Craig A.E. Mosley VCA Canada, 404 Veterinary Emergency and Referral Hospital, Newmarket, Ontario, Canada The delivery and maintenance of safe anesthesia have become increasingly dependent upon mechanical and electrical equipment. It is necessary for the anesthetist to have a thorough understanding of equipment function and common failures as well as potential patient and personnel risks before use for routine patient care. Anesthesia equipment includes various airway support products, oxygen delivery devices, anesthetic machines, scavenge systems, ventilators, and many configurations of patient monitors and other support products. The products available to the veterinary anesthetist include nearly any human‐patient product that can be adapted for use in veterinary anesthesia, regularly produced items specifically for the veterinary market, and many limited‐production and/or custom products that may only be occasionally available. There are several excellent textbooks devoted to describing in great detail the anesthetic equipment available for use in human anesthesia [1–3] and, although not entirely applicable to veterinary anesthesia, much of the equipment used is the same (i.e., vaporizers, laryngoscopes, endotracheal tubes, some anesthetic machines) or is a modification of human products. As such, it would be impossible to discuss all of the anesthetic‐related equipment and products available today in a single chapter. More recently, a veterinary‐specific anesthetic equipment textbook has been published which details the anesthetic and monitoring equipment frequently used in veterinary medicine [4]. This chapter intends to provide the reader with the operating principles and a practical working overview of common anesthetic‐related products (i.e., endotracheal tubes, intubating aids, etc.), the anesthetic machine, vaporizers, breathing circuits, and ventilators. In addition, there are products that have been designed specifically for veterinary use, which are described in more detail here. Since at least 1976, human anesthetic breathing circuits (i.e., circle system) and anesthesia machines sold in North America must meet both mandated and voluntary regulations established by the United States Food and Drug Administration (FDA) (Medical Device Amendments‐1976, Good Manufacturing Practices‐1978, and Mandatory Device reporting‐1984) and organizations such as the International Organization for Standardization (ISO 5358‐198a), American Society for Testing and Materials (ASTM F1850), and the Canadian Standards Association (CSA). Many of these standards are currently under re‐review with updates anticipated. Anesthetic machines designed for veterinary use are not required to meet any specific design or safety standards beyond those associated with basic hazards to the operator (i.e., electrical safety requirements). Safety features are often added on an ad hoc basis and there are no requirements for demonstrating equipment efficacy. Ideally, some safety features, such as airway pressure alarms, should be integral to the design of the anesthetic machine. The inclusion of some of these safety systems on anesthetic machines may help eliminate preventable anesthetic accidents. However, until safety and design standards are adopted by the manufacturers of veterinary anesthetic equipment, there will remain equipment options of varying quality, efficacy, and safety available for delivering inhalant anesthetics to veterinary patients. Ancillary and support equipment for veterinary patients, including patient monitors and ventilators, are similarly devoid of required efficacy and safety testing. Fortunately, most reputable manufacturers and distributors readily provide the specifications, accuracy, and any available efficacy data for their designs. Regardless of the presence of standards, it will always be incumbent upon the veterinary anesthetist to understand thoroughly the function, principles of operation, and use of all anesthetic‐related pieces of equipment and to ensure that the machine or piece of equipment is designed suitably well to accomplish its functions safely. Airway management and support are vital for the safe delivery of anesthesia. Most, if not all, anesthetics cause respiratory depression at doses suitable for anesthesia. In addition, relaxation and/or loss of airway reflexes make the patient more prone to upper airway obstruction. Both of these factors put the anesthetized patient at higher risk for the development of hypoxia. Additionally, the inhalant anesthetics require delivery to the lungs while minimizing environmental and personnel exposure to waste anesthetic gases. For these reasons, airway management and support are critical aspects of properly performed inhalant general anesthesia. Endotracheal tubes are commonly used to maintain an airway in anesthetized patients. Supraglottic airway devices (SGADs) have also been evaluated in a number of domestic species and may be suitable alternatives in some instances [5–9]. A properly placed endotracheal tube or SGAD with a properly inflated cuff provides a patent airway, facilitates positive‐pressure ventilation, protects the lungs from aspiration of fluids, and prevents contamination of the work environment with waste anesthetic gases. Occasionally, it is desirable to limit airway management to one lung field (i.e., thoracoscopy), and specially designed equipment is available to accomplish this task in dogs. There are many styles and types of endotracheal tubes available that can be used in veterinary medicine. Most are manufactured for humans but can also be used in most small animal patients. There are some veterinary‐specific products available for patients requiring tube sizes larger and smaller than those available for human use. Endotracheal tubes manufactured for human patients must have various markings and abbreviations directly on the tube describing the tube’s characteristics as well as interval markings to measure the depth of insertion. The markings may include the manufacturer, internal diameter (ID), outer diameter (OD), and length of the tube, as well as codes indicating tissue toxicity or implantation testing (e.g., F29) (Fig. 6.1). There is no requirement for similar markings on tubes manufactured solely for veterinary use, but it is common for them to minimally list tube diameters and lengths. Endotracheal tubes are often sized according to their internal diameters. For example, a size 6.0 endotracheal tube refers to a tube with an internal diameter of 6 mm. Some tubes manufactured specifically for the veterinary market have their sizes indicated using the French gauge/catheter scale, and this should, but may not always, reflect the internal size of the tube. The outer diameter for any given tube size may vary depending upon the construction of the tube. Endotracheal tubes having thicker walls will have greater differences between the internal and outer diameters. This can become important when selecting tubes for very small patients. Very thick‐walled tubes will effectively reduce the internal airway diameter compared with a thin‐walled tube as ultimately, the size of the endotracheal tube that can be placed in a patient is limited by the outer diameter of the tube and not the inner diameter. However, very thin‐walled soft tubes are susceptible to obstruction by external compression or kinking (Fig. 6.2). Common endotracheal tube materials include polyvinyl chloride, silicone, or red rubber. Clear endotracheal tubes are generally preferred so that they can be inspected visually for the presence of mucus or blood intraoperatively, or debris within the tube lumen after cleaning. Generally, the largest size of an endotracheal tube that will fit without causing trauma in the patient’s trachea should be used. Although various “rules‐of‐thumb” for selecting tube size exist, it is probably easiest to estimate the most appropriate tube size by palpating the individual patient’s cervical trachea. The tube should not extend distally beyond the thoracic inlet and ideally should not extend rostrally beyond the patient’s incisors, as any additional tube length extending beyond the patient’s incisors will increase mechanical deadspace. If the endotracheal tube is too long, and further insertion would lead to the possibility of endobronchial intubation, the machine end can be cut, and the endotracheal tube connector reinserted. Figure 6.1 A. Most endotracheal tubes have common design features as shown here; however, the specific design and materials can vary among the various manufacturers. B. Tubes can be made of silicone, polyvinyl chloride, and red rubber (top to bottom). Source: Dr. Craig Mosley, with permission. Figure 6.2 Very thin‐walled endotracheal tubes are prone to occlusion from external compression or twisting. Continual evaluation of the endotracheal tube for patency is required when thin pliable walled endotracheal tubes are used. Source: Dr. Craig Mosley, with permission. The most commonly used type of endotracheal tube in both large and small animals is the cuffed Murphy‐type tube shown in Fig. 6.1. Cole‐type and guarded (spiral embedded, armored) tubes are also occasionally used in veterinary medicine. Cole tubes are uncuffed and have a smaller diameter at the patient (distal) end relative to the machine (proximal) end. The distal smaller diameter portion of the tube is inserted into the trachea to a point where the shoulder contacts the larynx, forming a seal. However, Cole tubes will not produce the same degree of airway security as a standard cuffed tube and are normally used only in very small patients for short‐term intubation (see Fig. 6.3). Guarded tubes incorporate a metal or nylon spiral‐wound reinforcing wire into the endotracheal tube wall that helps prevent tube collapse and occlusion (Fig. 6.4). Guarded tubes are useful in situations where the tube is likely to be compressed or kinked, such as procedures requiring extreme flexion of the head and neck (e.g., cervical cerebrospinal fluid collection and ophthalmic procedures) or those that involve compression of the trachea (e.g., tracheal retraction during the ventral approach to the cervical spinal cord). The machine end of the tube contains the endotracheal tube connector. The most proximal portion of the connector used for small animals and human patients is a uniform size (15 mm OD) facilitating universal connection to all standard anesthetic circuits. Tubes designed for use in large animals typically have larger connectors, which include metal‐type and funnel‐type connectors. The distal (patient) end of the connector varies in size according to the diameter of the endotracheal tube. Endotracheal tube adapters may also incorporate gas sampling ports (Fig. 6.5). These are particularly useful in small patients where minimizing equipment deadspace can be important and may improve the accuracy of gas sampling in smaller patients where non‐rebreathing systems are often used. Figure 6.3 Cole endotracheal tube demonstrating the tapered shoulder used to position the tube in the larynx forming a seal. Note that the tube does not have a cuff or pilot balloon. Figure 6.4 A. Guarded endotracheal tube with a metal or nylon spiral reinforcing wire. B. This spiral wire prevents collapse if the tube is bent or folded. Source: Dr. Craig Mosley, with permission. Figure 6.5 Two endotracheal tube adapters that incorporate a gas sampling port. Note the internal diameter (volume) of the pediatric design on the right. This type of design can help improve the accuracy of side‐stream end‐tidal gas sampling in smaller patients. Source: Dr. Craig Mosley, with permission. Endotracheal tubes designed for large animals are normally manufactured with a silicone funnel adapter attached (Fig. 6.6) that is designed to fit over the large animal Y‐piece (54 mm OD). There are also stainless‐steel Bivona endotracheal tube adapters (22 OD) that are designed to fit the Bivona insert sometimes found on large animal Y‐pieces. The patient (distal) end of the endotracheal tube is normally beveled. Murphy‐type tubes have a hole in the endotracheal tube wall opposite the bevel, referred to as a “Murphy eye” or “Murphy hole” (see Fig. 6.1A). The purpose of the hole is to provide an alternative route for gas flow should the beveled opening become occluded. Endotracheal tubes that lack a Murphy eye are referred to as Magill‐type tubes. Most endotracheal tube sizes can be found without an inflatable cuff, although the use of cuffed tubes provides a more reliable airway. Tubes lacking cuffs tend to be very small diameter tubes where the addition of a cuff may not be possible or will limit the maximum diameter tube that can be used in a patient. The cuff is located on the machine‐end side of the Murphy eye for cuffed tubes, and can be a low‐volume, high‐pressure or high‐volume, low‐pressure design (Fig. 6.7). In general, high‐volume, low‐pressure cuffs are preferred to minimize the risk of ischemic tracheal injury that may result from excessive cuff pressure against the tracheal wall. When a properly fitting endotracheal tube with a high‐volume, low‐pressure cuff is used, the pressure exerted by the cuff on the tracheal wall is similar to the intracuff pressure. This allows for a better estimation of the pressure on the tracheal wall exerted by the cuff. When using a low‐volume, high‐pressure cuffed endotracheal tube, the intracuff pressure does not reflect the pressure on the tracheal wall but rather the pressure created by the elastic recoil of the cuff, making estimates of pressure exerted by the cuff on the tracheal wall difficult. Tracheal wall pressures exceeding 48 cmH2O may impede capillary blood flow, potentially causing ischemic tracheal damage, and pressure below 18 mmHg may increase the risk of aspiration [10]. There are also several cases of tracheal rupture or disruption reported in veterinary medicine leading to pneumothorax, pneumomediastinum, and/or subcutaneous emphysema [11]. A reliable method for ensuring that cuff pressures are within the recommended range is to use a cuff monitor to inflate high‐volume, low‐pressure cuffs. A cuff monitor is essentially a low‐pressure manometer similar to those used for Doppler blood pressure measurement that is attached to the pilot balloon of the cuff and provides a measure of intracuff pressure. As noted above, these devices are only useful when using appropriately sized high‐volume, low‐pressure cuffs. If high‐pressure cuffs are used or if the endotracheal tube is markedly undersized for the patient, the measured cuff pressure may not accurately reflect the pressure exerted on the tracheal wall. Other commercially available cuff inflation guides are available for the human market and can be adapted to veterinary use (Fig. 6.8). Alternatively, it is more common to use a leak test or the minimal occlusive volume (MOV) technique, performed by inflating the cuff until a leak is no longer audible while maintaining airway pressures of 20–30 cmH2O. Recently two commercially available syringe devices specifically designed for endotracheal tube cuff inflation have been evaluated for use in veterinary medicine that may help reduce the incidence of improperly inflated cuffs compared to MOV [12]. The pilot balloon used for inflating the endotracheal tube cuff is connected to the cuff via a channel incorporated into the endotracheal tube and normally includes a syringe‐activated self‐sealing valve system. However, there are also valveless pilot balloons that do not self‐seal and require occlusion using either a clamp or plug. Figure 6.6 Two endotracheal tubes used for large animal anesthesia. These tubes are typically silicone and are commonly manufactured with a silicone funnel adapter that is compatible with the Y‐piece of most large animal anesthetic breathing circuits. Source: Dr. Craig Mosley, with permission. Figure 6.7 The upper endotracheal tube has a high‐volume, low‐pressure cuff, whereas the lower endotracheal tube shows an example of a low‐volume, high‐pressure cuff. Note the bulkiness that can be associated with the high‐volume, low‐pressure cuff compared with the low‐volume, high‐pressure cuff. The bulkiness associated with some cuffs can limit the endotracheal tube size, which can be problematic in very small patients. However, high‐volume, low‐pressure cuffs may help reduce tracheal damage resulting from cuff overinflation. Source: Dr. Craig Mosley, with permission. An uncuffed self‐sealing endotracheal tube (Safe‐Seal™, Innovative Animal Products, Rochester, MN, USA, 55901) has been introduced into the veterinary market. The tube is designed with a series of flexible circumferential flanges at the patient end of the endotracheal tube that deform to the contours of the trachea, forming a seal against the tracheal wall eliminating the need to inflate a cuff (Fig. 6.9). There are currently no independent research studies evaluating the effectiveness of this tube for use in veterinary anesthesia. It is available with only a limited number of internal tube diameters and differs from a conventional endotracheal tube in that it has no Murphy eye or inflatable cuff. Figure 6.8 Examples of endotracheal tube inflation guides or pressure monitoring devices. These devices can be used to evaluate intracuff pressures and may help avoid tracheal injury secondary to excessive tracheal wall pressures. Several styles are available. Source: Dr. Craig Mosley, with permission. In addition to the endotracheal tubes described above, there are tubes specially designed for isolating or ventilating one lung. Indications for the use of these tubes include improving surgical conditions for various thoracic procedures (i.e., thoracoscopy), the control of contamination or hemorrhage, and use in circumstances where unilateral pathology exists. There are generally three methods of isolating or ventilating a single lung: a double‐lumen tube (DLT), a bronchial blocker, or by using a long, standard endotracheal tube as an endobronchial tube. Endobronchial intubation for single lung ventilation or isolation is probably the least desirable option as it provides less direct control for making changes in the non‐intubated lung. However, it does not require specialized equipment, apart from a sufficiently long endotracheal tube, and is relatively easy to perform. Endobronchial intubation has been used in dogs successfully and may be an alternative when DLTs and bronchial blockers are not available [13,14]. DLTs tend to be the preferred option in human medicine. All commercially available DLTs have been designed specifically for human patients and have been adapted for use in dogs. There have been several types evaluated in a range of dog sizes and breeds [15–18]. DLTs consist of two single‐lumen tubes bonded together and are available as right‐ or left‐sided tubes (Fig. 6.10), where right and left designate which mainstem bronchus the tube is designed to fit. Most DLTs are designed with an angled distal tip to facilitate placement into either the right or left bronchus. The three most commonly available styles of DLTs are the Robertshaw, Carlens, and White. The tubes have two elliptical cuffs: one occludes the trachea and the other occludes the bronchus (Fig. 6.11). The bronchial cuff and pilot balloon are normally colored blue for differentiation from the tracheal cuff. Figure 6.9 A. Example of a self‐sealing endotracheal tube that lacks an inflatable cuff and instead relies on a series of soft flexible flanges to provide airway security. B. Lateral radiograph illustrating placement of a self‐sealing endotracheal tube. Source: Dr. Craig Mosley, with permission. Figure 6.10 An example of a left‐sided Robertshaw double‐lumen endotracheal tube. DLTs can be used to ventilate selectively one or both lung fields in appropriately sized dogs. Source: Dr. Craig Mosley, with permission. The bronchial cuffs of right‐sided tubes vary in shape and design in order to facilitate ventilation of the upper right lung lobe in humans. The use of right‐sided tubes can introduce greater placement uncertainty and failures when used in dogs as the right cranial lung lobe bronchus of the dog branches more proximally than in humans; consequently, the bronchial cuff may occlude the bronchus or failure to completely isolate the hemithorax may occur. In general, left‐sided tubes are most often used and can be used effectively for both right‐ and left‐sided procedures. Even if proximal clamping or transection of the left mainstem bronchus is required, the left‐sided tube can simply be withdrawn into the trachea so the distal bronchial portion of the tube does not interfere with clamping. The internal lumen of the tracheal portion of the tube is oval or D‐shaped, and the sizes are designated using the French scale, ranging from 26 to 41 Fr. The reduced lumen size will increase resistance to breathing compared to an appropriately sized standard single lumen tube, but this is overcome by the frequent use of intermittent positive‐pressure ventilation (IPPV) in these cases. DLTs allow the anesthetist to ventilate each lung field independently of the other or both lung fields together without replacing or moving the tube, but it does require disconnection and reconnection of the anesthetic circuit to the appropriate endotracheal tube adapter (bronchial or tracheal) or both by using a Y‐piece adapter (Fig. 6.12). The ability to ventilate selectively either or both lung fields is a distinct advantage over bronchial blocking systems or endobronchial intubation when surgical conditions require operating on both sides of the chest. However, the available sizes typically limit the use of DLTs to dogs between 5 and 20 kg. Some DLTs (Carlens and White) also incorporate a carinal hook designed to aid in the proper placement of the tube and prevent movement after positioning. In veterinary patients, this modification may actually hinder rather than facilitate correct tube placement [15]. Additional care should also be taken when placing DLTs with a carinal hook to ensure that it does not catch on any tissues or structures when introducing the tube into the trachea. For this reason, the Robertshaw left‐sided DLT is probably the most versatile type for use in dogs. In veterinary patients, the correct placement of a DLT is generally confirmed by direct visualization using a small‐diameter bronchoscope. A thoracoscopic‐assisted technique has also been described [15]. Correct and complete placement is further confirmed by ventilating both the right and left lung fields while auscultating for lung sounds. Correct and complete placement should ventilate all intended lung fields (i.e., right or left) without ventilating any lung fields on the contralateral side. Although blind placement is possible using some tubes, it is associated with a relatively high failure to achieve correct and complete tube placement [15]. Small movements of the tube and/or the patient may disrupt correct and complete placement and occasionally bronchial cuffs will prolapse into the trachea, leading to complete airway obstruction. Vigilance is required by the anesthetist to recognize and correct any positional problems should they occur (e.g., deflation of bronchial cuff). Figure 6.11 A closer image demonstrating the distal end of a left‐sided Robertshaw double‐lumen endotracheal tube. Note the angle of the distal end of the tube and the two cuffs. The more distal blue cuff is the bronchial cuff. This tube is designed to facilitate placement into the left mainstem bronchus. Source: Dr. Craig Mosley, with permission. Bronchial blockers represent another system for facilitating one‐lung isolation or ventilation in dogs (Fig. 6.13) [14,16,19–22]. In human anesthesia, they are often used when patient size precludes the use of a DLT or when anatomic abnormalities or differences are present that may preclude optimal tube fit. The fact that they are very adaptable for use over a wider range of patient sizes and are not as anatomically specific as DLTs are distinct advantages of using bronchial blockers in veterinary anesthesia. However, independent lung ventilation is not possible without withdrawing and replacing the bronchial blocker in the contralateral bronchus. Figure 6.12 Double‐lumen tubes (DLTs) can be used to ventilate (or collapse) each lung field independently or can ventilate both lung fields simultaneously. A. Adapter configuration required for independent lung field ventilation (or collapse). B. Use of a Y‐adapter to facilitate simultaneous ventilation of both lung fields. Note the blue pilot balloon cuff and the blue tubing corresponding to the bronchial portion of the tube. Source: Dr. Craig Mosley, with permission. Figure 6.13 An example of a bronchial blocking system commonly used in veterinary medicine. The system consists of a bronchial blocker (balloon‐tipped catheter) and a swivel adapter allowing for coaxial placement of the blocker. Source: Dr. Craig Mosley, with permission. Figure 6.14 The yellow (or outermost) catheter with the obvious pilot balloon is specifically designed for use as a bronchial blocker. The inner shorter catheter is a balloon‐tipped Foley catheter. Although Foley catheters are not designed for use as bronchial blockers, they have been placed alongside endotracheal tubes and used successfully for bronchial blockade, but they are not as simple to use or place as a purpose‐designed bronchial blocker. Source: Dr. Craig Mosley, with permission. Bronchial blockers are essentially long catheters, tipped with an elliptical or round inflatable cuff or balloon (Fig. 6.14). The cuffs and pilot balloons of most purpose‐designed bronchial blockers are blue to differentiate them from those of the endotracheal tube. Foley or balloon‐type catheters used as bronchial blockers obviously do not follow this color scheme. Bronchial blockers can be used coaxially or in parallel with a standard endotracheal tube. Various swivel adapters are supplied with commercially available bronchial blockers to facilitate coaxial use. The swivel adapters connect to the endotracheal tube adapter and have ports for passing the bronchial blocker, a bronchoscope, and a connector for the anesthetic circuit (Fig. 6.15). The ports are designed in a way to prevent leakage and to secure the bronchial blocker once it is in place. There is also a commercially available human coaxial product (Univent® tube, Teleflex Inc., Limerick, PA, USA) that incorporates the bronchial blocker in a channel running in the lumen of the endotracheal tube. However, the length of the bronchial blocker is limited and may not be sufficiently long for larger patients. A balloon catheter (Fogarty or Foley catheter) can also be used as a bronchial blocker placed in parallel with the endotracheal tube or coaxially with a custom adapter solution (see Fig. 6.14). Bronchial blockers require fiber‐optic‐assisted direct visualization for correct placement. One of the unique features of bronchial blockers over DLTs is that they can be used to isolate a single lung lobe in addition to the entire hemithorax. The bronchial blocker can be directed into the bronchus to be blocked by directly manipulating the proximal portion of the bronchial blocker or by placing a guide wire into the bronchus to be blocked and sliding the bronchial blocker over the guide wire. One bronchial blocker (Ardnt Endobronchial Blocker™, Cook Medical, Bloomington, IN, USA) has a small wire loop exiting the distal end of the blocker (Fig. 6.16). The loop can be used to facilitate placement by sliding the loop over the end of the bronchoscope. Once correct placement has been achieved, the balloon or cuff can be inflated, precluding ventilation of that lung region. The lung is then allowed to collapse by opening the bronchial blocker catheter channel. The open channel can be used for continuous positive airway pressure (CPAP) application, oxygen insufflation, and/or suctioning. The placement of the bronchial blocker in the right bronchus can be challenging owing to the proximal branching of the cranial lung lobe. Prolapse of a bronchial blocker into the trachea can lead to complete airway obstruction; this is most likely to occur when placed proximally in the bronchus and/or if the tube and bronchial blocker assembly are withdrawn inadvertently when moving or manipulating the patient. Figure 6.15 An example of the airway adapter supplied with the Arndt Endobronchial Blocker™ (Cook Medical, Bloomington, IN, USA). The adapter is placed between the endotracheal tube adapter and the breathing circuit. The port to the right attaches to the endotracheal tube adapter; the ports moving clockwise include the bronchial port (with an annular compression fitting), a bronchoscope port, and the patient circuit port. Source: Dr. Craig Mosley, with permission. Figure 6.16 The distal end of an Arndt Endobronchial Blocker™ (Cook Medical, Bloomington, IN, USA) showing the wire loop used to facilitate proper placement using a bronchoscope. Source: Dr. Craig Mosley, with permission. Another bronchial blocker (Rüsch® EZ‐Blocker® endobronchial blocker, Teleflex Inc., Limerick, PA, USA) consists of paired of “bonded” blockers in a single tube that bifurcate at the distal tip (Fig 6.17). This design can ease placement by allowing the bifurcation to more intuitively straddle the carina with a blocker tip in both the right and left mainstem bronchus allowing for right or left blockade. However, the EZ‐Blocker® cannot be used to isolate single lung lobes and, similar to other techniques, it remains difficult to collapse the right hemithorax due to the proximal branching of the right cranial lung lobe [21,22]. Figure 6.17 A. The Rüsch® EZ‐Blocker® is a dual‐lumen endobronchial blocker. B. Proximal end showing two inflatable cuffs/pilot balloons. C. Integrated distal bifurcation that can conveniently straddle the patient’s carina when placed. The system comes with a multiport adapter that connects between the patient endotracheal tube and anesthetic breathing circuit. Source: Dr. Craig Mosley, with permission. Supraglottic airway devices (SGADs), also commonly referred to as “laryngeal mask airways” (LMAs), are becoming increasingly popular for veterinary use. There are a large number of products available that were designed for humans that have been adapted for veterinary use. However, these products have been optimized specifically for the orolaryngeal/pharyngeal anatomy of humans and may not conform well to the varied anatomy, patient size, species, and breeds commonly encountered in veterinary medicine. Appropriate use of these products is important as inappropriate SGAD and/or patient selection may lead to placement difficulties/failures, damage to tissues of the oropharyngeal region, and/or improper protection and patency of the airway. There are several veterinary‐specific SGADs that have been introduced (dog, cat, and rabbit v‐gel®, Docsinnovent Ltd, London, UK) designed for use in dogs, cats, and rabbits (Fig. 6.18). Figure 6.18 Veterinary‐specific laryngeal masks (v‐gel®) designed for use in A. cats and B. rabbits. These masks can be used as alternatives to tracheal intubation and may be easier to place than endotracheal tubes in some species. Source: Docsinnovent Ltd, London, UK; reproduced with permission. SGADs represent an alternative to endotracheal intubation for maintenance of a patent airway and there is some evidence that they may be simpler and faster to place than endotracheal tubes in some species [5,23,24]. There is also some evidence that less anesthetic is required for the placement of an SGAD compared with an endotracheal tube [25]. SGADs do not require the use of a laryngoscope for placement and do not enter the larynx or trachea. A typical device consists of a tube, similar to an endotracheal tube, connected to an elliptical mask that has an inflatable outer edge. When placed and inflated correctly, they form a seal around the glottis. The criteria for correct SGAD placement have been described for various species and should be reviewed prior to using these devices. Properly placed and inflated SGADs are not associated with greater leakage of anesthetic gases compared with endotracheal tubes and positive‐pressure ventilation using an SGAD has been successfully performed and evaluated in several veterinary species [5–9,26–28]. The use of SGADs is relatively uncommon in veterinary medicine but as their use increases and further large prospective studies are completed, additional advantages and disadvantages related to relatively rare events (e.g., gastroesophageal reflux and subsequent aspiration, significant post‐extubation airway irritation) may be detected. Laryngoscopes consist of a handle and lighted blade and are used to aid tracheal intubation and oropharyngeal evaluation during intubation. Unfortunately, laryngoscopes are often considered an optional piece of anesthetic‐related equipment, but their proper use can be vital for successful intubation in some patients (e.g., brachycephalics and patients with laryngeal/oral trauma). Regardless of the absolute need for laryngoscope‐assisted intubation, their use is recommended for all intubations to ensure that the anesthetist maintains the motor skills and coordination to use a laryngoscope properly while permitting a cursory oropharyngeal evaluation. There are several styles and types of laryngoscopes and blades available. Some laryngoscopes have a fixed blade (i.e., one blade type and size) and may be made of plastic, whereas others are designed for use with multiple blade sizes and styles of blades and are made of stainless steel. Because there is such a range of patient sizes and different oral cavity configurations in veterinary medicine, the option to use multiple blades is a significant advantage when selecting a laryngoscope. The handle may also vary in size and, although this rarely impacts the functional use of the laryngoscope, a smaller handle may be more comfortable and easier to manipulate for some anesthetists, particularly when used for intubating very small patients. The handles are usually specific for either fiber‐optic or bulb‐in‐blade illumination, although there are some handles that can accept either type of blade illumination system. There is no clear advantage of one lighting system over the other. There are two main types of blades that are used in veterinary medicine, the MacIntosh and the Miller blade. Both come in a wide range of sizes (000–5). The MacIntosh is a curved blade with a prominent vertical flange, whereas the Miller is a straight blade with a less prominent vertical flange; both are suitable for intubation of most patients and the decision to use one over the other is often determined by personal preference (Fig. 6.19). However, the prominent flange of the MacIntosh blade can potentially interfere with laryngeal visualization when used for intubating veterinary patients (see below). In addition to the standard‐sized blades available in human medicine, extremely long (~300 mm) Miller‐style blades (useful for intubating swine, camelids, sheep, and goats) are also available. Interestingly, the majority of human‐designed laryngoscope blades and endotracheal tubes are designed for the anesthetist to use their right hand to pass the endotracheal tube while their left hand holds the laryngoscope. The endotracheal tube bevel faces the left when viewing the tube from the concave aspect and the laryngoscope blade flange is normally on the right side of the blade when viewing the blade from the top. This configuration provides optimal visualization of the larynx when intubating a patient in the supine position (dorsal recumbency) where the laryngoscope is held with the blade in a downward position (inverted) and the endotracheal tube is held with the concave surface directed upwards. However, most veterinary patients are intubated in sternal recumbency where the flange of the laryngoscope, when held in the left hand, can obscure visualization and the bevel of the endotracheal tube will do little to improve visualization when held in the right hand. There are left‐handed MacIntosh blades available that may be more appropriate for routine intubation in veterinary species as these blades place the flange on the left side of the blade, improving visualization of the laryngeal area when the laryngoscope is held in the left hand in an upright position. Since the Miller blade’s flange is far less prominent, there is no real need for a left‐handed design. Figure 6.19 Laryngoscope handle with Miller (upper) and Macintosh (lower) blades. Note the more prominent vertical flange on the MacIntosh blade. This flange may impair visualization of the larynx when intubating a patient in sternal recumbency using the right hand. Source: Dr. Craig Mosley, with permission. Orotracheal intubation of most veterinary patients does not require any special equipment beyond the use of a laryngoscope and a familiarity with normal patient anatomy. However, there are circumstances and situations resulting from anatomic features, pathology, or trauma that make oral endotracheal intubation difficult or impossible. It is important that the veterinary anesthetist be familiar with and prepared to use alternative techniques to obtain a patent airway. The following sections present some of the options available, the associated equipment required, and descriptions of the techniques in general terms. For more specific details, the reader should consult species‐specific chapters in this book or other species‐specific veterinary anesthesia references [29]. Nasotracheal intubation is a useful technique for procedures involving the oral cavity where a standard orotracheal tube may preclude or limit optimal surgical or diagnostic access or it can be used for procedures in sedated conscious animals that will not tolerate an orotracheal tube but require oxygen supplementation and support. Nasotracheal intubation can also be safely used for the administration of inhalant anesthetics for inducing anesthesia in some animals (foals and calves) [30,31]. The technique for nasotracheal intubation has been described in foals, calves, horses, camelids, rabbits, and a kangaroo but has certainly been performed and not reported in many other animal species [30–34]. The characteristics of an ideal nasotracheal tube include a tube with minimal curvature and a suitable length to extend distally beyond the larynx. The tube should be made of inert material (e.g., silicone rubber) and have relatively thin walls for maximum internal diameter, although this may increase the risk of tube compression or kinking. Low‐volume, high‐pressure cuffs are typically less bulky and may be less traumatic during placement, but high‐volume, low‐pressure cuffs may be best for longer periods of anesthesia. Tube size will be dependent upon species and patient size but will often be smaller than an appropriately sized orotracheal tube. The smaller internal diameter can increase resistance to gas flow and may be a problem for some spontaneously breathing patients. Nasotracheal intubation involves passage of a properly sized endotracheal tube through the nostril, nasal meatus, and larynx, and into the trachea. Lidocaine‐containing gel can facilitate placement and provide lubrication for the tube. It should be applied to the nostril and rostral portion of the nasal passage before advancing the tube in awake or sedated animals. A sterile water‐soluble lubricant without lidocaine is appropriate for anesthetized patients. The tube should be passed gently and may require some rotation and redirection in order to facilitate passage between nasal conchae. Nasal hemorrhage or other tissue damage occasionally occurs during this procedure, particularly if excessive force or an excessively large tube is used relative to the nasal passages or if the tube is passed into the incorrect nasal meatus. In general, the patient’s head and neck should be extended to facilitate passage of the tube from the nasopharynx into the trachea. However, species differences may necessitate alternative positioning or further manipulation of the head, neck, and laryngeal positions. Air should move freely through a correctly placed tube during spontaneous ventilation. Confirmation of correct tube placement can be readily, and initially, assessed by using a bulb syringe adapted to the end of the nasotracheal tube. Once the tube is in place, the bulb can be squeezed and attached to the tube; if it readily expands, this suggests the tube is an airway, and if the bulb fails to expand, it is likely that the tube has entered the esophagus (Fig. 6.20). Further confirmation of correct tube placement using auscultation of lung sounds during manual ventilation and/or capnography waveform appearance should be used for definitive confirmation. Extubation following nasotracheal intubation should be done carefully. After deflation of the cuff, the tube should be withdrawn slowly and deliberately, with the patient’s head restrained to avoid any sudden, jerky motions. Rapid, rough extubation may cause nasal hemorrhage. Wire‐ or tube‐guided techniques are sometimes used when direct visualization of the laryngeal opening is not possible or obscured. This is commonly a result of species‐specific anatomic features (e.g., rabbits and brachycephalic breeds) and patient size relative to available equipment. For example, the laryngoscope may be too small to be effective in a cow, and manual palpation and guide tube placement using a small‐diameter tube is frequently used to facilitate passage of the desired endotracheal tube; in very small patients, the presence of both the laryngoscope and the endotracheal tube may obscure visualization and a thin wire can be initially placed to facilitate intubation [35]. In some patients, trauma or other pathological conditions (nasopharyngeal polyp or mass) may obscure the laryngeal opening with only a small portion visible. In these circumstances, the placement of a small‐diameter tube or a wire can be used as a guide for placement of a properly sized endotracheal tube (Fig. 6.21). The technique involves using a guide (wire or tube) with a smaller outer diameter than the internal diameter of the intended endotracheal tube. It should also be sufficiently long to allow complete intubation (about half the distance from the cricoid cartilage to the thoracic inlet) while still allowing the tube to be placed over the guide leaving a portion of the guide available for the operator to hold while advancing the tube (Fig. 6.22). The tube or wire should be blunt‐ended so as not to damage the trachea or associated structures. The wire guides associated with some intravenous catheters (e.g., multiple‐lumen jugular catheters) are blunt‐ended and make excellent wire guides for some small patients. The guide is first appropriately placed and then the endotracheal tube is fed over the guide and through the laryngeal opening blindly; occasionally, slight rotation of the tube is required, and passing the tube through the larynx during inspiration when the arytenoids are fully abducted helps facilitate smooth passage. The guide is then removed, and the tube secured into place. Figure 6.20 A suction bulb adapted to the end of an endotracheal tube can be used for the rapid assessment of correct endotracheal intubation. The bulb is deflated and attached to the tube using an appropriately sized adapter. If the endotracheal tube is in the trachea, the bulb should immediately reinflate. If the endotracheal tube is in the esophagus, the bulb will not generally reinflate. This technique provides a rapid assessment of proper intubation when direct visualization is not possible (i.e., nasotracheal intubation) but should always be used in conjunction with additional methods of confirming proper endotracheal intubation (i.e., observation of capnography waveform or auscultation of lung sounds during manual ventilation). Source: Dr. Thomas Riebold, College of Veterinary Medicine, Oregon State University, Corvallis, OR, USA; reproduced with permission of Dr. Riebold. Figure 6.21 Examples of various guide wires and tube combinations used for guided endotracheal intubation. A wire or tube guide that is smaller and typically easier to place than the appropriately sized endotracheal tube is first placed in the trachea. The endotracheal tube is then fed over the guide, normally without further visualization. Once endotracheal intubation has been achieved, the guide is removed. Source: Dr. Craig Mosley, with permission. Laryngoscopy with a flexible or rigid endoscope can be useful for assisting intubation in patients with abnormal anatomy or disease processes involving the pharynx, head, or neck. It is also often used in patients that can be challenging to intubate using direct visualization (e.g., pygmy rabbits and other small mammals). Depending on the species and the specific conditions, the endoscope can be placed inside the endotracheal tube to guide intubation directly or passed orally beside the endotracheal tube to guide correct placement visually. Rabbits and other small mammals are frequently intubated using this technique [36]. When appropriate, endoscopes can also be used to facilitate nasotracheal intubation. This technique can be particularly advantageous in horses (and other large animals) with abnormal oropharyngeal, laryngeal, and/or nasal anatomy where direct visualization using a laryngoscope is virtually impossible without specialized custom equipment. Figure 6.22 Diagram illustrating the passage of an endotracheal tube into the trachea of a dog using a guide device (urinary catheter). Source: Hartsfield [37], with permission. Changing endotracheal tubes during a surgical or diagnostic procedure in an anesthetized animal is occasionally required due to a failing cuff or when an alternative tube size or length is required. Patients that are positioned and draped for surgery are generally not situated “normally” for intubation. Changing the tube using a wire‐ or tube‐guided technique may be the easiest and most efficient way to accomplish the procedure. However, in many instances, using good technique and a high‐quality laryngoscope will allow for intubation using direct visualization. Depending on the size of the patient, standard commercially available human tube exchangers can be used. Alternatively, any tubing or wire of sufficient diameter and length can be used; for example, stomach tubes and catheter wire guides. To change endotracheal tubes, the guide (tube or wire) is inserted through the original endotracheal tube to the area of the midcervical trachea. It should be noted that if the patient is being maintained under anesthesia using an inhalant anesthetic, it should be discontinued during tube exchange. Depending on the duration required to exchange the tube, the patient’s anesthetic depth may become very light, and additional intravenous anesthetic and a means to administer it should be readily available. Next, the endotracheal tube cuff is deflated, and the endotracheal tube is pulled over the guide without removing the guide from the trachea. Then, the new endotracheal tube is maneuvered through the larynx and into the trachea by using the guide to direct its passage. The cuff of the new tube is inflated to protect the airway, and the new tube is secured in the manner appropriate for the specific species. Figure 6.23 Illustration of intubation using a retrograde guide device in a dog. Catheter guide wires or other blunt‐ended wires are optimal for this procedure owing to their small diameter. This technique should be reserved for cases that cannot be intubated by other methods. Source: Hartsfield [37], with permission. If direct visualization of at least a portion of the glottis is impossible, retrograde intubation may be performed. Retrograde intubation has been evaluated as an alternative technique for endotracheal intubation in South America camelids and mice [38,39], and a cadaveric study has been performed in rabbits [40]. The technique essentially involves passing a needle through the skin of the ventral neck and into the trachea between upper tracheal rings. In human patients, the needle is passed through the cricothyroid membrane. A guidewire is then maneuvered through the needle rostrally into the larynx, pharynx, and oral cavity until it can be used as a guide for passage of an endotracheal tube (Fig. 6.23). The endotracheal tube is then fed over the guidewire and manipulated into the larynx. After the tip of the endotracheal tube is within the larynx, the needle and the guide tube are removed, and the endotracheal tube is manipulated into its final position with the cuffed end near the thoracic inlet. The cuff should be located caudal to the puncture site of the hypodermic needle to avoid forcing gases subcutaneously or into the mediastinum during positive‐pressure ventilation. Subcutaneous emphysema and pneumothorax are possible complications with this technique. A temporary tracheostomy can be performed for airway management during anesthesia. However, as it is invasive, it is typically reserved for those patients where oro‐ or nasotracheal intubation is not possible even with guided assistance, owing to anatomy, pathology, or surgical procedures. Occasionally a tracheostomy may be recommended to facilitate a surgical procedure involving the oropharynx although, if appropriate, a pharyngostomy tube may be a more desirable alternative in these cases. Tracheostomy tube placement may also be used when there is a reasonable expectation that the patient will require an ongoing tracheostomy following the anesthetic procedure (e.g., incomplete resection of laryngeal tumor, brachycephalic airway surgery) [41,42]. Occasionally patients may present to anesthesia with an emergency tracheostomy already present as the result of acute upper airway obstruction. Figure 6.24 An example of a cuffed tracheostomy tube with an inner stylet to ease placement. Some tubes are also designed with a removal inner tube to facilitate cleaning of the tube. Source: Dr. Craig Mosley, with permission. Intubation via a tracheostomy is relatively simple to perform but may be complicated in patients with very small‐diameter tracheas or those with very thickened and calcified tracheal rings (e.g., some brachycephalics). A standard endotracheal tube or tracheostomy tube can be used for intubation; however, care should be taken when using a standard‐length endotracheal tube as endobronchial intubation can easily result. Tracheostomy tubes are generally short with a pronounced curvature in the tube and an inner stylet to ease placement (Fig. 6.24). The curvature also allows the tube to be secured flatly to the patient’s neck and tubes are normally fitted with a standard 15 mm endotracheal tube adapter, inflatable cuffs, and pilot balloons, although many of the smaller‐sized tubes will be uncuffed. Some tracheostomy tubes also have an inner removable cannula to facilitate cleaning and longer‐term maintenance of tracheostomy tubes in patients. Care of the tube is very important. Neglected tubes that are not cleaned regularly can be obstructed by mucus that dries within the lumen of the tube. Tracheostomy has been associated with infection, granulomas, tracheal stricture, cartilage damage, hemorrhage, pneumothorax, subcutaneous emphysema, tracheocutaneous or tracheoesophageal fistula, aspiration, dysphagia, and tracheal malacia; hence tracheostomy should not be considered an innocuous procedure. The specific techniques, indications, and outcomes have been reviewed in cattle, horses, dogs, and cats [43–47]. A lateral pharyngotomy is an alternative to a tracheostomy and is suitable for facilitating surgical procedures of the mandible, maxilla, or oral cavity (Fig. 6.25). The use of a pharyngotomy provides improved visualization within the operative field during oropharyngeal surgery and normal dental occlusion to aid in the proper reduction of mandibular or maxillary fractures. It is also a potentially less invasive alternative to a tracheostomy when oral or nasal airway management is undesirable or unfeasible for facilitating a surgical procedure. This technique has been described in detail elsewhere [48]. The basics of tube placement involve passage of a correctly sized, cuffed endotracheal tube and making a routine skin incision caudal to the angle of the mandible. Then, hemostats are bluntly passed through the skin incision into the caudal part of the pharynx. After the endotracheal tube adapter has been removed, the adapter end of the tube is grasped and pulled from the pharynx, through the subcutaneous tissue, and through the skin incision. The endotracheal tube adapter is replaced, and the tube is reconnected to the breathing system for maintenance. A correctly placed tube should be secured to the skin with several sutures and/or tape. Supplemental oxygen is used in anesthetized and critically ill patients to increase the partial pressure of oxygen in arterial blood (PaO2) and to promote the delivery of oxygen to the tissues. When a patient is breathing room air, values for PaO2 that are less than 80 mmHg indicate the potential for hypoxemia. Any patient in respiratory distress or those likely to experience hypoxia in the perianesthetic period (e.g., brachycephalic breeds) should be provided supplemental oxygen. If the PaO2 decreases to less than 60 mmHg, the need for supplemental oxygen is indicated. It is also important to recall that both hemoglobin and tissue perfusion play fundamental roles in ensuring sufficient delivery of oxygen to tissues and when indicated red blood cell transfusions and/or cardiovascular support may be required in addition to providing supplemental oxygen to prevent cellular hypoxia. The fraction of oxygen in inspired gases (FIO2) plays a significant role in establishing the PaO2. As a rule, the PaO2 value should be approximately five times the FIO2 value if there are no major abnormalities in the matching of pulmonary ventilation and perfusion. Supplemental oxygen can be an effective way of correcting hypoxemia in animals with diffusion abnormalities, ventilation‐perfusion mismatching, and/or hypoventilation. In addition to oxygen supplementation, the specific underlying condition(s) contributing to hypoxemia should be addressed when possible. Supplemental oxygen may not significantly improve PaO2 in patients with substantial right to left vascular shunts (pulmonary or cardiac shunts). Several techniques can be used to administer oxygen to anesthetized and ill patients. The effectiveness of oxygen supplementation is assessed by evaluation of the patient’s clinical responses (e.g., improvement in mucous membrane color and character of ventilation), by measuring the FIO2, and by monitoring of PaO2, arterial oxygen saturation (SaO2), and saturation of peripheral oxygen (SpO2). Although PaO2 and SaO2 data are reliable, they require periodic arterial blood sampling and the use of a blood gas analyzer. The SpO2 can be conveniently measured by pulse oximetry. Pulse oximetry is a practical method for non‐invasive, moment‐to‐moment estimation of the saturation of hemoglobin with oxygen in anesthetized, recovering, and ill patients [49–56]. Various techniques for supplementing oxygen to patients are outlined below and Table 6.1 summarizes the approximate FIO2 obtained using each of the commonly available techniques in dogs and cats. Masks for delivery of oxygen to veterinary patients are useful for preoxygenation immediately before induction of anesthesia and for conscious patients experiencing respiratory distress. The use of masks for oxygenation requires constant attention, and some patients will not accept a mask unless they are sedated. Both factors limit the effectiveness of masks in conscious patients. Indeed, some patients object to a mask so vigorously that the increase in oxygen consumption associated with restraint may nullify the benefits of a greater FIO2. If the oxygen mask is attached to an anesthetic breathing circuit, the circuit should be flushed in order to reduce anesthetic odors and potentially improve compliance, but this is variably useful. Figure 6.25 An endotracheal tube placed by external pharyngostomy in a dog to facilitate oropharyngeal surgery. A. The machine end of the endotracheal tube is grasped via the pharyngostomy incision. B. The machine end of the endotracheal tube is exteriorized and sutured to the surrounding tissues. C. Completed tube placement distal to the left mandibular ramus demonstrating ability to assess proper dental occlusion in orofacial surgeries. Source: Dr. Craig Mosley, with permission. Table 6.1 Approximate FIO2 values obtained from various oxygen supplementation techniques for dogs and catsa. a These values are not applicable for all veterinary species during all circumstances and will be flow rate and respiratory character (rate and depth) dependent. The flow rates generally recommended for increasing FIO2 when using masks are variable among species. For example, flow rates of 10–15 L/min of supplemental oxygen have been recommended to increase the inspired oxygen concentration to approximately 35–60% in adult horses, although supporting studies are lacking, and nasal and tracheal insufflation represent better, more practical options in larger patients. Flow rates for smaller patients, including dogs and cats, usually range from 2 to 8 L/min to produce inspired oxygen concentrations of 30–60% [57], although specific supporting studies are lacking. There is also increasing interest in the use of high‐flow oxygen therapy in veterinary medicine [58]. With a tight‐fitting mask, higher flow rates of oxygen tend to produce greater FIO2 values and less rebreathing of expired carbon dioxide. Tight‐fitting masks should be used with a breathing system (anesthetic circuit or manual resuscitation bag) with a reservoir that can meet the patient’s tidal volume demands or with a vented or valved mask allowing entrainment of air from outside the mask. As an example, a dog with a tidal volume of 300 mL and an inspiratory time of 1 s has a peak inspiratory gas flow of approximately 18 L/min, which exceeds the practical flow rate for oxygen during masking. High inspiratory flow rates can be accommodated if the mask is attached to an anesthetic breathing system with a reservoir bag or a mask fitted to a manual resuscitation bag. In addition, an appropriate breathing system has an overflow that prevents the buildup of excessive pressure with a tight‐fitting mask. Alternatively, a loose‐fitting mask that allows room air to be entrained can be used, but entrained room air will reduce inspired FIO2 associated with supplemental oxygen delivery. Face masks for oxygen supplementation in veterinary patients are readily available (Fig. 6.26). Most consist of a clear plastic cone fitted with a black rubber diaphragm that can improve fit and sealing around a patient’s face. Unless they are sedated or otherwise minimally responsive, most conscious patients rarely accept snug‐fitting face masks. Although face masks designed specifically for very small patients are available (Fig. 6.27), it is not uncommon to use a plastic syringe case, latex glove, and endotracheal tube adapter to “custom” design a face mask (Fig. 6.28). Traffic cones have been adapted and fitted to pigs for delivery of inhalant anesthetics. Insufflation involves the delivery of oxygen into the patient’s airway at relatively high flow rates; the patient inspires oxygen and room air, the relative proportions of each being determined primarily by the oxygen flow rate and the rate of gas flow during inspiration. Insufflation can be accomplished by a variety of methods. For horses recovering from anesthesia, oxygen may be delivered from a flowmeter through a delivery tube and into an appropriately sized insufflation tube in the horse’s nasal cavity or trachea. Appropriately sized endotracheal tubes or large animal stomach tubes are often used as nasal tubes. Intact stomach tubes are sufficiently long that they may also be used for tracheal insufflation if advanced through the nasal cavity and into the trachea. For most conscious patients, oxygen is insufflated through a nasal catheter, the tip of which is positioned in the nasopharynx. The catheter is usually made of soft rubber, and the tube should have several fenestrations to minimize jetting lesions from developing in the nasopharyngeal mucosa. For awake patients, instilling 2% lidocaine (or lidocaine gel) or 0.5% proparacaine into the nasal passage with the patient’s head and neck extended and held upward may facilitate passage of the tube. Placement involves insertion of a suitable‐sized insufflation catheter into the nasal passage and the nasopharynx, the distance being approximately the same as from the tip of the nose to the medial canthus of the eye. Specifically designed nasal insufflation catheters (with multiple fenestrations) are available or they can be made from feeding tubes or other soft non‐reactive tubing and adapted to the oxygen delivery line. The external portion of the catheter is secured to the patient’s head with tissue adhesive, tape, and/or sutures or staples. A flexible length of tubing supplies oxygen from a flowmeter and allows the patient some freedom for movement in a cage or stall. Changing the catheter to the opposite nasal passage every 1–2 days has been recommended to prevent pressure necrosis, jet lesions, and accumulation of mucus [59]. Humidification of the oxygen is also advisable if the need for insufflation will be for more than a short period of time. Figure 6.26 Commercial face masks are available in many styles and sizes. A face mask should be chosen to minimize the potential for rebreathing of exhaled gases (i.e., snug fitting), especially when used with the rubber diaphragm in place. Source: Dr. Craig Mosley, with permission. Figure 6.27 Commercial face masks designed specifically for use with very small patients. A. The masks are available with two sizes of interchangeable diaphragms. B. Use of the face mask in a small avian patient. Source: Advanced Anesthesia Specialists, Phoenix, AZ, USA; with permission. Figure 6.28 Face masks can be fashioned from syringe cases and rubber gloves are often made for use in very small patients. Source: Dr. Craig Mosley, with permission. The flow‐rate requirements for oxygen during insufflation vary depending on a number of factors, with the patient’s ventilation character and the desired FIO2 being two of the most important. Following anesthesia, adult horses require a minimum of 15 L/min of oxygen flow to improve the PaO2 in arterial blood, and proportionally lower flows (e.g., 5 L/min) are suitable for smaller horses, foals, and calves [60–63]. In small animals, flow rates of 1–7 L/min are typically used for the administration of nasal oxygen. Approximate flow rates for dogs and cats to achieve rather specific ranges of FIO2 have been suggested but monitoring of SpO2 or PaO2 should ultimately guide flow rate adjustment [64]. In dogs, various flow rates of 100% oxygen administered intranasally were studied, and flow rates of 50, 100, 150, and 200 mL/kg/min produced inspired oxygen concentrations measured at the tracheal bifurcation of 28, 37, 40, and 47, respectively [59]. To prevent mucosal drying with prolonged insufflation, oxygen should be flowed through a bubble‐type humidifier. Frequently, bilateral nasal insufflation catheters are placed and can be expected to improve the maximum achievable FIO2, achieving up to 80% at 200 mL/kg/min [65]. Tracheal insufflation can be achieved via nasotracheal or transtracheal oxygen administration. These techniques are useful for patients suffering from conditions causing upper airway obstruction. Nasotracheal insufflation is achieved by passing a catheter through the nares into the trachea. In conscious animals, this process is normally performed after instilling a topical local anesthetic (lidocaine, proparacaine) into the nose and using a lubricant containing a local anesthetic (lidocaine gel). To facilitate tube placement into the trachea rather than the esophagus, the neck should be extended. Conscious patients will frequently cough as the catheter enters the larynx; coughing can be reduced by instilling a small amount of topical lidocaine through the nasotracheal tube on to the laryngeal area. Proper nasotracheal tube placement can be assessed by demonstrating a lack of negative pressure when air is evacuated from the tube/catheter. Air can be evacuated by attaching an appropriately sized syringe or compressed suction bulb to the catheter. If negative pressure is detected while evacuating air from the tube, this suggests that the tube is not in the trachea and may have been swallowed by the patient and is in the esophagus. Two‐view radiographs may also be used to help confirm proper tube placement. A transtracheal catheter can be placed percutaneously into the trachea through the cricothyroid membrane or between tracheal rings near the larynx and can be used to insufflate oxygen in a compromised patient. Intratracheal administration of 100% oxygen has been evaluated in dogs, and flow rates of 10, 25, 50, 100, 150, 200, and 250 mL/kg/min produced inspired oxygen concentrations at the tracheal bifurcation of 25, 32, 47, 67, 70, 78, and 86%, respectively [64]. The technique for tracheal insufflation has been described for small animals [64]. The catheter should be placed aseptically, be of the over‐the‐needle type, be relatively large bore, have several smooth fenestrations to prevent jet lesions, and ultimately be positioned with the tip near the carina. Oxygen should be humidified, and flow rates should approximate those used for nasal insufflation. Oxygen cages (Fig. 6.29) specifically designed for small animals are commercially available, but expensive. These cages regulate oxygen flow, control humidity and temperature, and eliminate carbon dioxide from exhaled gases. Most oxygen cages are capable of producing oxygen concentrations between 30 and 60% but the flow rates required can be as high as 15 L/min [66]. However, there is considerable variability in design and efficacy among many commercially available products. There is also considerable variation in the time required to obtain the desired oxygen concentration, usually 30–45 min [66]. Factors that will influence this lag time include internal volume of the cage, air tightness of the cage, and how often the cage is opened. For small animals, flow rates of oxygen, cage temperature, and cage humidity have been recommended to be less than 10 L/min (but this will depend greatly on overall efficiency of the cage), approximately 22 °C, and 40–50%, respectively [67]. Oxygen concentrations of 30–40% generally are adequate for patients with moderate pulmonary disease [68]. Oxygen cages are not practical for horses or larger animals and, even in smaller animals, the effectiveness of an oxygen cage diminishes as body size increases. Smaller patients can be managed easily in oxygen cages, but temperature and humidity are more difficult to control with larger dogs. A major disadvantage of an oxygen cage is that the animal must be removed from the cage (or the doors opened) for examination and treatment, requiring the patient to breathe room air or oxygen by mask during this period. Prior to purchasing an oxygen cage, it is important to understand fully its operation and oxygen requirements. Significant amounts of oxygen can be consumed by oxygen cages that are poorly sealed and during frequent openings of the cage for patient treatments and management. In these circumstances, the oxygen consumption required can quickly deplete a hospital’s oxygen reserves. Figure 6.29 Commercial oxygen cages are available that can precisely control the oxygen concentration within the cage and remove exhaled CO2. Many frequently also incorporate humidity and temperature controls (heating and cooling). Source: Dr. Craig Mosley, with permission. Comparatively, oxygen cages are a relatively inefficient method of oxygen supplementation but can be extremely useful for managing specific patients. Oxygen cages are best reserved for short‐term immediate supplementation of oxygen to smaller patients in respiratory distress. Clinically, some dogs and cats with serious ventilatory compromise respond very well to an oxygen‐enriched environment as initial therapy; the increase in FIO2 is associated with decreased ventilatory effort, and the patient stabilizes and becomes more manageable prior to further examination and treatment. Nasal insufflation is a far more effective and efficient method of oxygen supplementation in most circumstances, particularly those requiring longer‐term supplementation, even for smaller dogs and cats. Oxygen toxicity develops with prolonged exposure to high oxygen concentrations, leading to deterioration of pulmonary function. Initial effects are endothelial damage, destruction of alveolar cells, and increasing microvascular permeability, leading to edema, hemorrhage, and congestion [57]. Later stages of toxicity are associated with alveolar type II and fibroblast proliferation resulting in fibrosis [57]. The length of time that a patient’s PaO2 is elevated may be more predictive of oxygen toxicity than the duration of exposure to a high FIO2 [69]. There is significant species and individual variability in susceptibility to oxygen toxicity [69]. In veterinary patients, the following guidelines are recommended: (1) use a PaO2 of 70 mmHg as an endpoint of O2 therapy; (2) use the lowest FIO2 possible to achieve a PaO2 of 70 mmHg; and (3) do not use an FIO2 greater than 0.6 for longer than 24 h if possible [57]. Inhalant anesthesia forms the foundation of most modern anesthetic protocols in veterinary medicine. The administration of potent inhaled anesthetics requires specific delivery techniques. The anesthetic machine permits the delivery of a precise yet variable combination of inhalant anesthetic and oxygen. The basic components and functions of all anesthetic machines are similar but significant design differences exist among them. Machines can be very simple, for example, those used for mobile applications, to very complex anesthetic workstations with built‐in ventilators, monitors, and safety systems (Fig. 6.30). Regardless of the complexity of the design, all anesthetic machines share common components: (1) a source of oxygen, (2) a regulator for oxygen (this may be part of the gas supply system), (3) a flowmeter for oxygen, and (4) a vaporizer. If additional gases are used (e.g., nitrous oxide), there will also be a source, regulator, and flowmeter for each gas that generally parallels the path of oxygen with some exceptions (e.g., oxygen flush valve). The basic anesthetic machine is then used in conjunction with a breathing circuit and anesthetic waste gas scavenge system for delivery of anesthetic to and from the patient. Anesthetic machines ideally have two gas supplies, one from small, high‐pressure tanks attached directly to the machine and a second source often originating from a hospital’s central pipeline supply. The small tanks mounted directly to the anesthesia machine are normally intended to be used as back‐up or reserve gas sources should the pipeline malfunction or for working in an area without access to the pipeline. Oxygen is by far the most commonly used medical gas during veterinary anesthesia, with nitrous oxide being used in conjunction with oxygen as an adjunct carrier gas for inhalants much less frequently. Most medical gases are normally stored under high pressure in gas cylinders of various sizes or in low‐pressure insulated cryogenic liquid bulk tanks. The characteristics (e.g., working pressure) and capacity of the gas cylinders vary with the type of gas they contain (see Table 6.2). Alternatively, oxygen concentrators can be used to supply a hospital with its oxygen requirements in circumstances where obtaining and storing tanks is inconvenient, impossible, or prohibitively expensive (e.g., remote communities). Most oxygen concentrators use a system of absorbing nitrogen from air to produce gas with an oxygen concentration between 90 and 96%. Small, integrated, single‐machine oxygen concentrating units have been made available in the veterinary market (Pureline™, Supera Anesthesia Innovations, Clackamas, OR, USA) (Fig. 6.31). Figure 6.30 Anesthetic machines for veterinary use can vary considerably in their complexity and sophistication. A. Complete veterinary anesthetic workstation for large animal use. B. Portable anesthetic system for field use. Both systems provide all the components necessary for the controlled delivery of inhalant anesthetics. Source: part A., Hallowell EMC, Pittsfield, MA, USA; reproduced with permission. Source: Part B., Dr. Craig Mosley, with permission. Table 6.2 Characteristics of medical gas cylinders. Source: Adapted from Dorsch and Dorsch [2]. ISO, International Organization for Standardization; psi, pounds per square inch. Most modern veterinary facilities will have some form of central gas supply and pipeline distribution system delivering medical gases to various work sites. The complexity of these systems can vary significantly, from a small bank of large (G or H) cylinders and a regulator to more complex systems consisting of multiple large liquid oxygen tanks, automatic manifolds, regulators, alarms, and banks of large high‐pressure cylinders for back up (Fig. 6.32). The size and complexity of the gas distribution system will depend upon the gas needs, the area of required gas distribution, and the number of work sites required. Proper installation of large gas distribution systems is essential for safety and efficacy. All gas installations should be installed and properly evaluated by those with expertise in this area prior to using them to deliver gas to patients. There are several international (ASTM), national, and local documents related to the safe use, transport, and storage of pressurized gases. There are also standards surrounding the installation of medical gas piping systems and some of these provisions have been incorporated into hospital accreditation requirements in veterinary medicine. However, the specific guidelines can vary significantly among jurisdictions and regions. There have been several well‐documented medical accidents related to the inappropriate use of medical gases in humans, but the incidence of such accidents seems to be decreasing [70,71]. The reduction of such accidents is likely due in large part to better monitoring and maintenance of gas delivery systems. Consequently, there are several safety systems that have been developed to help reduce and eliminate these problems. For example, all anesthetic equipment has a gas‐specific non‐interchangeable connector that is part of the base unit (anesthetic machine, ventilator). These connectors, diameter index safety system (DISS), pin index safety system (PISS), and quick connector are described below. Figure 6.31 Small, quiet, portable oxygen concentrators are becoming increasingly available as an alternative to using refillable compressed gas tanks. This figure depicts an anesthesia machine with a built‐in oxygen concentrator. The oxygen concentrator is located in the case on the bottom of the machine. Source: Supera Anesthesia Innovations, Clackamas, OR, USA; reproduced with permission. Gas cylinders and gas lines are commonly color‐coded to avoid improper use, but color‐coding systems can vary among countries. For example, oxygen is colored white in Canada and green in the United States. In addition to color coding, all tanks have a labeling scheme consisting of various shaped labels, key words, and colors that are all used to identify hazards associated with the gas they contain. Most tanks originating from gas supply facilities normally have perforated tags (full, in use, empty) to track the cylinder’s use status. Figure 6.32 Bulk oxygen requirements for larger hospitals are frequently met using either A. large liquid oxygen tanks or B. banks of compressed gas tanks. Both of these systems are normally designed with a backup supply should the main supply fail or become depleted. Source: Dr. Craig Mosley, with permission. Figure 6.33 The diameter index safety system (DISS) uses a gas‐specific non‐interchangeable thread pattern to avoid incorrect gas delivery. Gases are also color‐coded as apparent in the figure. Source: Dr. Thomas Riebold, College of Veterinary Medicine, Oregon State University, Corvallis, OR, USA; reproduced with permission of Dr. Riebold. The diameter index safety system (DISS) is a non‐interchangeable gas‐specific threaded connection system (Fig. 6.33). DISS is the gas connection used almost universally by all equipment and cylinder manufactures for the connection of medical gases. There are many proprietary (manufacturer‐specific) quick‐connect systems that have been developed. These are standardized within a manufacturer but are not generally compatible with the quick‐connect systems of another manufacturer (Fig. 6.34). These systems facilitate rapid connecting and disconnecting of gas hoses and may be useful in situations where frequent connects and disconnects are required (e.g., multipurpose work areas). Figure 6.34 Proprietary quick‐connect gas couplings are available to ease frequent connecting and disconnecting of gas lines. These systems, such as the Ohmeda system pictured here, are manufacturer‐specific and are incompatible among various manufacturers. Source: Dr. Thomas Riebold, College of Veterinary Medicine, Oregon State University, Corvallis, OR, USA; reproduced with permission of Dr. Riebold. The pin index safety system (PISS) uses gas‐specific pin patterns that only allow connections between the appropriate cylinder yokes and small gas cylinders (E size). The PISS is commonly found on the yokes mounted on anesthesia machines and some cylinder‐specific regulators/flowmeters (Fig. 6.35). Figure 6.35 The pin index safety system (PISS) uses a series of gas‐specific pin positions on the yolk that correspond to similarly positioned pin receiver ports on the tank. A. The PISS typical of the cylinder yolk of an anesthesia machine. B. The PISS being used on a combination regulator/flowmeter for the delivery of oxygen. Source: Dr. Craig Mosley, with permission. Figure 6.36 Pressure‐reducing valves/regulators are used to decrease the pressure of the gas in a compressed gas cylinder down to a lower pressure, sometimes referred to as the “working pressure,” normally 40–55psi. These valves maintain a constant pressure delivery from the gas supply and help prevent fluctuations associated with gas depletion and use. Source: Dr. Craig Mosley, with permission. The pressure‐reducing valve (regulator) is a key component required to bring the high pressures of gas cylinders down to a more reasonable and safe working pressure (i.e., 40–55 psi). Regulators also reduce or prevent fluctuations in pressure as the tank empties. Regulators are normally found wherever a high‐pressure gas cylinder is in use (e.g., gas pipelines, cylinder connected directly to machine) (see Fig. 6.36). The regulators used for pipelines are normally adjustable whereas those on most anesthesia machines are set by the manufacturer. The ASTM standard requires that regulators on anesthesia machines be set to use pipeline gases preferentially before using gas from the backup cylinder on the anesthesia machine. However, since neither pipeline systems nor veterinary anesthesia machines are required to meet ASTM standards, it is not uncommon for machines to draw from the reserve or backup tank preferentially rather than the pipeline. This problem can be avoided by ensuring that the pipeline pressure is set approximately 5 psi higher than the anesthesia machine’s regulator for the reserve oxygen cylinder. Pressure gauges are commonly used to measure cylinder pressures, pipeline pressures, anesthetic machine working pressures, and pressures within the breathing system. Cylinder, pipeline, and anesthetic machine working pressures are normally expressed in pounds per square inch (psi) or kilopascals (kPa), whereas the pressures within the breathing system of the anesthetic machine are normally expressed in centimeters of water (cmH2O) (Fig. 6.37). The gauge measuring the pressure of the breathing system is often also referred to as a “pressure manometer.” The information provided by these gauges is vital for safe operation of anesthesia equipment. The basic anesthesia delivery apparatus is made up from a series of parts that work collectively to deliver inhalant anesthetics safely and support breathing. These components include: the carrier gas delivery system (normally oxygen), the vaporizer, the breathing circuit, and the waste gas scavenge system. Perhaps the simplest way to describe an anesthetic machine is to describe the components in order of the flow of gas through the machine, from source to patient. However, prior to describing these components, it is important to recognize that the pressures of gas vary at different locations in an anesthesia machine and knowledge of these pressures facilitates the evaluation and safe operation of these machines. There are high‐, intermediate‐, and low‐pressure areas. The high‐pressure area accepts gases at cylinder pressure and reduces and regulates the pressure; this area includes gas cylinders, hanger yokes, yoke blocks, high‐pressure hoses, pressure gauges, and regulators, with the pressure being as high as 2200 psi. The intermediate‐pressure area accepts gases from the central pipeline or from the regulators on the anesthesia machine and conducts them to the flush valve and flowmeters; this area includes pipeline inlets, power outlets for ventilators, conduits from pipeline inlets to flowmeters, and conduits from regulators to flowmeters, the flowmeter assembly, and the oxygen‐flush apparatus. The pressure usually ranges from 40 to 55 psi. The low‐pressure area consists of the conduits and components between the flowmeter and the common gas outlet; this area includes vaporizers, piping from the flowmeters to the vaporizer, conduit from the vaporizer to the common gas outlet, and the breathing system. The pressure in the low‐pressure area is close to ambient pressure. Pressures within the low‐pressure area can vary depending upon how the system is being used (e.g., positive‐pressure ventilation) but should generally never exceed 30 cmH2O as these pressures are transmitted directly to the patient’s lungs (see Fig. 6.38). Figure 6.37 Pressure gauges are used to measure pressure in gas cylinders, anesthetic machines, and breathing systems. A. Pressure gauge associated with a compressed oxygen tank, measured in pounds per square inch (psi). B. Pressure gauge found commonly on most anesthetic breathing circuits. Note that the pressure in the breathing circuit is measured in cmH2O. Source: Dr. Craig Mosley, with permission. Figure 6.38 Diagram of the basic anesthetic machine and circle breathing system. The exact positions of the various components and specific features can vary markedly among manufacturers. Source: Dr. Kath Klassen, Douglas College, New Westminster, BC, Canada; reproduced with permission of Dr. Klassen. Occasionally in veterinary medicine, multiple medical gases (e.g., oxygen, air, and nitrous oxide) are used with the anesthetic machine. However, 100% oxygen is normally the only gas used to deliver anesthesia and power anesthetic equipment (e.g., ventilators) in veterinary medicine. If the reader plans to use multiple gases for delivering anesthesia, it is their responsibility to understand fully the implications of the usage (indications and contraindications) and to ensure that the anesthetic equipment is properly designed and monitored to prevent the possibility of delivering a hypoxic gas mixture to the patient. All human machines must have a proportioning system associated with the oxygen flow and oxygen concentration monitors to ensure that a hypoxic gas mixture cannot be delivered; this is not generally the case with veterinary anesthesia machines. The flow of gas within an anesthetic machine may take multiple routes once it enters the intermediate pressure areas of the machine. Minimally, gas must be delivered to the flowmeter, where it is then directed to the vaporizer and subsequently to the patient. However, in addition to this route of movement, there may be several more routes available for gas distribution in the anesthesia machine. Normally, on most anesthesia machines intermediate pressure gas is also diverted to a fresh gas flush valve that bypasses the flowmeter and vaporizer and delivers fresh gas directly to the breathing circuit. There are circumstances where flush valves may not be present or are unavailable on veterinary anesthesia machines. Additionally, gas from the intermediate pressure area may be diverted to one or more auxiliary oxygen outlets that may be used as the driving gas for a built‐in or external ventilator or an external oxygen flowmeter. Flowmeters control the rate of gas delivery to the low‐pressure area of the anesthetic machine and determine the fresh gas flow (FGF) to the anesthetic circuit. There must be a separate flowmeter for each gas type used with the anesthetic machine (Fig. 6.39). The type of breathing system used, the volume of the breathing circuit, and the size of the patient are all factors that influence the FGF rate. Figure 6.39 Two flowmeters arranged in parallel on an anesthetic machine with nitrous oxide (blue) on the left and oxygen (green) on the right. Flowmeters on human anesthesia machines must conform to specific positioning (i.e., oxygen right‐most flowmeter) and control‐knob dimension (i.e., oxygen is furled and protrudes out furthest); this is not the case for flowmeters on anesthetic machines designed solely for veterinary use. Source: Dr. Craig Mosley, with permission. There are several flowmeter designs available, but most are based on a tapered gas tube with a movable float. The gas normally flows into the bottom of the tube and out at the top. The tube is narrower at the bottom and wider at the top, so as the float moves up the tube more gas can flow around the float, producing higher flow rates (Fig. 6.40) The amount of gas entering the tube is controlled by a flow‐control knob that adjusts a needle valve. A float indicates the gas flow on a calibrated scale. The gas flow rates are normally expressed in mL/min or L/min. The spatial distance between vertical markings on the flowmeter does not necessarily correspond to equal changes in flow rate. In other words, the distance between 0 and 1000 mL as measured vertically on the flowmeter may not be the same as the vertical distance between 1000 and 2000 mL. This is similar to the spatial separation of the percentages found on many vaporizers where there is a greater spatial allocation on the dial for normal working percentages than for those rarely used. Some anesthetic machines may also have two flowmeters for the same gas placed in series for allowing even greater precision at lower gas flow rates (Fig. 6.41). Flowmeters are gas‐specific and calibrated at 760 mmHg and 20 °C; accuracy may be affected if they are used under conditions significantly different from calibration, although this is rarely significant for routine clinical use. Flowmeters are also calibrated as a unit (flow tube, scale, and float) and therefore if any one of these fail it is best to replace the whole unit. Gasket, flow‐control dial/knob, and/or washer replacement or repair are unlikely to affect accuracy but should only be performed by individuals familiar with flowmeter design and operation. The flow‐control knob on contemporary human anesthesia machines must conform to ASTM standards. For example, the oxygen flow‐control knob must be uniquely shaped, and it must be on the right‐most side of the flowmeter bank downstream of all other gases. These design considerations are important for minimizing the accidental delivery of hypoxic gas mixtures. Figure 6.40 Diagram of a flowmeter illustrating gas flow from bottom to top with single and double tapers. As the flow indicator (bobbin, ball, or spindle) rises, flow increases because the orifice size increases. The double taper allows increased accuracy at the lower end of the tube while accurately metering higher flows from the top. Source: Hartsfield [72]; reproduced with permission. Figure 6.41 An example of multiple flowmeters being used on a single anesthetic machine designed for human use. Note the positioning of the flowmeters and shape of the control knobs. Also note that the nitrous oxide and oxygen flowmeters are dual‐stage flowmeters in series allowing for increased precision of gas delivery. Source: Dr. Craig Mosley, with permission. Figure 6.42 Schematic of a generic variable‐bypass vaporizer demonstrating the splitting of the fresh gas flow to the vaporizing chamber and bypass channel. The splitting ratio and vapor pressure of the volatile anesthetic will determine the final percent output from the vaporizer. Source: Dr. Craig Mosley, with permission. Vaporizers change liquid anesthetic into vapor and meter the amount of vapor leaving the vaporizer. Most modern vaporizers are agent‐specific, concentration‐calibrated, variable‐bypass, flow‐over with wick, out‐of‐circuit, and high‐resistance (or plenum) vaporizers that are compensated for temperature, flow, and back‐pressure. Non‐precision, low‐resistance, in‐circuit vaporizers are rarely found in veterinary medicine and, without accurate inspired inhalant concentration monitoring, these vaporizers would seem to pose unnecessary risks during anesthesia. Consequently, their use is discouraged unless used in conjunction with inhalant anesthetic agent monitoring. Most modern vaporizers work by splitting the carrier gas to flow into the vaporizing chamber (where it picks up anesthetic vapor) or to the bypass channel where it does not (Fig. 6.42). The ratio of the amount of gas that picks up inhalant to the gas that bypasses the inhalant, along with the vapor pressure of the volatile anesthetic, will determine the final concentration of the gas leaving the vaporizer. The output from the vaporizer is expressed as a concentration (e.g., volume percent) of vapor in the gas leaving the vaporizer. Temperature, flow, and pressure are all factors that can potentially alter vaporizer output. The mechanisms for temperature, flow, and pressure compensation vary among vaporizer manufacturers and models. In general, most modern precision compensated vaporizers will maintain consistent output at flows between 0.5 and 10 L/min, temperatures between 15 and 35 °C, and pressure changes associated with positive‐pressure ventilation and the use of the flush valve. Temperature compensation is achieved by using materials for vaporizer construction that supply and conduct heat efficiently, promoting greater thermostability. In addition, most vaporizers have mechanical, and a few have electrical circuit‐driven, thermocompensation systems. These vaporizers compensate for temperature changes by altering the splitting ratio so that a greater or lesser amount of gas is conducted through the vaporizing chamber as the temperature changes during use. In mechanical systems, as the vaporizer cools, the thermal element restricts gas flow to the bypass chamber, causing more carrier gas to enter the vaporizing chamber. The opposite will occur if the vaporizer becomes too warm. The exact thermocompensation systems vary among manufacturers but the thermal element is normally a heat‐sensitive metal(s) that will reliably expand and contract when subjected to temperature changes. Changes in gas flow rate through the vaporizer can potentially lead to changes in the output. For example, if the flow is excessively high, complete saturation of the gas moving through the vaporizing chamber may not occur, leading to a reduction in output. Flow rate compensation is achieved by ensuring reliable and consistent saturation of all gas flowing through the vaporization chamber by using a series of wicks, baffles, and spiral tracks to facilitate liquid gas vaporization. These techniques are used to increase the surface area of the carrier gas–liquid interface, ensuring that all the gas exiting the vaporization chamber is fully saturated. All properly functioning modern vaporizers have very predictable outputs within a clinically useful range of gas flow rates (0.5–10 L/min). Back‐pressure on the vaporizer can occur during intermittent positive‐pressure ventilation (IPPV) or with the use of the flush valve, and this effect can increase vaporizer output if compensation mechanisms are not in place. There are a number of ways in which the effects of back‐pressure are minimized in modern vaporizers. One is to reduce the size of the vaporizing chamber relative to the bypass chamber. Another option is to use a long, spiral, or small‐diameter tube connecting the vaporizer chamber to the bypass channel. Both will reduce the amount of pressurized gas reaching the vaporizing chamber. The use of one‐way check valves immediately upstream from the vaporizer can also prevent the effects of back‐pressure and they are incorporated into some machines. One‐way check valves are also sometimes used downstream of the flush valve (upstream of the vaporizer) to prevent back‐pressure associated with use of the flush valve. There are many vaporizers available for use in veterinary anesthesia, but most are based on three main styles of vaporizers: the Ohmeda Tec, the Dräger Vapor, and the Penlon Sigma series. The primary differences among vaporizer series include capacity of the vaporization chamber (larger chambers allow longer times between fillings, particularly useful for large animal applications), susceptibility to alterations in output due to tipping (rarely a consideration under normal circumstances), and mounting options. All are very accurate and well designed; the use of one over another is often more about personal preference and availability than performance differences. Figure 6.43 Many vaporizers are filled using agent‐specific filler keys represented by the four fillers located on the left in A. The keys are indexed to both the bottle and vaporizer (B.) and prevent inadvertent filling of a vaporizer with the wrong anesthetic agent. There are also many vaporizers that are not equipped with a keyed filler port and instead use a standard screw‐topped filler port. Filling of these vaporizers is best performed using an inhalant‐specific filler spout (second right‐most in A.). These are indexed to a specific agent bottle but not the vaporizer. Source: part A., Dr. Thomas Riebold, College of Veterinary Medicine, Oregon State University, Corvallis, OR, USA; reproduced with permission of Dr. Riebold. Source: part B., Dr. Craig Mosley, with permission. Most vaporizers are mechanical devices requiring no external power to function normally. However, due to the unique vapor properties of desflurane, this agent requires a specially designed electronic heated vaporizer to ensure consistent output. Despite the need for external power, most desflurane vaporizers function much like standard variable‐bypass vaporizers, although technically they are classified as measured‐flow vaporizers (see the description of the Tec 6 vaporizer below). There are also electronic vaporizers available for use in both humans (Aladin™ and Aladin2™ cassette vaporizer, GE Healthcare) and veterinary anesthesia (Vetland EX3000 Electronic Vaporization System). These vaporizers function as variable‐bypass vaporizers but the splitting ratio of the carrier gas is determined electronically rather than mechanically. Since the system is electronic, various manufacturers and user alarm settings can be incorporated. For example, the vaporizer may alert the user if unusually high concentrations of anesthetic are being delivered from the vaporizer or if the vaporizer setting has not been altered within a specified time period, potentially avoiding inadvertent anesthetic overdose (e.g., the vaporizer output is momentarily increased and the user forgets to reduce the setting). Although electronic systems arguably provide additional information that may be valuable to the anesthetist, they may also be more prone to problems and damage related to the fact they rely on properly operating electronics to function. More recently, target‐controlled inhalation anesthesia, also called “end‐tidal‐driven anesthetic delivery,” has become available in some countries on newer anesthetic machines (e.g., Aisys CS2, Anesthesia Delivery System with Et Control, GE Healthcare). These systems allow the anesthetist to select a target end‐tidal inhalant concentration and use the sampled end‐tidal readings and computer processing to effectively optimize vaporizer output and FGF to achieve efficient inhalant delivery with minimal waste anesthetic gas (i.e., closed FGF rates) and oxygen use. These systems help the user safely and easily utilize low FGF rates; thereby, reducing costs, waste, and environmental contamination [73,74]. Vaporizers can be filled using a standard screw‐capped filler port or an agent‐specific keyed filler port. Keyed filler ports are intended to prevent inadvertent filling of a vaporizer with the wrong anesthetic agent (Fig. 6.43). Most modern vaporizers are extremely dependable and durable, requiring very little routine maintenance and care. However, maintenance and care should be performed according to the manufacturer’s recommendations and only by a qualified technician. Vaporizers are generally mounted on what is referred to as the “back bar,” which simply refers to the rail or mounting system used to hold vaporizers on the machine. Most veterinary vaporizers use cagemount systems for securing the vaporizer to the back bar; this system uses 23 mm taper push fittings (inlet and outlet, female and male) to attach the vaporizer to the gas delivery system, and the vaporizer is bolted directly to the back bar (Fig. 6.44). The tapered fittings are often referred to as “vaporizer caps,” “adapters,” or “elbows.” There are also several proprietary mounting bracket systems that allow vaporizers to be easily removed; for example, the GE Healthcare Ohmeda Selectatec and the Dräger Interlock, with the former being significantly more popular. The Selectatec mount consists of two vertically situated male valve ports, with an accessory pin and locking recess located between the inlet and outlet ports (Fig. 6.45). Selectatec‐compatible vaporizers have two female ports with a recessed assembly to receive the accessory pin. The vaporizer is lowered on to the male valve ports and then locked into place using the locking knob located on the top of the vaporizer. The O‐rings on the male valve ports ensure a gas‐tight seal between the vaporizer and the Selectatec mount. Loss or deformation of these rings can lead to leaks between the vaporizer and the mount. The vaporizer cannot be turned on unless it is locked in place on the mount. When the vaporizer is turned on (Tec 4, 5, 6, 7), a retractable spindle depresses the ball valve in the male valve ports, allowing gas to flow from the vaporizer to the anesthetic machine. Tec 4 and above vaporizer models also incorporate a safety interlock system (vaporizer exclusion/isolation) that uses a horizontal push rod system that ensures that only one vaporizer can be used at a time when multiple vaporizers are mounted in series on a Selectatec mount. The Dräger Interlock mount system is very similar to the Selectatec mount and also includes a safety interlink system; however, the dimensions are unique, meaning that each system can only be used with a vaporizer compatible with the individual mounting system. Most vaporizers are available in models compatible with the Selectatec mounting system. Figure 6.44 An example of a typical cagemount vaporizer with tapered push fittings for delivery of gas to and from the vaporizer. Source: Dr. Craig Mosley, with permission. The vaporizers described here are those most commonly used in veterinary anesthesia. Earlier vaporizers no longer being manufactured may still be in use and some have been described in earlier editions of this book and elsewhere [75,76]. Figure 6.45 A Selectatec mounting bar for Tec‐style vaporizers. This mounting system is convenient for quick placement and removal of vaporizers. The vertical male ports deliver gas to and from the vaporizer; the pin between the two ports is the locking system used to mount the vaporizer The vaporizer must be locked in place before the control dial can be turned on. Source: Dr. Craig Mosley, with permission. Figure 6.46 A machine mounted with several of the Ohmeda/Datex‐Ohmeda Tec series of vaporizers. From the left: the Tec 4, Tec 5, and Tec 6 vaporizers. Source: Dr. Craig Mosley, with permission. Tec vaporizers specifically designed for halothane or isoflurane vaporization, particularly the Fluotec Mark 3 and Isotec 3, are commonly used in veterinary anesthesia and are described in more detail below. They are considered reliable and are temperature, flow, and back‐pressure compensated under normal operating conditions. The Fluotec Mark 3 predecessor, the Fluotec Mark 2, is no longer being manufactured but may still be available as used equipment and may still be found in some veterinary practices [75]. It was associated with relatively poor performance characteristics and replacement with more accurate and reliable units should be considered. Tec 4, 5, 6, and 7 vaporizers have superseded the Tec 3 vaporizers for use with contemporary human anesthesia machines [2]. The Tec 4 was only available for the delivery of isoflurane and the Tec 6 is a desflurane‐specific model (Fig. 6.46). The Tec 5 series is available for delivery of enflurane, halothane, isoflurane, and sevoflurane and has been replaced by the more recent Tec 7 series of vaporizers (Fig. 6.47). These vaporizers are becoming increasingly common for use in veterinary anesthesia and various publications and specific operating manuals offer information about their use and performance [1]. Figure 6.47 A Datex‐Ohmeda/GE Healthcare Tec 7 isoflurane‐specific vaporizer. The Tec 7 series is the latest variable‐bypass plenum vaporizer series offered by Datex‐Ohmeda/GE Healthcare. Source: Dr. Thomas Riebold, College of Veterinary Medicine, Oregon State University, Corvallis, OR, USA; reproduced with permission of Dr. Riebold. These vaporizers are classified as variable‐bypass, flow‐over with wick, automatic thermocompensation, agent‐specific, high‐resistance, and back‐pressure compensated [68]. The Tec 3 model includes the Fluotec Mark 3, the Pentec Mark 2, and the Isotec 3 vaporizers (Fig. 6.48). As the Fluotec and Pentec were specific for halothane and methoxyflurane, respectively, and these inhalants are no longer marketed, it is unlikely that these vaporizers have clinical applicability in veterinary practice today. The Tec 3 (Isotec 3) vaporizer is temperature compensated with a bimetallic, temperature‐sensitive element associated with the vaporization chamber. Output from the Tec 3 vaporizer is nearly linear over the range of concentrations and flow rates that would typically be selected for veterinary patients (250 mL/min–6 L/min). Back‐pressure compensation is accomplished in the internal design of the vaporizer with a long tube leading to the vaporization chamber, an expansion area in the tube, and exclusion of wicks from the area of the vaporization chamber near the inlet [76]. These vaporizers are classified as variable‐bypass, flow‐over with wick, automatic thermocompensation, agent‐specific, high‐resistance, and back‐pressure compensated [76]. The vaporizer’s main design features over earlier models are associated with its obligatory use with a Selectatec mounting system. The Tec 4 was the earliest version of these vaporizers and was only available for isoflurane delivery (Fig. 6.49). The Tec 5 is available for the delivery of enflurane, halothane, isoflurane, and sevoflurane and can be found in either a key‐ or funnel‐filled configuration (Fig. 6.50). When the dial is turned to “0” and the flowmeter is activated, all of the gas bypasses the vaporizer through the Selectatec mount rather than passing through the vaporizer’s bypass channel. Only when the vaporizer is turned from “0” will the gas enter the vaporizer. Temperature compensation is accomplished through a bimetallic strip located in the bypass channel; as the temperature decreases, less gas is allowed through the bypass channel so that relatively more gas passes through the vaporizing chamber. Tec 5 vaporizers also feature a safety interlock system (vaporizer isolation/exclusion system), activated when multiple vaporizers are mounted in series. Figure 6.48 An example of a Tec 3 style isoflurane‐specific vaporizer. This style of vaporizer is very common in many veterinary practices. Source: Dr. Craig Mosley, with permission. Figure 6.49 The Ohmeda Tec 4 (IsoTec) was the first vaporizer designed for use with the Selectatec mounting system and was only available for the delivery of isoflurane. Source: Dr. Craig Mosley, with permission. Figure 6.50 The Tec 5 series of vaporizers included enflurane‐, halothane‐, isoflurane‐, and sevoflurane‐specific models. The Tec 5 model also incorporated an interlock safety system that prevents multiple vaporizers from being turned on when mounted in series. Source: Dr. Thomas Riebold, College of Veterinary Medicine, Oregon State University, Corvallis, OR, USA; reproduced with permission of Dr. Riebold. The Tec 6 vaporizer is designed for use only with desflurane (Fig. 6.51). Owing to its relatively low potency and low boiling point (near room temperature), desflurane liquid requires heating to ensure complete and stable vaporization of the liquid and accurate vaporizer output. The vaporizer is classified as a measured‐flow vaporizer, automatic thermocompensation, agent‐specific, high‐resistance (see below), and back‐pressure compensated. The vaporizer uses electronics to indicate its operational status, the level of agent, to control the pressure balance (between the diluent/bypass gas and the vaporized inhalant), to heat the liquid desflurane, and to charge the backup battery. The vaporizer can only be turned on once the electronics determine it to be operable. The heaters in the agent sump heat the desflurane to 39 °C, producing saturated desflurane at a vapor pressure of approximately 1500 mmHg in the reservoir. Figure 6.51 The Datex‐Ohmeda Tec 6 vaporizer is a desflurane‐specific model incorporating a heated vaporizing chamber. The Tec 6 Plus from Datex‐Ohmeda/GE Healthcare is the latest available in this vaporizer series. Source: Dr. Craig Mosley, with permission. When the vaporizer is turned on, the valve is opened and the pressurized vapor moves to a pressure regulator that reduces the pressure to that normally found in a high‐resistance (plenum) vaporizer (10–20 cmH2O). The desflurane vapor then moves to a variable restrictor controlled by the concentration selection dial, where it is added to the carrier gas leaving the vaporizer. The fresh gas (carrier gas) flow into the vaporizer passes through a fixed restrictor that increases its pressure to that normally found in a high‐resistance (plenum) vaporizer. Two independent pressure sensors in the pathway detect pressure changes and instruct the pressure regulator to change the desflurane pressure (and flow) proportionately so as to maintain the set vapor concentration. For example, if the FGF rate is increased, the pressure created by the carrier gas moving through the fixed restrictor will increase. This will then instruct the desflurane pressure (and flow) to increase proportionately, maintaining the vaporizer output despite changes in carrier gas flow. If the readings from the two sensors are not similar, the vaporizer will shut off the vaporizing chamber, initiating an alarm. The filler port accepts only the specific filler nozzle associated with the desflurane bottle. Dräger Vapor 19.1 series vaporizers are commonly found in veterinary medicine. An interlock‐compatible model is also available, the Vapor 19.3 series. The Vapor 19.1 probably first became popular in veterinary anesthesia for large animal applications (Fig. 6.52). The Vapor 19.1 had a significantly larger inhalant reservoir capacity relative to the standard Tec 3 and 4 vaporizers commonly used at the time (although extended capacity Tec 3 models were also produced). The larger inhalant reservoir is well suited to the increased inhalant demands associated with large animal inhalant anesthesia and reduced the likelihood of needing to refill the vaporizer in the middle of a case. The Vapor 19.1 series vaporizers are classified as variable‐bypass, flow‐over with wick, automatic thermocompensation, high‐resistance, agent‐specific, and pressure compensated [76]. Specific vaporizers are available for sevoflurane, isoflurane, enflurane, and halothane administration. Temperature compensation is automatic with a temperature compensator. The temperature compensator makes use of the thermal characteristics of two different materials to expand or contract the bypass channel, decreasing or increasing the amount of gas passing through the vaporization chamber. Pressure compensation is accomplished by the presence of a long spiral inlet tube to the vaporization chamber [2]. This vaporizer is accurate from 0.3 to 15 L/min of FGF at the lower settings on the control dial, but complete saturation may not occur at higher settings with higher flows. The vaporizer is designed for operation (temperature compensation) in the range 10–40 °C [2]. Dräger also produces desflurane‐specific vaporizers, the D Vapor and the DIVA, which are additional examples of measure flow vaporizers, similar to the Tec 6. Figure 6.52 Isoflurane and sevoflurane Dräger Vapor 19.1 vaporizers mounted on a large animal anesthesia machine. These vaporizers probably first became popular for use in veterinary medicine for providing large animal anesthesia owing to their relatively large inhalant reservoir, requiring less filling during a case. Source: Dr. Thomas Riebold, College of Veterinary Medicine, Oregon State University, Corvallis, OR, USA; reproduced with permission of Dr. Riebold. Penlon Sigma Delta vaporizers are another type of vaporizer occasionally used in veterinary medicine. The Sigma Delta vaporizer (Fig. 6.53) is classified as variable‐bypass, flow‐over with wick, temperature compensated, high‐resistance, agent‐specific, and vaporizer‐out‐of‐circuit (VOC) [76]. Specific vaporizers are available for sevoflurane, isoflurane, enflurane, and halothane administration. Penlon also produces a desflurane vaporizer, the Sigma Alpha. Temperature compensation is through a thermostat in the bypass channel. The operating temperature is between 15 and 35 °C and the temperature‐compensating mechanism reacts slowly, requiring 1–2 h to compensate for changes in room temperature. This vaporizer is accurate from 0.2 to 15 L/min of FGF at all dial settings, with only slightly decreased output at lower settings and slightly increased output at higher settings when using very low flow rates. Figure 6.53 A sevoflurane‐specific Penlon Sigma Delta vaporizer. The Sigma Delta is also available for the delivery of enflurane, halothane, and isoflurane. Source: Dr. Craig Mosley, with permission. This vaporizer (Fig. 6.54) has been available for veterinary use and was commonly employed on human anesthesia machines for many years. It is no longer being manufactured but is still readily found being redistributed through veterinary equipment companies. It is classified as variable‐bypass, flow‐over with wick, automatically temperature compensated, agent‐specific, VOC, and high‐resistance [76]. Specific units were manufactured for isoflurane, halothane, and sevoflurane administration. These vaporizers were designed for accuracy at FGFs between 0.3 and 10 L/min, and temperature compensation occurs between 16 and 32 °C. Tilting these vaporizers up to 20° while in use or up to 45° when not in use does not cause problems. Greater tipping of the vaporizer may cause delivery of high concentrations. Between paper wicks, the vaporizer has plastic spacers that may react with enflurane or isoflurane to cause discoloration of the liquid anesthetic, apparently without significant consequences [76]. Verni‐Trol and Copper Kettles are flowmeter‐controlled vaporizers that were once popular for use in anesthesia of human patients [76]. Although these vaporizers are no longer being manufactured, are not covered by the ASTM standards for anesthesia equipment, and are rarely found in clinical use (or otherwise), a description of their operation may be instructive for those wanting an alternative explanation of vaporizer function. Historically they are also important as they were the first devices to permit precise vaporization of liquid anesthetics and served as the precursors to today’s modern variable‐bypass vaporizers. Figure 6.54 An isoflurane‐specific Ohio vaporizer. It has also been manufactured for the delivery of halothane and sevoflurane. Source: Dr. Craig Mosley, with permission. These flowmeter‐controlled vaporizers are classified as measured‐flow, bubble‐through, high‐resistance, VOC, temperature compensated (thermally stable with manual flow adjustments based on temperature of the liquid anesthetic), and multipurpose. They have been classified as saturation vaporizers. These vaporizers are constructed of copper (Copper Kettle) or silicon bronze (Verni‐Trol) for thermostability. Back‐pressure compensation mechanisms are present on later production models and can be fitted on older models (e.g., check valves). Since these vaporizers are multipurpose or universal, they can accurately vaporize halothane, isoflurane, sevoflurane, or methoxyflurane, and should be clearly labeled for the agent in use. With measured‐flow vaporizers, manual adjustments of flow rates are required to account for variations in total gas flow, day‐to‐day changes in temperature, and changes in liquid temperature during use, especially with high FGF rates. In most cases, a calculator wheel is supplied with each vaporizer for determining proper flow rates. The user selects the total FGF, the desired % output, and the temperature of the inhalant, and the calculator will determine the flowrate through the vaporizer to achieve the desired output. Anesthesia machines with measured‐flow vaporizers have two oxygen flowmeters. One flowmeter routes all of its oxygen through the vaporization chamber, where it is fully saturated with anesthetic; the other flowmeter supplies oxygen that bypasses the vaporizer and supplies oxygen to meet the patient’s requirements. By manually altering the ratio of gas going to the vaporizer to that bypassing the vaporizer, precise concentrations of anesthetic could be delivered to the patient. Both gas sources combine at a mixing valve to achieve the proper anesthetic concentration before gases enter the breathing system. Modern agent‐specific variable‐bypass vaporizers simply eliminated the need to determine and adjust these ratios manually. Further details concerning the use of measured‐flow vaporizers can be found in earlier editions of this book [75]. Vaporizers are generally extremely robust and reliable but do require maintenance. In general, the best policy is to follow the manufacturer’s guidelines for care and servicing. Recommendations vary but, regardless of the maintenance schedule, servicing should be performed on a vaporizer if, based on the responses of patients, the dialed anesthetic concentration is suspected to be erroneous, or if any of the components of the vaporizer function improperly (e.g., the control dial is difficult to adjust). Servicing as recommended by the manufacturer often includes an evaluation of operation, cleaning, changing of filters, replacement of worn parts, and recalibration. Halothane and methoxyflurane contain preservatives (thymol and butylated hydroxytoluene, respectively) that are not highly volatile and therefore collect in the vaporization chambers and on the wicks, potentially affecting anesthetic output. These vaporizers should be periodically drained to remove most of these preservatives. Vaporizers should not be overfilled or tipped significantly once filled. They should also be drained before removal from the anesthesia machine for service. In the past, flushing a vaporizer with diethyl ether to dissolve preservatives that collect within it has been recommended. Owing to the flammability and explosiveness of diethyl ether, and the common use of 100% oxygen as a carrier gas, this is no longer recommended. Flushing the vaporizer does not eliminate the need for regular service by a certified vaporizer technician. Using an agent‐specific vaporizer for an anesthetic for which the vaporizer is not calibrated is problematic, especially if the introduction of an anesthetic is unintentional (i.e., the operator does not realize the mistake). Lower than indicated anesthetic output is expected if an anesthetic with a lower vapor pressure is placed in a vaporizer designed for a drug with a higher vapor pressure (e.g., sevoflurane used in an isoflurane vaporizer). Conversely, a highly volatile anesthetic used in an agent‐specific vaporizer designed for a drug with a lower vapor pressure is likely to produce a high, potentially lethal concentration. The differential potencies of the drugs in question would be expected to affect the depth of anesthesia in either situation. During the introduction of isoflurane into veterinary anesthesia, it was commonly administered with agent‐specific halothane vaporizers that were relabeled, but not recalibrated for isoflurane. Because the vapor pressures of halothane and isoflurane are similar (e.g., 243 and 240 mmHg at 20 °C, respectively), the output was not expected to differ greatly from the control‐dial setting. Indeed, halothane vaporizers produce concentrations of isoflurane that are reasonably close to the dial setting for halothane [77]. Nevertheless, current manufacturer recommendations are against the use of isoflurane in halothane‐specific vaporizers and vice versa [78]. Depending on the vaporizer and conditions of operation, isoflurane in a halothane vaporizer may produce 25–50% more vapor than expected, and halothane in an isoflurane‐specific vaporizer usually delivers a concentration that is lower than expected [78]. If isoflurane is to be used in an agent‐specific halothane vaporizer, the vaporizer should be serviced and completely recalibrated for isoflurane. Complete calibration implies that the vaporizer has been tested for accuracy with an anesthetic‐gas analyzer at various carrier gas flow rates and various temperatures to assure reliable function. Oxygen flush valves are found on most, but not all, veterinary anesthetic machines. There is no convention regarding location, size, or design of the flush valve in veterinary medicine and they vary dramatically (e.g., button, toggle, or switch) (Fig. 6.55). Flush valves on human anesthetic machines must be a recessed button type and easily accessible on the front of the machine. Flush valves are designed to rapidly deliver large volumes of non‐anesthetic‐containing gas to the patient breathing circuit in emergency situations. The flow originates downstream of the regulator within the intermediate pressure area of the anesthetic machine (~50 psi) and bypasses the flowmeter and vaporizer, delivering gas at rates ranging between 35 and 75 L/min to the patient circuit. To avoid over‐pressuring the patient circuit, the flush valve should not be used, or be used very cautiously, with non‐rebreathing circuits, circuits attached to mechanical ventilators, and circuits with very low volumes (e.g., pediatric circle systems) as pressures within the breathing circuit may temporarily rise, creating dangerously high pressures to the patient’s lungs. The adjustable pressure‐limiting (APL) valve should be fully open at all times to help prevent over‐pressurizing the breathing circuit. The common gas outlet leads from the anesthetic machine to the breathing circuit (Fig. 6.56). Gas reaching the common gas outlet has traveled from the gas supply (cylinder or pipeline), through the regulator, flowmeter, and vaporizer. The gas flowing from the common gas outlet normally delivers the anesthetic and carrier gas(es) to the patient circuit at the concentration and flow rate determined by the vaporizer setting and flowmeter flow rate. However, the concentration of inhalant gas from the common gas outlet is not usually equivalent to the gas concentration inhaled by the patient when using rebreathing circuits, particularly when using low flow rates, due to dilution of incoming gases with those already in the patient circuit. The exact configuration of the common gas outlet varies among manufacturers, but it is normally a taper 15 mm ID port. Human anesthesia machines must have a locking mechanism in place to prevent inadvertent disconnection from the machine (Fig. 6.56), but this is not a requirement in veterinary medicine. However, at least one company designs their fresh gas outlet with a quick‐connect system to prevent inadvertent disconnections (Fig. 6.57). Figure 6.55 Examples of various flush valve buttons on anesthetic machines. A. The oxygen flush button is recessed in a protective collar to prevent inadvertent activation if bumped. B. Many oxygen flush buttons are relatively small and are unprotected from accidental activation. Source: Dr. Craig Mosley, with permission. Figure 6.56 Common gas outlets deliver gas from the anesthetic machine to the patient breathing circuit. A. Push‐type taper connections are common in veterinary medicine. B. Locking common gas outlets is standard on human anesthesia machines. Locking systems will help prevent inadvertent disconnection of the gas supply from the machine to the breathing circuit. Source: Dr. Craig Mosley, with permission. Figure 6.57 A quick‐connect/disconnect system used for the common gas outlet on a machine designed for veterinary use can be a safety feature preventing inadvertent disconnections between the patient circuit and the anesthesia machine. Source: Dr. Craig Mosley, with permission. Although some parts of the breathing system are built into anesthesia machines (e.g., the circle system carbon dioxide absorbent canister), breathing systems are considered separately from the actual anesthesia machine (i.e., components upstream of the fresh gas outlet). This is a convenient way to discuss breathing systems in veterinary medicine as on any single anesthetic machine the breathing system may be frequently changed depending upon the needs of the patient or the circumstances in which anesthetic is delivered (Fig. 6.58). The primary purposes of the breathing system are to direct oxygen to the patient, deliver anesthetic gas to the patient, remove carbon dioxide from inhaled breaths (or prevent significant rebreathing of carbon dioxide), and provide a means of controlling/supporting ventilation. Breathing systems have been classified using numerous schemes with little uniformity or consensus of nomenclature (i.e., open, semi‐open, and semi‐closed). For this reason, it is suggested that these terms be abandoned and rather a description of the flow rates and breathing system (e.g., circle system and Mapleson D system) are all that is needed. For clarity, it is easiest to classify the breathing circuits into one of two groups: those designed for rebreathing of exhaled gases that contain a means to absorb carbon dioxide (rebreathing or partial rebreathing system) and those designed to be used under circumstances of minimal to no rebreathing that do not contain a means to absorb carbon dioxide (non‐rebreathing systems). Some have argued that this classification is somewhat of a misnomer since, depending upon the specific system used and the FGF rates used, a rebreathing system may have minimal rebreathing occurring (i.e., excessively high FGFs) or a non‐rebreathing system may not completely prevent rebreathing (i.e., inadequate/low FGF). To help circumvent this debate, it has been suggested that in addition to describing the design of the breathing circuit, the FGF rate should be provided to describe fully how the system is being used [79]. The FGF rate should be expressed in mL/kg/min in veterinary medicine owing to the vast range of patient sizes encountered. Additionally, the degree of rebreathing with breathing circuits can be affected by other factors such as the equipment deadspace and the patient’s respiratory pattern. Breathing systems have intentionally been designed to function as rebreathing (contain means to absorb carbon dioxide) or non‐rebreathing systems (no means to absorb carbon dioxide) and should be used in the manner for which they were originally intended. Another way to classify or describe breathing systems is by their means of preventing CO2 rebreathing. A non‐rebreathing system is entirely flow dependent for the prevention of rebreathing of CO2, whereas a rebreathing system is flow independent, in that regardless of the FGF rate there should be no rebreathing as long as all components of the system (one‐way valves, CO2 absorber) are functioning properly. Figure 6.58 An example of an anesthetic machine mounted with both circle (left) and Bain (right) breathing circuits. Changing gas flow between the circuits is achieved through use of a switch handle located on the lower right front of the machine. There is no obvious common gas outlet on this machine as it is integrated into the breathing circuit toggle switch. Source: Dr. Craig Mosley, with permission. The circle system is the most commonly used rebreathing system and will be the only one described here, although other types of rebreathing systems have been used (e.g., to‐and‐fro). Descriptions of these rarely used systems can be found in earlier editions of this book [75]. The circle system is designed to produce a unidirectional flow of gas through the system and has a means of absorbing carbon dioxide from exhaled gases and its ability to remove CO2 is independent of the FGF rate. The components of the circle system include a fresh gas inlet, inspiratory one‐way valve, breathing tubes, expiratory one‐way valve, APL valve, reservoir bag, and carbon dioxide absorber (see Fig. 6.38). The FGFs used with a circle system determine the amount of rebreathing; full rebreathing (closed), partial rebreathing (semi‐closed or low flow), and minimal rebreathing. Historically, many terms have been applied to describe the amount of rebreathing, but there is no universally accepted standard or description of these terms. However, it has been suggested by several authors that the use of the terms “open,” “semi‐open,” and “semi‐closed” should be dropped to avoid confusion [80]. This describes a circle system using flow rates equal to, or nearing, the metabolic oxygen consumption of the patient, between 3 and 14 mL/kg/min. This is sometimes also described as a closed system, but it may be best to avoid using such terms to avoid confusion. Note that in a “closed system,” the APL is not normally closed, as it could create a potentially dangerous situation (increasing pressure within the patient circuit) should FGF rates exceed metabolic oxygen requirements. This generally describes a circle system using a flow rate greater than metabolic oxygen consumption (e.g., 20 mL/kg/min) but less than that required to prevent rebreathing. Since this is a very large range, it is often divided arbitrarily into low flow (20–50 mL/kg/min), mid (“so‐so”) flow (50–100 mL/kg/min), and high flow (100–200 mL/kg/min), although this is not a universally accepted description. This describes a circle system using flow rates greater than 200 mL/kg/min (flow rates that would normally not be used in most circumstances). Such unusually high flow rates may result when circle systems are used for maintenance of anesthesia in very small patients (< 5 kg) with flow rates of 1000 mL/min or greater. Frequently in veterinary medicine, it is suggested that flow rates below 1000 mL/min should not be used. Although this recommendation may be clinically useful for preventing some anesthetic delivery errors, most modern anesthetic systems (i.e., vaporizers) continue to function optimally down to flow rates of 200–500 mL/min. With the availability of low‐volume, low‐deadspace pediatric and neonatal circle systems, coupled with improved patient monitoring (pulse oximetry and end‐tidal carbon dioxide), it is common to use circle systems with partial rebreathing flow rates (i.e., < 1000 mL/min) in small patients (< 5 kg). It is most economical in terms of both oxygen and inhalant anesthetic use to employ low flow rates when possible. Lower flow rates are also associated with less environmental contamination by halogenated hydrocarbons (all commonly available inhaled anesthetics) and marginally improved maintenance of body temperature. However, lower flow rates are also associated with decreased anesthetic gas delivery (unless higher vaporizer settings are utilized). Additionally, when low carrier gas flows are used (relative to the volume of the circuit), the time required to change the anesthetic concentration within the circuit significantly increases. Most inhalant anesthetics are delivered initially using relatively high FGF rates (e.g., 50–100 mL/kg/min for dogs) to facilitate rapid increases in the inspired inhalant concentration within the breathing system and to replace the anesthetic vapor that is dissolving into patient tissues during the initial uptake period of inhalant anesthesia delivery. The flow rates are then decreased (e.g., to 20–50 mL/kg/min for dogs) after the initial uptake period (e.g., the first 10–20 min) to economize on gas use and waste. The initial and maintenance recommended flow rates (mL/kg/min) often used clinically tend to be much higher in smaller patients (e.g., cats) relative to larger patients (e.g., horses). Using higher flow rates ensures that the patient’s inspired gas concentration is more reflective of the concentration of anesthetic gas delivered from the vaporizer. This is in contrast to using low FGF rates, where the inspired patient anesthetic concentration will not necessarily reflect the vaporizer concentration of gas until nearing equilibration. The use of anesthetic agent analyzers to monitor inspired and expired anesthetic partial pressures greatly facilitates decision‐making with respect to vaporizer settings and timing changes in gas flows when using lower carrier gas flows. The interaction between the vaporizer output, circuit volume, patient size, and flow rate is often an unfamiliar and difficult concept to grasp. Equating anesthetic delivery to a constant rate infusion of an intravenous drug is perhaps a more familiar comparison for understanding inhalant anesthetic delivery. The configuration and features of available circle systems vary somewhat depending upon the manufacturer, but in general, a common pattern of gas flow is followed through the fresh gas inlet, the inspiratory one‐way valve, the inspiratory and expiratory breathing tubes (into and out of patient), the expiratory one‐way valve, the APL valve, the reservoir bag, and the carbon dioxide absorbing canister back to the fresh gas inlet (Fig. 6.59). Some circle systems may also have additional incorporated features such as a switch to automatically engage the mechanical ventilator and oxygen sensor ports (Fig. 6.60). The fresh gas inlet is the site of gas delivery to the circle system from the common gas outlet of the anesthetic machine. The fresh gas inlet is normally found after the carbon dioxide absorber and before the inspiratory one‐way valve. Figure 6.59 Examples of various circle rebreathing circuits used in veterinary medicine. The degree of integration into the anesthetic machine and added details vary markedly among manufacturers but the essential features and functions are similar. Source: Dr. Craig Mosley, with permission. Figure 6.60 An example of a circle breathing system on an anesthetic machine with a built‐in ventilator that uses a switch to engage the ventilator circuit. This can minimize the potential for misconnections, disconnections, or kinked hoses during ventilator set up. Source: Dr. Craig Mosley, with permission. During inspiration, the inspiratory one‐way valve opens, allowing gas to move from the fresh gas inlet and reservoir bag past through the one‐way inspiratory valve into the inspiratory limb of the breathing circuit. These valves normally consist of a clear dome (for direct visualization of valve function), a light‐weight valve, and a valve housing (valve seat and valve guides). The valves are normally accessible for cleaning and repair through a removable cover. During expiration, the inspiratory valve is closed, preventing exhaled gas from entering the inspiratory limb of the breathing circuit, and forcing it into the expiratory limb of the breathing circuit. At least one system (Matrx® circle system, Midmark Animal Health, Versailles, OH, USA) also incorporates a negative‐pressure relief valve, providing an alternative path of gas flow (room air) to the patient should the inspiratory valve become stuck in the closed position (Fig. 6.61). The most basic breathing circuit is made up of a corrugated plastic or rubber inspiratory and expiratory limbs. The corrugated tubing helps prevent kinking and allows for some expansion if the breathing circuit is subjected to compression or traction. The two breathing limbs are connected via a Y‐piece that connects to the endotracheal tube or facemask. There are also various coaxial designs that place the inspiratory limb within the expiratory limb of the breathing circuit. Coaxial systems reduce the bulk associated with the breathing system and (at least theoretically) facilitate warming the inspired gases by the expired gases. The Universal F‐circuit (a coaxial breathing system) is designed to function with standard circle systems (e.g., 22 mm OD connectors of the circle system). Most breathing circuits are adapted for use with all circle systems as the fitting diameters are standardized. However, there is a proprietary coaxial circuit available that utilizes non‐standard‐sized circle system connectors requiring the use of a proprietary circle system (Moduflex Coaxial Breathing Circuit, Dispomed, Joliette, QC, Canada). There are also several sizes of breathing circuits available that vary in length, diameter, volume, and the amount of deadspace to meet various anesthetic requirements. Pediatric and neonatal rebreathing circuits are normally low‐volume and low‐deadspace systems, allowing them to function optimally in small patients (i.e., those with small tidal volumes) (Fig. 6.62). Figure 6.61 Examples of negative‐pressure relief valves that are incorporated into some circle systems. Should the inspiratory valve become occluded or stuck, the negative‐pressure relief valve is activated, allowing the patient in inhale air from the room. Figure 6.62 The Y‐piece of three different‐sized circle system circuits demonstrating the difference in deadspace. Circle system circuits are commonly available in neonatal/pediatric and adult configurations (middle and right, respectively). The system on the left is a veterinary‐specific circuit designed specifically for very small patients and has minimal deadspace associated with its use. Source: Dr. Craig Mosley, with permission. The expiratory one‐way valve functions together with the inspiratory one‐way valve, closing upon inspiration and opening during expiration. This valve helps direct gas into the expiratory limb of the breathing system, through the expiratory valve, and into the reservoir bag. The reservoir bag is also referred to as a “breathing bag” or “rebreathing bag”. The purpose of the reservoir bag is to provide a compliant reservoir of gas that can rapidly change volume with the patient’s expiration and inspiration. It is commonly recommended that the reservoir bag has a volume that is approximately 5–10 times the patient’s normal tidal volume (10–20 mL/kg) or roughly equivalent to the patient’s minute volume. Ultimately, the reservoir bag should be large enough to provide a reasonably sized reservoir of gas but not so large that it becomes difficult to observe movements of the bag associated with breathing. In addition, a very large reservoir bag will contribute to the overall functional volume of the rebreathing system (i.e., circle system), contributing to slower rates of change in anesthetic concentration within the breathing system when the vaporizer output is altered. This would not be the case when using a non‐rebreathing system. The APL valve is also commonly referred to as the “overflow,” “pop‐off,” or “pressure relief valve.” The APL valve is a safety valve allowing excess gas to escape from the patient circuit. If the valve is functioning properly, gas should escape if system pressures exceed 1–3 cmH2O. Normally, it should be left fully open at all times unless positive‐pressure ventilation is being used, and then it should be immediately reopened when ventilation ceases to prevent excessive pressure from building in the patient circuit. The APL valve is partially closed in some instances to prevent collapse of the reservoir bag due to the negative pressure/vacuum from the scavenge system. Although this can remedy the situation, it is strongly discouraged as it can lead to partial or full closure of the valve, leading to excessive pressure building up in the patient circuit. If the reservoir bag continually collapses under normal use, this indicates the need to adjust the central vacuum/scavenge system and or the need to incorporate a properly functioning scavenge interface to offset this effect. Several manufacturers market products that allow intermittent closure of the APL system. These devices allow temporary closure of outflow to the scavenge system when a button or the valve itself is depressed. These momentary closure systems are built directly into some APL valves or can be added to currently used APL valves (Fig. 6.63). These are extremely valuable additions to the APL valve system and help to prevent excessive pressure associated with an inadvertently closed APL valve that can lead to patient barotrauma or death. They also greatly facilitate the ease and safety of manual IPPV. Figure 6.63 A. An example of a momentary closure valve built into the adjustable pressure‐limiting (APL) valve. B. Momentary closure valve that can be added to any standard APL valve. C. Demonstration of the use of a momentary occlusion valve to deliver a breath to a patient. Source: part A., Supera Anesthesia Innovations, Clackamas, OR, USA; with permission. Source: Parts B. and C., Dr. Craig Mosley, with permission. The carbon dioxide absorber contains the chemical absorbent for removing carbon dioxide from exhaled gases. There are many types (dual canister, disposable, etc.) and sizes of carbon dioxide absorbers available (Fig. 6.64). All contain some type of screen to prevent absorbent granules from entering the breathing circuit and most contain a baffling system to prevent channeling of gases within the absorber canister. However, despite the screens, the absorbent granules and/or dust will occasionally enter the breathing circuit. This is probably most commonly encountered in large animal systems, where relatively high peak flows of gas (associated with inspiration and expiration) are more common. Gas flow patterns within canisters vary considerably, but canister design normally attempts to ensure optimal and efficient absorbent use. It is frequently suggested in veterinary medicine that the absorber canister must be twice the patient’s tidal volume to ensure complete absorption of carbon dioxide, but there seems to be little evidence to support this statement. Most large animal machine canisters rarely have a volume equal to twice the patient’s tidal volume and may in fact have a volume less than the patient’s tidal volume. Moreover, many carbon dioxide absorbers used in human anesthetic machines have volumes less than the patient’s tidal volume. Smaller canisters are often preferred to ensure more frequent changing of the absorbent, lessening the likelihood that toxic byproducts will be produced by desiccated absorbent. However, the relative efficiency of absorption (i.e., the carbon dioxide load absorbed when an absorbent appears exhausted) may improve with larger carbon dioxide absorbers [4]. Smaller carbon dioxide absorbers will reduce the internal volume of the breathing circuit, leading to vaporizer concentration changes being reflected more rapidly in the inspired gas concentration, but will require more frequent absorbent changes. Figure 6.64 Three different absorber canisters. The canister on the far left is a bulk flow‐through type canister where the exhaled gas from the patient circuit enters one side (i.e., top) of the canister and exits the other (i.e., bottom) upon inspiration. The two canisters on the right utilize a conducting tube to direct the exhaled or inhaled gases in a predictable pattern through the canister. Gas must enter and exit the canister from the same opening (i.e., the top) through different paths. Source: Dr. Craig Mosley, with permission. The basic chemistry of carbon dioxide (CO2) absorbents relies on an exothermic reaction combining CO2 with calcium hydroxide (Ca(OH)2) to form calcium carbonate (CaCO3) and water (H2O). Strong bases such as the metal hydroxides, sodium hydroxide (NaOH), potassium hydroxide (KOH), and barium hydroxide (BaOH), are added as catalysts to enhance the speed of the reaction and to increase the capacity to absorb CO2. Absorbent brands are largely distinguished by the type and relative concentrations (3–20%) of strong bases (metal hydroxides) added to the Ca(OH)2. There is also at least one absorbent, Amsorb® Plus (Armstrong Medical, Londonderry, UK) that contains no strong base. The general chemical reaction when the strong base NaOH is present as the catalyst is as follows.
6
Anesthesia Equipment
Introduction
Safety and design
Introduction to airway management and support equipment
Endotracheal tubes, lung isolation devices, supraglottic airway devices, laryngoscopes, intubation aids, and techniques
Endotracheal tubes
Endotracheal tubes for isolating one lung
Supraglottic airway devices
Laryngoscopes
Intubation aids and techniques
Nasotracheal intubation
Wire‐ or tube‐guided techniques
Endoscope‐guided technique
Endotracheal tube exchangers
Retrograde intubation
Tracheostomy
Lateral pharyngotomy
Techniques of oxygen administration
Mask delivery
Technique
Approximate FIO2 obtainable (%)
Flow rate
Flow by oxygen
25–40
0.5–5 L/min
Face mask
35–60
2–8 L/min
Nasal insufflation
30–70
100–150 mL/kg/min
Tracheal insufflation
40–60
50 mL/kg/min
Oxygen cages
25–50
Variable
Nasal insufflation
Tracheal insufflation
Oxygen cages
Oxygen toxicity
Introduction anesthetic machines and breathing circuits
Medical gas supply
Size
Gas
Gas symbol
Color code (US)
Color code
(ISO)
Capacity and pressure
(at 70 °F)
Empty cylinder weight (pounds)
E
Oxygen
O2
Green
White
660 L
1900 psi
14
E
Nitrous oxide
N2O
Blue
Blue
1590 L
745 psi
14
G
Nitrous oxide
N2O
Blue
Blue
13,800 L
745 psi
97
H
Oxygen
O2
Green
White
6900 L
2200 psi
119
H
Nitrous oxide
N2O
Blue
Blue
15,800 L
745 psi
119
Medical gas safety
Color coding
Diameter index safety system
Quick connectors
Pin index safety system
Pressure‐reducing valve (regulator)
Pressure gauges
The modern anesthetic machine
Gas flow within the anesthetic machine
Flowmeters
Vaporizers
Temperature compensation
Flow rate compensation
Back‐pressure compensation
Operational considerations
Descriptions of vaporizers common in veterinary medicine
Ohmeda/Datex‐Ohmeda/GE Healthcare Tec vaporizers
Tec 3 vaporizers
Tec 4 and 5 vaporizers
Tec 6
Dräger vapor vaporizers: Vapor 19.1
Penlon Sigma Delta vaporizers
Ohio calibrated vaporizer
Measured‐flow vaporizers
Maintenance of vaporizers
Use of the wrong anesthetic in an agent‐specific vaporizer
Oxygen flush valve
Common gas outlet
Breathing systems
Rebreathing (circle) system
Full (complete) rebreathing system
Partial rebreathing system
Non‐(minimal) rebreathing system
Fresh gas inlet
Inspiratory one‐way valve
Breathing circuit tubing
Expiratory one‐way valve
Reservoir bag
Adjustable pressure‐limiting valve
Carbon dioxide absorber
Carbon dioxide absorbents
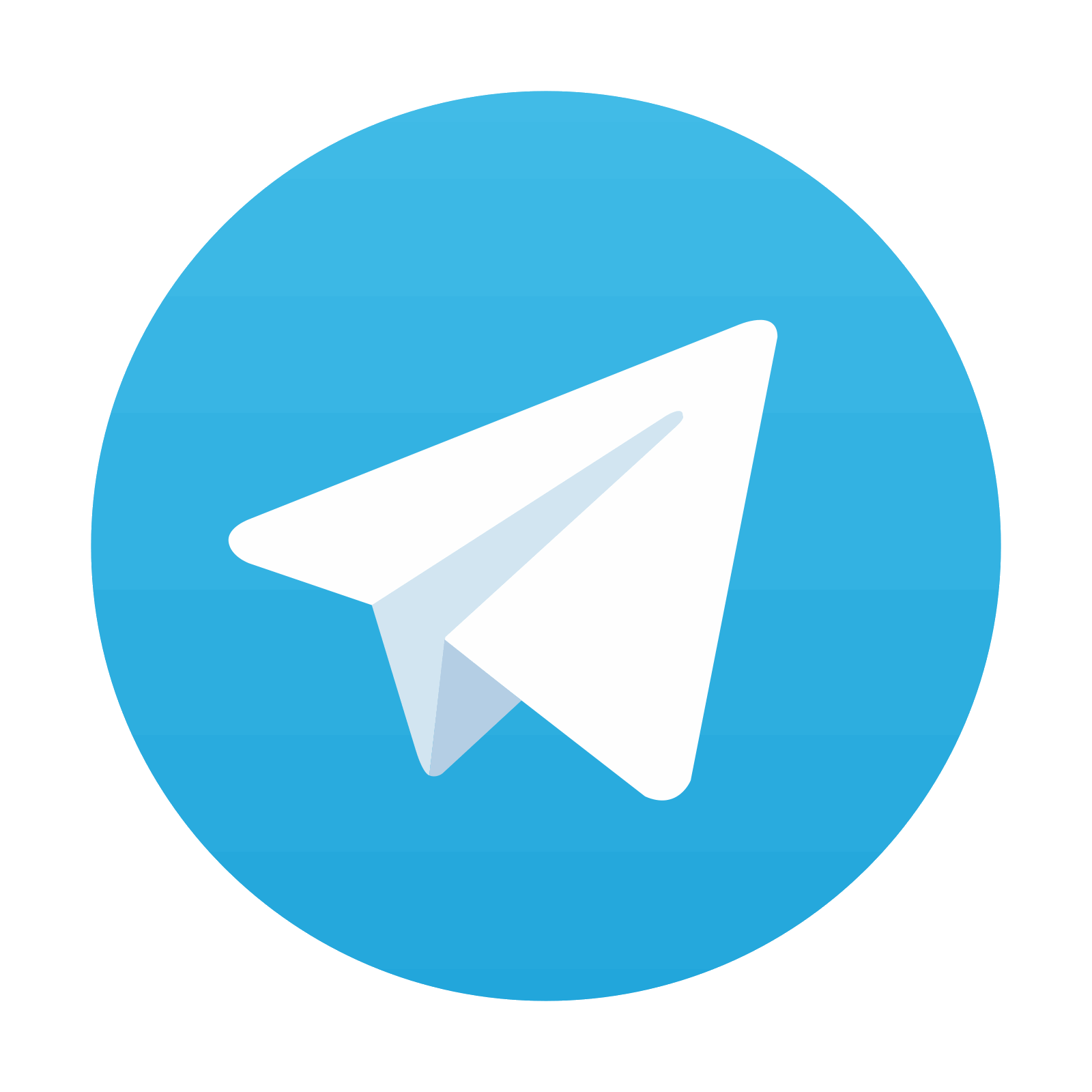
Stay updated, free articles. Join our Telegram channel
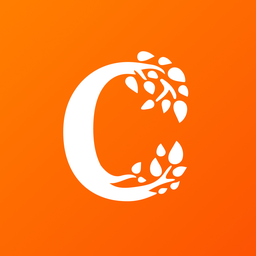
Full access? Get Clinical Tree
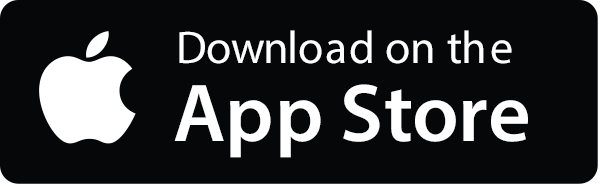
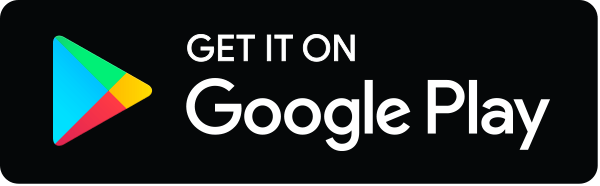