7. Grubb R.L., Raichle M.E., Eichling J.O., et al. The effects of changes in PaCO2 on cerebral blood volume, blood flow, and vascular mean transit time. Stroke 5: 530–539, 1974.
8. Cottrell J.E. Deliberate hypotension. Annual Refresher Course Lectures, American Society of Anesthesiologists, sect 245, 1989:1.
9. Harp J.R., Wollman H. Cerebral metabolic effects of hyperventilation and deliberate hypotension. Br J Anaesth 45: 256–262, 1973.
10. Frost E.A.M. Central nervous system trauma. Anesthesiol Clin North America 5: 565–585, 1987.
11. Hirshfeld A. Fluid and electrolyte management in neurosurgical patients. Anesthesiol Clin North America 5: 491–505, 1987.
12. Gilroy B.A. Neuroanesthesiology. In: Textbook of Small Animal Surgery. D. Slatter, ed. Philadelphia, PA: WB Saunders, 1985, pp 2643–2650.
13. Gray P.R., Lowrie C.T., Wetmore L.A. Effect of intravenous administration of dextrose or lactated Ringer’s solution on seizure development in dogs after cervical myelography with metrizamide. Am J Vet Res 48: 1600–1602, 1987.
14. Franklin R.T. The use of glucocorticoids in treating cerebral edema. Compend Contin Educ Pract Vet 6: 442–448, 1984.
15. Sato O., Hara M., Asai T., et al. The effect of dexamethasone phosphate on the production rate of cerebrospinal fluid in the spinal subarachnoid space of dogs. J Neurosurg 39: 480–484, 1973.
16. Gleed R.D. Tranquilizers and sedative. In: Principles and Practice of Veterinary Anesthesia. C.E. Short, ed. Baltimore, MD: Williams and Wilkins, 1987, pp 16–28.
17. Tobias K.M., Marioni-Henry K., Wagner R.A. A retrospective study on the use of acepromazine maleate in dogs with seizures. J Am Anim Hosp Assoc 42: 283–289, 2006.
18. Nugent M., Artru A.A., Michnfelder J.D. Cerebral metabolic, vascular and protective effects of midazolam maleate. Anesthesiology 56: 172–176, 1982.
19. Keegan R.D., Greene S.A., Bagley R.S., et al. Effects of medetomidine administration on intracranial pressure and cardiovascular variables of isoflurane-anesthetized dogs. Am J Vet Res 56: 193–198, 1995.
20. Karlsson B., Forsman M., Roald O., et al. Effect of dexmedetomidine, a selective and potent alpha 2-agonist, on cerebral blood flow and oxygen consumption during halothane anesthesia in dogs. Anesth Analg 71: 125–129, 1990.
21. Zornow M.H., Fleischer J.E., Scheller M.S., et al. Dexmedetomidine, an alpha-2 adrenergic agonist, decreases cerebral blood flow in the isoflurane-anesthetized dog. Anesth Analg 70: 624–630, 1990.
22. Marsh M.L., Marshall L.F., Shapiro H.M. Neurosurgical intensive care. Anesthesiology 47: 149–163, 1977.
23. Bonica J.J., ed. The Management of Pain, 2nd ed. Philadelphia, PA: Lea and Febiger, 1990.
24. Artru A.A., Katz R.A. Cerebral blood volume and CSF pressure following administration of ketamine in dogs: modification by pre-or posttreatment with hypocapnia or diazepam. J Neurosurg Anesthesiol 1: 8–15, 1989.
25. Strebel S., Kaufmann M., Maitre L., et al. Effects of ketamine on cerebral blood flow velocity in humans: influence of pretreatment with midazolam or esmolol. Anaesthesia 50: 223–228,1995.
26. Himmelseher S., Durieux M.E. Revising a dogma: ketamine for patients with neurological injury? Anesth Analg 101: 524–534, 2005.
27. Patel P.M., Drummond J.C. Cerebral physiology and the effects of anesthetic drugs. In: Miller’s Anesthesia, Vol. 1, 7th ed. R.D. Miller, ed. Philadelphia, PA: Churchill-Livingstone, 2009, pp 594–674.
28. Smith J.A. Hazards, safety and anesthetic considerations for MRI. Top Companion Anim Med 25(2): 98–106, 2010.
29. Chaffin M.K., Walker M.A., McArthur N.H., et al. Magnetic resonance imaging of the brain of normal neonatal foals. Vet Radiol Ultrasound 38: 102–111, 1997.
30. Lukasik V.M., Gillies R.J. Animal anaesthesia for in-vivo magnetic resonance. NMR Biomed 16: 459–467, 2003.
31. Willis C.K., Quinn R.P., McDonnell W.M., et al. Functional MRI as a tool to assess vision in dogs: the optimal anesthetic. Vet Ophthalmol 4: 243–253, 2001.
32. Young A.E., Brown P.N., Zorab J.S. Anaesthesia for children and infants undergoing magnetic resonance imaging: a prospective study. Eur J Anaesthesiol 13: 400–403, 1996.
33. Kross J., Drummond J.C. Successful use of a Fortec II vaporizer in the MRI suite: a case report with observations regarding magnetic field-induced vaporizer aberrancy. Can J Anaesth 38: 1065–1069, 1991.
34. Zimmer C., Janssen M.N., Treschan J.A., et al. Near-miss accident during magnetic resonance imaging by a “flying sevoflurane vaporizer” due to ferromagnetism undetectable by handheld magnet. Anesthesiology 100: 1329–1330, 2004.
35. Shelley K., Shelley S., Bell C. Monitoring in unusual environments. In: Clinical Monitoring. C.L. Lake, R.L. Hines, and C.D. Blitt, eds. Philadelphia, PA: WB Saunders, 2001, pp 524–538.
36. Wheeler S.J., Davies J.V. Iohexol myelography in the dog and cat: a series of one hundred cases and a comparison with metrizamide and iopamidol. J Small Anim Pract 26: 247–256, 1985.
37. Cox F.H. The use of iopamidol for myelography in dogs: a study of twenty-seven cases. J Small Anim Pract 27: 159–165, 1986.
38. Redman H.C., Wilson G.L., Hogan J.E. Effect of chlorpromazine combined with intermittent light stimulation on the electroencephalogram and clinical response of the Beagle dog. Am J Vet Res 34: 929–936, 1973.
39. McConnell J., Kirby R., Rudloff E. Administration of acepromazine maleate to 31 dogs with a history of seizures. J Vet Emerg Crit Care 17: 262–267, 2007.
40. Klemm W.R. Subjective and quantitative analyses of the electroencephalogram of anesthetized normal dogs: control data for clinical diagnosis. Am J Vet Res 29: 1267–1277, 1968.
41. Klemm W.R., Mallo G.L. Clinical electroencephalography in anesthetized small animals. J Am Vet Med Assoc 148: 1038–1042, 1976.
42. Newberg L.A., Milde J.H., Michenfelder J.D. The cerebral metabolic effects of isoflurane at and above concentrations that suppress cortical electrical activity. Anesthesiology 39: 23–28, 1983.
43. Rampil I.J., Weiskopf R.B., Brown J.G., et al. I653 and isoflurane produce similar dose-related changes in the electroencephalogram of pigs. Anesthesiology 69: 298–302, 1988.
44. Goodrich J.T. Electrophysiologic measurements: intraoperative evoked potential monitoring. Anesthesiol Clin North Am 5: 477–489, 1987.
45. Smith L.J., Greene S.A., Moore M.P., et al. Effects of altered arterial carbon dioxide tension on quantitative EEG in halothane-anesthetized dogs. Am J Vet Res 55: 467–471, 1994.
46. Sebel P.S., Lang E., Rampil I.J., et al. A multicenter study of bispectral electroencephalogram analysis for monitoring anesthetic effect. Anesth Analg 84: 891–899, 1997.
47. Takkallapalli R., Mehta M., DeLima L., et al. Bispectral index: can it predict arousal from noxious stimuli during general anesthesia? Anesth Analg 88(Suppl 2): S58, 1998.
48. Rampil I.J. A primer for EEG signal processing in anesthesia. Anesthesiology 89: 980–1002, 1998.
49. Katoh T., Suzuki A., Ikeda K. Electroencephalographic derivatives as a tool for predicting the depth of sedation and anesthesia induced by sevoflurane. Anesthesiology 88: 642–650, 1998.
50. Rosow C., Manberg P.J. Bispectal index monitoring. Anesthesiol Clin North America 16: 89–107, 1998.
51. Billard V., Gambus P.L., Chamoun N., et al. A comparison of spectral edge, delta power, and bispectral index as EEG measures of alfentanil, propofol, and midazolam drug effect. Clin Pharmacol Ther 61: 45–58, 1997.
52. Sleigh J., Andrzejowski J., Steyn-Ross A., et al. The bispectral index: a measure of depth of sleep? Anesth Analg 88: 659–661, 1999.
53. Kearse L.A., Rosow C., Zaslavsky A., et al. Bispectral analysis of the EEG predicts conscious processing of information during propofol sedation hypnosis. Anesthesiology 88: 25–34, 1998.
54. Greene S.A., Tranquilli W.J., Benson G.J., et al. Effect of medetomidine on bispectral index measurements in dogs during anesthesia with isoflurane. Am J Vet Res 64: 316–320, 2003.
55. Greene S.A., Benson G.J., Tranquilli W.J., et al. Relationship of canine bispectral index to multiples of sevoflurane minimal alveolar concentration, using patch or subdermal electrodes. Comp Med 52: 424–428, 2002.
56. Carrasco-Jimenez M.S., Cancho M.F.M., Lima J.R., et al. Relationship between a proprietary index, bispectral index, and hemodynamic variables as a means for evaluating depth of anesthesia in dogs anesthetized with sevoflurane. Am J Vet Res 65: 1128–1135, 2004.
57. Lamont L.A., Greene S.A., Grimm K.A., et al. Relationship of bispectral index to minimum alveolar concentration multiples of sevoflurane in cats. Am J Vet Res 65: 93–98, 2004.
58. March P.A., Muir W.W. Use of bispectral index as a monitor of anesthetic depth in cats anesthetized with isoflurane. Am J Vet Res 64: 1534–1541, 2003.
59. March P.A., Muir W.W. Bispectral analysis of the electroencephalogram: a review of its development and use in anesthesia. Vet Anaesth Analg 32: 241–255, 2005.
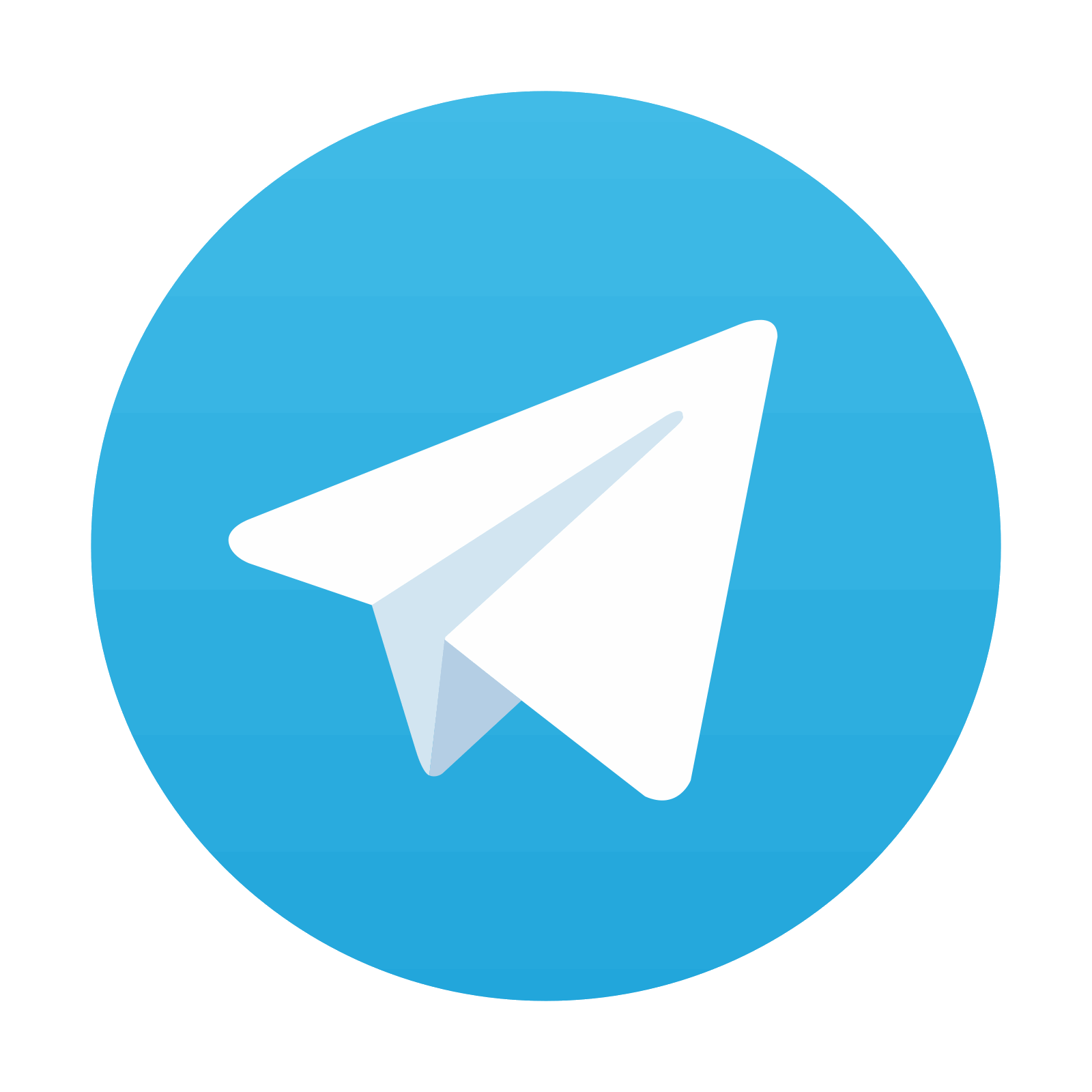
Stay updated, free articles. Join our Telegram channel
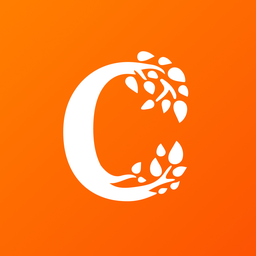
Full access? Get Clinical Tree
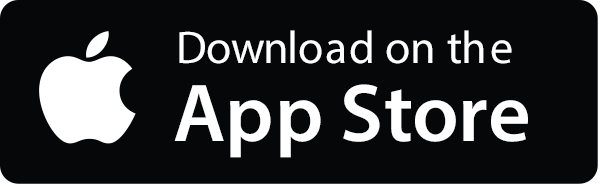
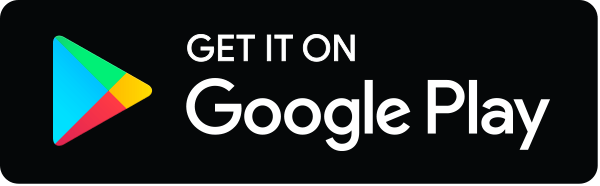