Introduction
Inhalant anesthesia forms the basis of most modern anesthetic protocols in veterinary medicine. The administration of potent inhaled anesthetics requires specific delivery techniques. The anesthetic machine enables the delivery of a precise yet variable combination of inhalant anesthetic and oxygen. The basic components and functions of all anesthetic machines are similar but significant design differences exist among them. Machines can be very simple, for example, those used for mobile applications to very complex anesthetic workstations with built-in ventilators, monitors, and safety systems (see Figure 5.1). Regardless of the complexity of the design, all anesthetic machines share common components: a source of oxygen, a regulator for oxygen (this may be part of the gas supply system), flow meter for oxygen, and a vaporizer. If additional gases are used (i.e., nitrous oxide) there will also be a source, regulator, and flow meter for each gas that generally parallels the path of oxygen with some exceptions (i.e., oxygen flush valve). The basic anesthetic machine is then used in conjunction with a breathing circuit and anesthetic waste gas scavenging system for anesthetic delivery to the patient.
Since 2000, human anesthetic machines sold in North America must meet minimum design and safety standards established by organizations such as the American Society for Testing and Materials (ASTM) and the Canadian Standards Association (CSA). Anesthetic machines designed for veterinary use are not required to meet any specific design or safety standards beyond those associated with basic hazards to the operator (i.e., electrical safety requirements). Safety features are often added on an ad hoc basis and there are no requirements for demonstrating equipment efficacy. Ideally, safety features, such as airway pressure alarms, should be designed into the anesthetic machine itself. The inclusion of some of these safety systems on anesthetic machines may help eliminate preventable anesthetic accidents. However, until safety and design standards are adopted by the manufacturers of veterinary anesthetic equipment, there will remain numerous equipment options of varying quality, efficacy, and safety available for delivering inhalant anesthetics to veterinary patients. Regardless of the presence of standards, it will always be incumbent upon the veterinary anesthetist to thoroughly understand the function of each component of the anesthesia machine and to ensure that the machine is designed suitably well to accomplish these tasks safely.
Figure 5.1. Anesthetic machines for veterinary use can vary considerably in their complexity and sophistication. (A) A complete veterinary anesthetic workstation for large animal use. (B) A portable anesthetic system for field use. Both systems provide all the components necessary for the controlled delivery of inhalant anesthetics. Photo A courtesy of Hallowell EMC, Pittsfield, MA.

It is beyond the scope of this chapter to describe in detail all the anesthesia machines and equipment currently available and used in veterinary medicine. There are many excellent textbooks devoted to describing in great detail the anesthetic equipment available for use in human anesthesia.1,2 This chapter aims to provide the reader with a general working overview of the anesthetic machine, vaporizers, breathing circuits, and ventilators. In addition, there are products that have been designed specifically for veterinary use that are not described elsewhere, and some of these will be described here.
Endotracheal tubes and laryngoscopes
Endotracheal tubes
Endotracheal tubes are commonly used to maintain an airway in patients anesthetized with inhalant anesthetics. However, laryngeal masks have been evaluated in a number of domestic species and may be suitable alternatives in some instances.3–6 A properly placed endotracheal tube or laryngeal mask with a properly inflated cuff provides a patent airway, facilitates positive pressure ventilation, protects the airway from aspiration of fluids, and prevents contamination of the work environment with waste anesthetic gases.
There are many styles and types of endotracheal tubes available that can be used in veterinary medicine (Figure 5.2A). Most are manufactured for humans but can be used in most small animal patients. For patients requiring tube sizes larger and smaller than those available for human use, there are some veterinary-specific products available. Endotracheal tubes manufactured for use in humans must have various markings and abbreviations directly on the tube that fully describes each tube. The markings may include the manufacturer, internal (ID) and outer (OD) tube diameter, length, and codes indicating tissue toxicity or implantation testing (e.g., F29) (Figure 5.2B). There is no requirement for similar markings on tubes manufactured solely for veterinary use but it is common for them to minimally list tube diameters and length. Endotracheal tubes are normally sized according to their IDs. For example, a size 6.0 endotracheal tube refers to a tube with an ID of 6.0 mm. The OD for any given tube size may vary depending upon the construction of the tube. Endotracheal tubes having thicker walls will have greater differences between the IDs and ODs. This can become important when selecting tubes for very small patients. Very thick-walled tubes will effectively reduce the internal airway diameter compared with a thin-walled tube, as ultimately the size of the endotracheal tube that can be placed in a patient is limited by the OD of the tube and not the ID. However, very thin -walled soft tubes are susceptible to obstruction by external compression (i.e., tube tie) or kinking (Figure 5.3).
Common endotracheal tubes materials include polyvinyl chloride, silicone, or red rubber. Clear endotracheal tubes are generally preferred so that they can be visually inspected for the presence of mucous or blood intraoperatively, or debris within the tube lumen after cleaning. Generally, the largest size endotracheal tube that will fit in the patient’ s trachea should be used. Although various “rules of thumb” for selecting tube size exist, it is probably easiest to estimate the most appropriate tube size by palpating the individual patient’ s trachea. The tube should not extend distally beyond the thoracic inlet and ideally should not extend rostrally significantly beyond the patients incisors, as this will increase mechanical dead space. If the endotracheal tube is too long and further insertion would lead to the possibility of endobronchial intubation, it can be cut and the endotracheal tube connector replaced.
The most commonly used type of endotracheal tube in both large and small animals is the cuffed Murphy-type tube shown in Figure 5.2B. Cole-type and guarded (spiral embedded, armored) tubes are also occasionally used in veterinary medicine. Cole tubes are an uncuffed tube that has a smaller diameter at the patient (distal) end relative to the machine (proximal) end. The distal smaller diameter portion of the tube is inserted into the trachea to a point where the shoulder contacts the larynx, forming a seal. However, Cole tubes will not produce the same degree of airway security compared with a standard cuffed tube and are normally only used in very small patients for short-term intubation (see Figure 5.4). Guarded tubes incorporate a metal or nylon spiral reinforcing wire into the endotracheal tube wall that helps prevent tube collapse and occlusion. Guarded tubes are useful in situations where the tube is likely to be compressed or kinked, such as procedures requiring extreme flexing of the head and neck (i.e., cerebrospinal fluid [CSF] taps) or those that involve compression of the trachea (i.e., tracheal retraction during ventral approach to the cervical spinal cord).
Figure 5.2. (A) The tubes include tubes made of silicone, polyvinyl chloride, and red rubber (top to bottom). (B) Diagram illustrating the parts and desirable characteristics (e.g., radius of curvature and angle of the bevel) of a Murphy endotracheal tube. OD, outer diameter. From Dorsch J.A., Dorsch S.E. Tracheal tubes. 1994. In: Understanding Anesthesia Equipment, 3rd ed. J.A. Dorsch and S.E. Dorsch, eds. Baltimore, MD: Williams and Wilkins, p. 439.

Figure 5.3. Very thin-walled endotracheal tubes are prone to occlusion from external compression or twisting. Continual evaluation of the endotracheal tube for patency is required when thin pliable walled endotracheal tubes are used.

Figure 5.4. A 10-French Cole endotracheal tube appropriate for small veterinary patients. Note the smaller diameter of the laryngotracheal portion of the tube (distal end of the tube, right side of the figure).

The machine end contains the endotracheal tube connector. The most proximal portion of the connector is a uniform size (15 mm OD) facilitating universal connection to all standard anesthetic circuits. The distal end of the connector varies in size according to the diameter of the endotracheal tube.
The patient (distal) end of the endotracheal tube is normally beveled. Murphy-type tubes have a hole in the endotracheal tube wall opposite the bevel, referred to as a Murphy eye or hole. The purpose of the hole is to provide an alternate route for gas flow should the beveled opening become occluded. Endotracheal tubes without a Murphy eye are referred to as Magill -type tubes. The cuff is located rostral to the Murphy eye for cuffed tubes, and can be a low-volume, high-pressure or high-volume, low-pressure design. In general, high-volume, low-pressure cuffs are preferred to minimize the risk of ischemic tracheal injury that may result from excessive pressure against the tracheal wall. When a properly fitting endotracheal tube with a high-volume, low-pressure cuff is used, the pressure exerted by the cuff on the tracheal wall is similar to the intracuff pressure. This allows for better estimation of the anticipated pressure on the tracheal wall exerted by the cuff. When using a high-pressure, low-volume cuffed endotracheal tube, the intracuff pressure does not reflect the pressure on the tracheal wall but rather the pressure created by the elastic recoil of the cuff, making estimates of pressure exerted by the cuff on the tracheal wall difficult. Tracheal wall pressures exceeding 48 cm H2O may impede capillary blood flow, potentially causing tracheal damage, and pressure below 18 mm Hg may increase the risk of aspiration.7 The best method for ensuring cuff pressures are within the recommended range is to use a cuff monitor to inflate the cuff. A cuff monitor is essentially a low-pressure manometer similar to those used for Doppler blood pressure measurement that are attached to the pilot balloon of the cuff and provide a measure of intracuff pressure. Other commercially available cuff inflation guides are available for the human market and have been adapted to veterinary use (Figure 5.5). Alternatively, it is common to use a leak test, performed by inflating the cuff until a leak is no longer audible at airway pressures of 20–30cmH2O. The pilot balloon used for inflating the endotracheal tube cuff is connected to the cuff via a channel incorporated into the endotracheal tube and normally includes a syringe-activated self-sealing valve system. However, there are also pilot balloons that do not self-seal and require some sort of manual occlusion using either a clamp or plug.
Laryngoscopes
Laryngoscopes consist of a handle and lighted blade; and are used to aid tracheal intubation and oropharyngeal evaluation during intubation. Unfortunately, laryngoscopes are often considered an optional piece of anesthetic-related equipment but their proper use can be vital for successful intubation in some patients (i.e., brachycephalics, patients with laryngeal/oral trauma). Regardless of the absolute need for laryngoscope-assisted intubation, its use is recommended for all intubations to ensure the anesthetist maintains the motor skills and coordination to properly use a laryngoscope and that a cursory oropharyngeal evaluation can be performed.
There are several styles and types of laryngoscopes and blades available. Some disposable laryngoscopes have a fixed blade (i.e., one blade type and size) and may be made of plastic while others are designed for use with multiple blade types and styles of blades and made of stainless steel. Because there is such a range of patient sizes, with different oral cavity configurations found in veterinary medicine, the option to use multiple blades is a significant advantage when selecting a laryngoscope. The handle may also vary in size and although this rarely impacts the functional use of the laryngoscope, a smaller handle may be more comfortable and easier to manipulate for some anesthetists, particularly when used for intubating very small patients. The handles are usually specific for either fiber-optic or bulb-in-blade illumination, although there are some handles that can accept either type of blade illumination system. There is no clear advantage of one lighting system over the other.
Figure 5.5. An endotracheal tube inflation guide or monitor can be used to evaluate the intracuff pressures of the endotracheal tube and may help avoid tracheal injury secondary to excessive tracheal wall pressures. Several styles are available, including (A) an incorporated manometer and (B) a visual indicator.

There are two main types of blades that are used in veterinary medicine, the MacIntosh and the Miller blade. Both come in a wide range of sizes (000–5). The MacIntosh is a curved blade with a prominent vertical flange, whereas the Miller is a straight blade with a less prominent vertical flange; both are suitable for intubation of most patients and the decision to use one over the other is often determined by personal preference. However, the prominent flange of the MacIntosh blade can potentially interfere with laryngeal visualization when used for intubating veterinary patients. In addition to the standard-sized blades available in human medicine, extremely long (∼ 300mm) Miller-style blades (useful for intubating swine, camelids, sheep, and goats) are also available (Figure 5.6).
Figure 5.6. Laryngoscope handle with Miller (upper) and MacIntosh (lower) blades. Note the more prominent vertical flange on the MacIntosh blade. This flange may impair visualization of the larynx when intubating a patient in sternal recumbency using the right hand.

Interestingly the majority of human-designed laryngoscope blades and endotracheal tubes are designed for anesthetists using their right hand to pass the endotracheal tube while their left hand holds the laryngoscope. The endotracheal tube bevel faces the left when viewing the tube from the concave aspect and the laryngoscope blade flange is normally on the right side of the blade when viewing the blade from the top. This configuration provides optimal visualization of the larynx when intubating a patient in the supine position (dorsal recumbency), where the laryngoscope is held with the blade in a downward position (inverted) and the endotracheal tube is held with the concave surface directed upwards. However, most veterinary patients are intubated in sternal recumbency, where the flange of the laryngoscope when held in the left hand can obscure visualization and the bevel of the endotracheal tube will do little to improve visualization when held in the right hand. There are left-handed MacIntosh blades available that may be more appropriate for intubation in veterinary species, as these blades place the flange on the left side of the blade, improving visualization of the laryngeal area when the laryngoscope is held in the left hand in an upright position. Since the Miller blade’ s flange is far less prominent, there is no real need for a left-handed design.
Anesthetic machines normally have two gas supplies, one from small high-pressure tanks attached directly to the machine and a second source often originating from a hospital’ s central pipeline supply. The small tanks mounted directly to the anesthesia machine are normally intended to be used as back up or reserve gas sources should the pipeline malfunction or for working in an area without access to the pipeline. Oxygen is by far the most commonly used medical gas during anesthesia, with nitrous oxide being used in conjunction with oxygen as an adjunct carrier gas for the inhalants much less frequently. Most medical gases are normally stored under high pressure in gas cylinders of various sizes or in low-pressure insulated cryogenic liquid bulk tanks. The characteristics (i.e., working pressure) and capacity of the gas cylinders varies with the type of gas they contain (see Table 5.1). Alternatively, oxygen concentrators can be used to supply a hospital with its oxygen requirements in circumstances where obtaining and storage of tanks is inconvenient, impossible, or prohibitively expensive (i.e., remote communities). Most oxygen concentrators use a system of absorbing nitrogen from air to produce gas with an oxygen concentration between 90% and 96%.
Most modern veterinary facilities will have some form of central gas supply and pipeline distribution system delivering medical gases to various work sites. The complexity of these systems can vary significantly; from a small bank of large (G or H) cylinders and a regulator to more complex systems consisting of multiple large liquid oxygen tanks, automatic manifolds, regulators, alarms, and banks of large high-pressure cylinders for back up. The size and complexity of the gas distribution system will depend upon the gas needs, area of required gas distribution, and the number of work sites required. Proper installation of large gas distribution systems is essential for safety and efficacy. All gas installations should be properly evaluated by those with expertise in this area prior to using them to deliver gas to patients.
Medical gas safety
There are several international (ASTM), national, and local documents related to the safe use, transport, and storage of pressurized gases. There are also standards surrounding the installation of medical gas piping systems and some of these provisions have been incorporated into hospital accreditation requirements in veterinary medicine. However, the specific guidelines can vary significantly among jurisdictions and regions. There have been several well-documented medical accidents related to the inappropriate use of medical gases. Consequently, there are several safety systems that have been developed to help reduce and eliminate these problems. For example, all anesthetic equipment has a gas-specific noninterchangeable connector that is part of the base unit (anesthetic machine, ventilator). These connectors — diameter index safety system (DISS), pin index safety system (PISS), and quick connector — are described below.
Table 5.1. Characteristics of medical gas cylinders

Color coding
Gas cylinders and gas lines are commonly colored coded to avoid improper use, but color coding systems can vary among countries. For example, oxygen is colored white in Canada and green in the United States. In addition to color coding, all tanks have a labeling scheme consisting of various shaped labels, key words, and colors that are all used to identify hazards associated with the gas they contain. Most tanks originating from gas supply facilities normally have perforated tags (full, in use, empty) to track the cylinder’s use status.
DISS
The DISS is a noninterchangeable, gas-specific threaded connection system. DISS is the gas connection used almost universally by all equipment and cylinder manufactures for the connection of medical gases.
Quick connectors
There are many proprietary (manufacturer specific) quick-connect systems that have been developed. These are standardized within a manufacturer but are not generally compatible with the quick-connect systems of another manufacturer. These systems facilitate rapid connecting and disconnecting of gas hoses and may be useful in situations where frequent connects and disconnects are required.
PISS
The PISS uses gas-specific pin patterns that only allow connections between the appropriate cylinder yokes and small gas cylinders (E size). The PISS is commonly found on the yokes mounted on anesthesia machines and some cylinder-specific regulator/flow meters.
Pressure reducing valve (regulator)
The pressure reducing valve (regulator) is a key component required to bring the high pressures of gas cylinders down to a more reasonable and safe working pressure (i.e., 35–50psi). Regulators also reduce or prevent fluctuations in pressure as the tank empties. Regulators are normally found wherever a high-pressure gas cylinder is in use (i.e., gas pipelines, cylinder connected directly to machine). The regulators used for pipelines are normally adjustable, whereas those on most anesthesia machines are set by the manufacturer. The ASTM standard requires that regulators on anesthesia machines be set to preferentially use pipeline gases before using gas from the backup cylinder on the anesthesia machine. However, since neither pipeline systems nor veterinary anesthesia machines are required to meet ASTM standards, it is not uncommon for machines to draw from the reserve or backup tank preferentially rather than the pipeline. This problem can be avoided by ensuring that the pipeline pressure is set approximately 5 psi higher than the anesthesia machines regulator for the reserve oxygen cylinder.
Pressure gauges
Pressure gauges are commonly used to measure cylinder pressures, pipeline pressures, anesthetic machine working pressures and pressures within the breathing system. Cylinder, pipeline and anesthetic machine working pressures are normally expressed in pounds per square inch (psi) or kiloPascals (kPa), whereas the pressures within the breathing system of the anesthetic machine are normally expressed in centimeters of water (cm H2O). The gauge measuring the pressure of the breathing system is often also referred to as a pressure manometer. The information provided by these gauges is vital for the safe operation of anesthesia equipment.
Gas flow within the anesthetic machine
The basic anesthesia machine is made up of a series of parts that work collectively to safely deliver inhalant anesthetics and support breathing. These components include: the basic anesthetic machine (gas delivery system), the vaporizer, the breathing circuit, and the waste gas scavenge system. Perhaps the simplest way to describe an anesthetic machine is to describe the components in order of the flow of gas through the machine, from source to patient. However, prior to describing these components, it is important recognize that the pressures of gas vary at different locations in an anesthesia machine and knowledge of these pressures facilitates the evaluation and safe operation of these machines. There are high-, intermediate-, and low-pressure areas. The high-pressure area accepts gases at cylinder pressure and reduces and regulates the pressure; this area includes gas cylinders, hanger yokes, yoke blocks, high-pressure hoses, pressure gauges, and regulators, and the pressure may be as high as 2200 psi. The intermediate-pressure area accepts gases from the central pipeline or from the regulators on the anesthesia machine and conducts them to the flush valve and flow meters; this area includes pipeline inlets, power outlets for ventilators, conduits from pipeline inlets to flow meters, and conduits from regulators to flow meters, the flow meter assembly, and the oxygen-flush apparatus. The pressure usually ranges from 40 to 55 psi. The low-pressure area consists of the conduits and components between the flow meter and the common gas outlet; this area includes vaporizers, piping from the flow meters to the vaporizer, conduit from the vaporizer to the common gas outlet, and the breathing system. The pressure in the low-pressure area is essentially equivalent to ambient pressure and the pressures being experienced by the patient. Pressures within the low-pressure area can vary depending upon how the system is being used (i.e., positive pressure ventilation) but should generally never exceed 30 cmH2O, as these pressures are transmitted directly to the patient’ s lungs (Figure 5.7).
Occasionally in veterinary medicine, multiple sources of medical gases (i.e., oxygen, air, nitrous oxide) are used with the anesthetic machine. However, 100% oxygen is normally the only gas used to deliver anesthesia and power anesthetic equipment (i.e., ventilators) in veterinary medicine. If the reader plans to use multiple gases for delivering anesthesia, it is the responsibility of the user to fully understand the implications of their use (indications and contraindications) and to ensure that the anesthetic equipment is properly designed and monitored to prevent the possibility of delivering a hypoxic gas mixture to the patient.
The flow of gas within an anesthetic machine may take multiple routes once it enters the intermediate-pressure areas of the anesthetic machine. Minimal gas must be delivered to the flow meter, where it is then directed to the vaporizer and subsequently to the patient. However, in addition to this route of movement, there may be several more routes available for gas distribution in the anesthesia machine. Normally on most anesthesia machines intermediate-pressure gas is also diverted to a fresh gas flush valve that bypasses the flow meter and vaporizer and delivers fresh gas directly to the breathing circuit. There are circumstances where flush valves may not be present or unavailable on veterinary anesthesia machines. Also, where present, gas from the intermediate pressure area may be diverted to one or more auxiliary oxygen outlets that may be used as the driving gas for a built-in or external ventilator or an oxygen flow meter.
Flow meter
Flow meters control the rate of gas delivery to the low-pressure area of the anesthetic machine and determine the fresh gas flow (FGF) to the anesthetic circuit. There must be a separate flow meter for each gas type used with the anesthetic machine. The type of breathing system used, the volume of the breathing circuit, and the size of the patient are all factors that influence the rate of FGF. There are several flow meter designs available but most are based on a tapered gas tube with a moveable float. The gas normally flows in the bottom of the tube and out the top. The tube is narrower at the bottom and wider at the top so as the float moves up the tube more gas can flow around the float, producing higher flow rates. The gas flow rates are normally expressed in liters per minute. The spatial distance between vertical markings on the flow meter does not necessarily correspond to equal changes in flow rate. In other words, the distance between 0 and 1000 mL as measured vertically on the flow meter may not be the same as the vertical distance between 1000 and 2000 mL. This is similar to the spatial separation of the percentages found on many vaporizers, where there is a greater spatial allocation on the dial for normal working percentages than for those rarely used. Some anesthetic machines may also have two flow meters for the same gas placed in series for allowing even greater precision at lower gas flow rates.
Figure 5.7. (A). Diagram of the basic anesthetic machine: the circle system. Exact position of the various components and specific features can vary markedly among manufacturers. Illustration by Kath Klassen, BSc (Agr), DVM. (B). Diagram of the basic anesthetic machine: the scavenge interface. Exact position of the various components and specific features can vary markedly among manufacturers. Illustration by Kath Klassen, BSc (Agr), DVM.


Vaporizer
Vaporizers change liquid anesthetic into vapor and control the amount of vapor leaving the vaporizer. Most modern vaporizers are agent specific, concentration-calibrated, variable -bypass, flow-over the wick, out of the circuit vaporizers that are compensated for temperature, flow, and back pressure. Nonprecision, low-resistance, in the circle vaporizers continue to be found in veterinary medicine, but without proper inspired inhalant gas monitoring these vaporizers would seem to pose unnecessary risks during anesthesia and their use should probably be discouraged. Vaporizers essentially work by splitting the carrier gas to flow into either the vaporizing chamber where it picks up anesthetic vapor or to the bypass channel where it does not. The ratio of the amount of gas that picks up inhalant to the gas that does not pick up inhalant, along with the vapor pressure of the volatile anesthetic, will determine the final concentration of the gas leaving the vaporizer. The output from the vaporizer is expressed as a concentration (e.g., volume percent) of vapor in the gas leaving the vaporizer.
Temperature, flow, and pressure are all factors that can potentially alter vaporizer output. The mechanisms for temperature, flow, and pressure compensation vary among vaporizer manufacturer and model. In general, most precision-compensated vaporizers will maintain consistent output at flows between 0.5 and 10 L/min, temperatures between 15 and 35° C, and pressure changes associated with positive pressure ventilation and the use of the flush valve.
Temperature compensation
Temperature compensation is achieved through using materials for vaporizer construction that supply and conduct heat, efficiently promoting greater thermostability. In addition, most vaporizers have mechanical thermocompensation systems. These vaporizers compensate for temperature changes by altering the splitting ratio so that a greater or lesser amount of gas is conducted through the vaporizing chamber as the temperature changes during use. As the vaporizer cools, the thermal element restricts gas flow to the bypass chamber, causing more carrier gas to enter the vaporizing chamber. The opposite will occur if the vaporizer becomes too warm. The exact thermocompensation systems vary among manufacturers but the thermal element is normally a heat-sensitive metal that will reliably expand and contract when subjected to temperature changes.
Flow rate
Changes in gas flow rate through the vaporizer could potentially lead to changes in output. For example, if the flow was excessively high, complete saturation of the gas moving through the vaporizing chamber may not occur, leading to a reduction in output. Flow rate compensation is achieved by ensuring reliable and consistent saturation of all gas flowing through the vaporization chamber by using a series of wicks, baffles, and spiral tracks to facilitate liquid gas vaporization. These techniques are used to increase the surface area of the carrier gas–liquid interface, ensuring that all the gas exiting the vaporization chamber is fully saturated. All properly functioning modern vaporizers have very predictable outputs within a clinically useful range of gas flow rates.2
Back pressure
Back pressure on the vaporizer can occur during intermittent positive pressure ventilation or with the use of the flush valve, and this effect can increase vaporizer output if compensation mechanisms were not in place. There are a number of ways that the effects of back pressure are minimized in modern vaporizer. One is to reduce the size of the vaporizing chamber relative to the bypass chamber. Another option is to use a long, spiral or large-diameter tube leading to the vaporizing chamber; both will reduce the amount of pressurized gas reaching the vaporizing chamber. The use of one-way check valves immediately upstream from the vaporizer can also prevent the effects of back pressure and are incorporated into some machines. One-way check valves are also sometimes used downstream of the flush valve (upstream of the vaporizer) to prevent back pressure.
There are many vaporizers available for use in veterinary anesthesia but most are based on three main styles of vaporizers: the Ohmeda Tec (GE Healthcare, Fairfield, CT), Drager Vapor (Dr ä ger Medical, Inc., Telford, PA), and Penlon Sigma (Penlon, Inc., Minnetonka, MN) series of vaporizers. The primary differences among vaporizer series include capacity of the vaporization chamber (larger chambers allow greater time between fillings, particularly useful for large animal applications), susceptibility to alterations in output due to tipping (rarely a consideration under normal circumstances), and mounting options.
Most vaporizers are mechanical devices requiring no external power to function normally. However, as a result of the unique vapor properties of desflurane, specially designed heated vaporizers are required to ensure consistent output. Additionally, there are electronic vaporizers available for use in both human and veterinary anesthesia. These vaporizers function as variable bypass vaporizers, but the splitting ratio of the carrier gas is determined electronically rather than mechanically. Since the system is electronic, various manufacturer and user alarm settings can be incorporated. For example, the vaporizer may alert the user if unusually high concentrations of anesthetic are being delivered from the vaporizer or that the vaporizer setting has not been altered within a specified time period, potentially avoiding inadvertent anesthetic overdoses (i.e., vaporizer output is momentarily increased and the user forgets to reduce the setting). Although electronic systems arguably provide additional information that may be valuable to the anesthetist, they may also be more prone to problems and damage related to the fact that they rely on properly operating electronics to function.
Vaporizers can be filled using a standard screw capped filler port or an agent-specific keyed filler port. Keyed filler ports are intended to prevent inadvertently filling a vaporizer with the wrong anesthetic agent. Most modern vaporizers are extremely dependable and durable, requiring very little routine maintenance and care. However, maintenance and care should be done according to the manufacturers’ recommendations and only performed by a certified technician.
Oxygen flush
Oxygen flush valves are found on most but not all veterinary anesthetic machines. Flush valves are designed to rapidly deliver large volumes of nonanesthetic-containing gas to the patient circuit in emergency situations. The flow originates downstream of the regulator within the intermediate pressure area of the anesthetic machine (∼50psi) and bypasses the flow meter and vaporizer, delivering gas at rates ranging between 35 and 75 L/min to the patient circuit. To avoid overpressuring the patient circuit, the flush valve should not be used, or should be used very cautiously in nonrebreathing circuits, circuits attached to mechanical ventilators, and circuits with very low volumes (i.e., pediatric circle systems), as pressures within the breathing circuit may temporarily rise, creating dangerously high pressures to the patients lungs. The adjustable pressure -limiting (APL) valves should be fully open at all times to help prevent overpressurizing the breathing circuit.
Common gas outlet
The common gas outlet leads from the anesthetic machine to the breathing circuit. Gas reaching the common gas outlet has traveled from the gas supply (cylinder or pipeline), through the regulator, flow meter, and vaporizer. The gas flowing from the common gas outlet normally delivers the anesthetic to the patient circuit at the concentration and flow rate determined by the vaporizer setting and flow meter flow rate. However, the concentration of inhalant gas from the common gas outlet is not usually equivalent to the gas concentration inhaled by the patient when using rebreathing circuits, particularly when using low FGF rates, due to dilution of incoming gases with those already in the patient circuit. When depressed, the flush valve also delivers gas to the common gas outlet, bypassing the flow meter and vaporizer.
Although some breathing systems are often built-in to anesthesia machines (i.e., the circle system), they are frequently considered separately from the actual anesthesia machine (i.e., gas regulating and inhalant deliver components). This is a particularly convenient way to discuss breathing systems in veterinary medicine, as on any single anesthetic machine, the breathing system may be frequently changed depending upon the needs of the patient or the circumstances in which anesthetic is delivered. The primary purposes of the breathing circuit are to: direct oxygen to the patient, deliver anesthetic gas to the patient, remove carbon dioxide from inhaled breaths (or prevent significant rebreathing of carbon dioxide), and to provide a means of controlling ventilation.
Breathing systems have been classified using numerous schemes. However, for clarity, it is suggested that the breathing circuit be classified into one of two groups: those designed for rebreathing of exhaled gases (rebreathing or partial rebreathing system) and those designed to be used under circumstances of minimal to no rebreathing (nonrebreathing systems). Some have argued that this classification is a bit of a misnomer, since depending upon the specific system used and the FGF rates used, a rebreathing system may have minimal rebreathing occurring (i.e., excessively high FGFs) or a nonrebreathing system may not completely prevent rebreathing (i.e., inadequate/low FGF). To help circumvent this debate it has been suggested that in addition to describing the design of the breathing circuit, the FGF rate should be provided to fully describe how the system is being used.8 The FGF rate should be expressed in milliliters per kilogram per minute in veterinary medicine, owing to the vast range of patient sizes encountered. Additionally, the degree of rebreathing with breathing circuits can be affected by other factors such as the equipment dead space and the patient’ s respiratory pattern. Suffice it to say that breathing systems have been designed to function as rebreathing or nonrebreathing systems and should be used in the manner they were originally intended.
Rebreathing (circle system)
The circle system is the most commonly used rebreathing system and will be the only one described here, although other types of rebreathing systems have been used (i.e., to-and-fro). The circle system is designed to produce a unidirectional flow of gas through the system and has a means of absorbing carbon dioxide. The components of the circle system include: fresh gas inlet, inspiratory one-way valve, breathing tubes, expiratory one-way valve, APL valve, reservoir bag, and carbon dioxide absorber.
The FGFs used with a circle system determine the amount of rebreathing; full rebreathing (closed), partial rebreathing (semiclosed, low flow), and minimal rebreathing. Historically, many terms have been applied to describe the amount of rebreathing, but there is no universally accepted standard or description of these terms. However, it has been suggested by several authors that the use of the terms open, semiopen, and semiclosed be dropped to avoid confusion.9
Full (complete) rebreathing
This describes a circle system using flow rates equal to the metabolic consumption of the patient between 3 and 14 mL/kg/min.10 This is sometimes also described as a closed system; however, it may be best to avoid using such terms to avoid confusion.
Partial rebreathing
This describes a circle system using a flow rate greater than metabolic oxygen consumption (∼ 20 mL/kg/min) but less than that required to prevent rebreathing (∼200–300mL/ kg/min). Since this is a very large range it is often divided arbitrarily into low flow (20–50mL/kg/min), mid-flow (50–100mL/kg/min), and high flow (100–200mL/kg/min) although this is not a universally accepted description.
Non (minimal)-rebreathing circle system
This would describe a circle system using flow rates greater than 200 mL/kg/min. Flow rates that would not normally be used in most circumstances. However, this flow rate may result when circle systems are used for maintenance of anesthesia in very small patients (<5kg) with flow rates of 1000 mL/min or greater. Frequently in veterinary medicine it is suggested that flow rates below 1000 mL/min should not be used and although this recommendation may be clinically useful for preventing anesthetic-related errors by increasing the margin of anesthetic safety, most modern anesthetic systems (i.e., vaporizers) continue to function optimally down to flow rates of 500 mL/min. With the availability of low volume, low dead-space pediatric and neonatal circle systems, it is becoming increasingly common to use circle systems with partial rebreathing flow rates (i.e., <1 000 m L/min) in small patients (<5kg).
Obviously it is most economical in terms of both oxygen and gas anesthetic use to employ low flow rates when possible. Lower flow rates are also associated with less environmental contamination by halogenated hydrocarbons (all commonly available inhaled anesthetics) and the better maintenance of body temperature. However, lower flow rates are also associated with lower volumes of anesthetic delivery to the circuit, leading to slower changes of the inspired gas concentrations by the patient. Low FGF rates can be offset by using higher vaporizer settings.
Most anesthetics are delivered initially by relatively high gas flow rates to ensure rapid attainment of sufficient anesthetic concentration within the circle to maintain anesthesia in the patient following intravenous anesthetic induction. The flow rates are normally decreased after the first 10–20 minutes to economize on gas use and waste. The use of high FGFs enables the anesthetist to deliver large volumes of anesthetic vapor during the initial period of uptake by the patient and allows rapid changes in anesthetic depth. This ensures the patient’ s inspired gas concentration is more reflective of the concentration of anesthetic gas delivered from the vaporizer. This is in contrast to using low FGF, where the inspired patient anesthetic concentration will not necessarily reflect the vaporizer concentration of gas until nearing equilibration (i.e., anesthetic uptake, distribution, and metabolism are nearly equal to anesthetic delivery). The interaction between the vaporizer output, circuit volume, patient size, and flow rate is often an unfamiliar and difficult concept to grasp. Equating anesthetic delivery to a constant rate infusion of an intravenous drug is perhaps a more familiar comparison for understanding inhalant anesthetic delivery.
The actual configuration and features of each circle system vary somewhat depending upon the manufacturer, but in general a common pattern of gas flow is followed through the fresh gas inlet, the inspiratory one-way valve, the inspiratory and expiratory breathing tubes, the expiratory one-way valve, the APL valve, the reservoir bag, and the carbon dioxide absorbing canister back to the fresh gas inlet.
Fresh gas inlet
The fresh gas inlet is the site of gas delivery to the circle system from the common gas outlet of the anesthetic machine. The fresh gas inlet is normally found after the carbon dioxide absorber and before the inspiratory one-way valve.
Inspiratory one-way valve
During inspiration, the inspiratory one-way valve opens, allowing gas to move from the fresh gas inlet and reservoir bag to move through the valve into the inspiratory limb of the breathing circuit. During expiration, the inspiratory valve is forced closed, preventing exhaled gas from entering the inspiratory limb of the breathing circuit, forcing it into the expiratory limb of the breathing circuit.
Breathing circuit/tubing
The most basic breathing circuit is made up of a corrugated plastic or rubber inspiratory and expiratory limbs. The corrugated tubing helps prevent kinking and allows for some expansion if the breathing circuit is subjected to any compression or traction. The two breathing limbs are connected via a Y-piece and the Y-piece connects to endotracheal tubes and facemasks. There are also various coaxial designs that place the inspiratory limb within the expiratory limb of the breathing circuit. Coaxial systems reduce the bulk associated with the breathing system and at least theoretically the design facilitates warming the cold inspired gases by the warm expired gases. The Universal F-circuit (a coaxial breathing system) is designed to function with standard circle systems (i.e., 22 mm OD connectors of the circle system). Most breathing circuits can be adapted for use with all circle systems as the fitting diameters are standardized. However, there is a proprietary coaxial circuit available that utilizes nonstandard-sized circle system connectors requiring the use of a proprietary circle system. There are also several sizes of breathing circuits available that vary in length, volume, and the amount of dead space to meet various anesthetic requirements. Pediatric and neonatal rebreathing circuits are normally low volume and low dead space systems, allowing them to function optimally in small patients (i.e., those with small tidal volumes).
Expiratory one-way valve
The expiratory one-way valve functions together with the inspiratory one-way valve, closing upon inspiration and opening during expiration. This valve helps direct gas into the expiratory limb of the breathing system, through the expiratory valve, and into the reservoir bag. At least one system also incorporates a negative pressure relief valve, providing an alternative path of gas flow (room air) to the patient should the inspiratory valve become stuck in the closed position.
Reservoir bag
The reservoir bag is also referred to as a breathing or rebreathing bag. The purpose of the reservoir bag is to provide a compliant reservoir of gas that will expand and collapse with the patient’ s expiration and inspiration. It is commonly recommended that the reservoir bag be a volume equal to or greater than six times the patient’ s normal tidal volume (10–20 mL/kg). Ultimately the reservoir bag should be large enough to provide a reasonable-sized reservoir of gas, but not so large that it becomes difficult to quantify the size of the breath by observing movements of the bag. In addition, a very large reservoir bag will contribute to the overall functional volume of the rebreathing system (i.e., circle system), contributing to slower rates of change in anesthetic concentration within the breathing system when the vaporizer output is altered. This would not be the case when using a nonrebreathing system.
APL valve
The APL valve is also commonly referred to as the overflow, pop-off, or pressure relief valve. The APL is a safety valve allowing excess gas to escape from the patient circuit. If the valve is functioning properly gas should escape if pressures exceed 1–3 cm H2O and it should be left fully open at all times. It may be closed slightly if the rebreathing bag collapses completely but gas should still escape if pressures exceed 1–3 cm H2O. The APL valve may be closed temporarily to deliver positive pressure ventilation but should be immediately reopened to prevent excessive pressure from building in the patient circuit.
Figure 5.8. (A) An example of a momentary closure valve built into the adjustable pressure-limiting (APL) valve and (B) a momentary closure valve that can be added to any standard APL valve demonstrating its use to deliver a breath.

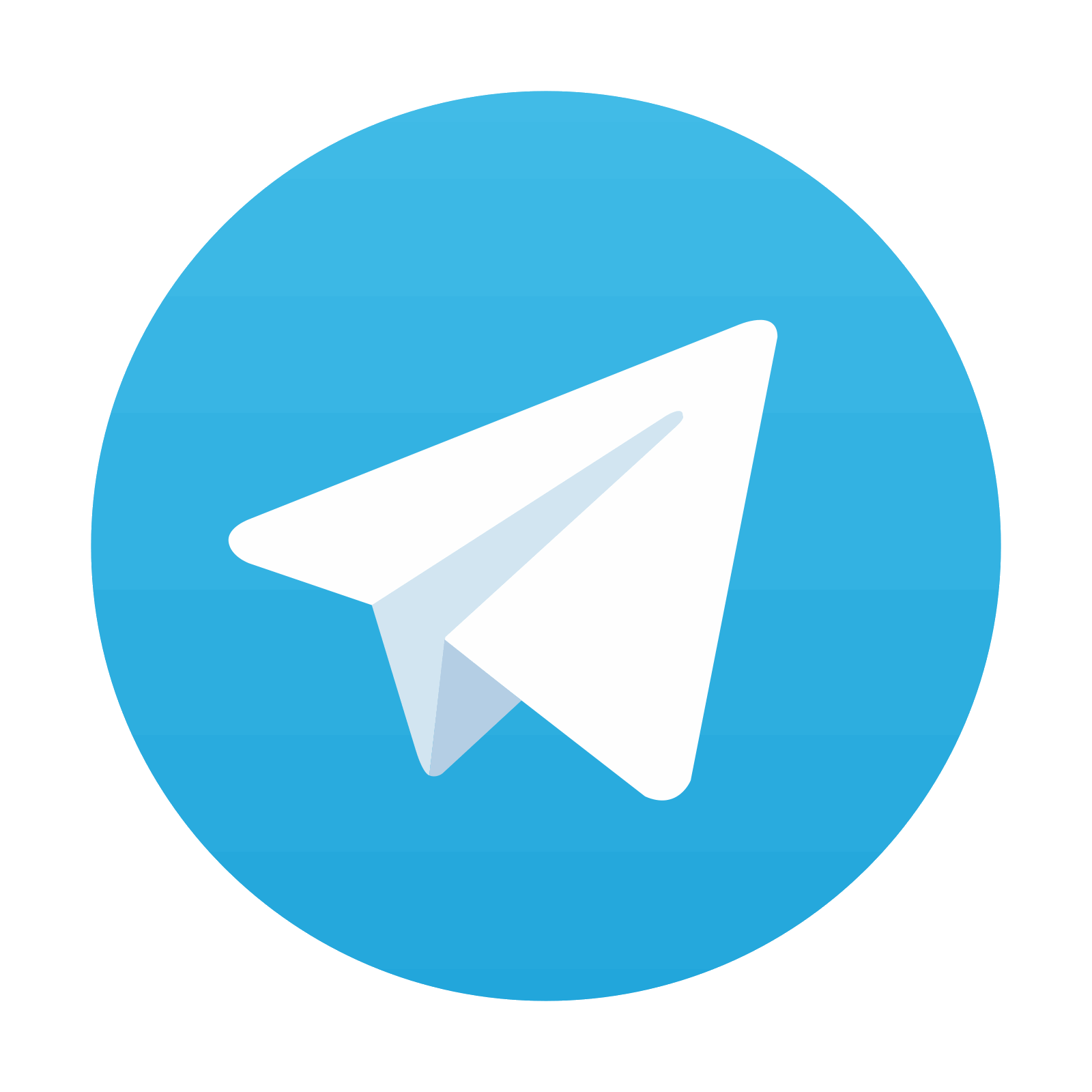
Stay updated, free articles. Join our Telegram channel
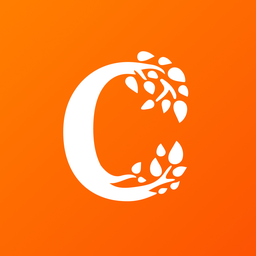
Full access? Get Clinical Tree
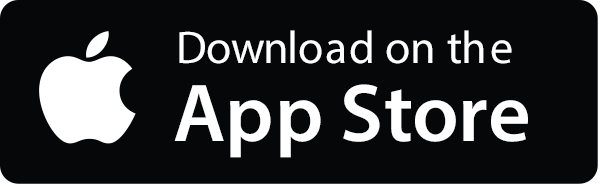
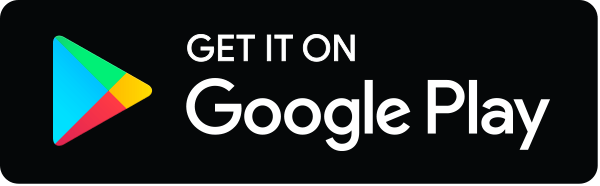